TECHNICAL ASPECTS OF RENAL REPLACEMENT THERAPY IN THE INTENSIVE CARE UNIT
This section of the chapter discusses different modalities of RRT in the ICU and review through the calculations for clearance. Also, it reviews forms of anticoagulation for CRRT, dialysis solution, dialysis access, and other technical aspects.
Modes of Renal Replacement Therapy
There are multiple options for RRT that can be used in the treatment of AKI in the ICU. These include conventional IHD; various modalities of CRRT; “hybrid” modalities that combine features of IHD and CRRT, which are known as prolonged intermittent renal replacement therapy (PIRRT); and peritoneal dialysis (PD). TABLE 40.1 summarizes solute clearance and prescription for each therapy.
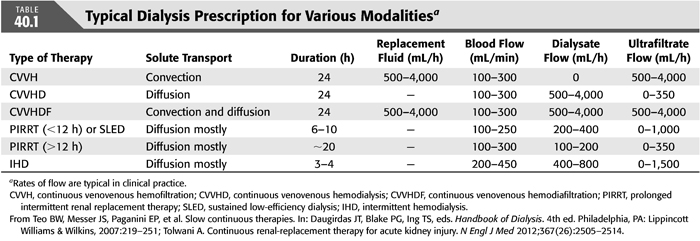
Intermittent Hemodialysis
Traditionally, nephrologists have used IHD to treat AKI in the ICU. Solute clearance is mainly through diffusion, and volume is removed via ultrafiltration. The principal advantages of IHD include rapid removal of solute and volume, making it ideal for certain clinical scenarios such as drug intoxication and management of severe hyperkalemia. The major disadvantage of IHD is the risk of hypotension, which occurs in approximately 20% to 30% of hemodialysis sessions (11,12). The rapid solute removal and shift in electrolytes can exacerbate hemodynamic instability, causing IHD to be intolerable in critically ill patients. For adequacy, the target Kt/V is 1.2 per treatment or urea reduction ratio greater than 0.7. IHD and PD are discussed in greater detail in other sections of this book.
Prolonged Intermittent Renal Replacement Therapy
This category is also known as extended daily dialysis (EDD). The common form of PIRRT is sustained low-efficiency dialysis (SLED), which uses the same dialysis machine as for IHD, but the therapy is delivered at reduced blood and dialysate flow rate over a longer duration of time, usually over 6 to 10 hours. Typical blood-flow rate is around 200 to 300 mL/min with dialysate flow rate around 200 to 400 mL/min. Also, PIRRT can be delivered longer than SLED with duration around 20 hours. In this situation, blood flow is around 150 mL/min with dialysate flow around 100 mL/min. The major benefits of SLED are the reduced cost and staff time while providing solute removal equivalent to CRRT. Also, SLED may be routinely performed without anticoagulation. In one study, the weekly cost to the hospital was $1,431 for SLED; $2,607 for CRRT with heparin; and $3,089 for CRRT with citrate (13).
Continuous Renal Replacement Therapy
CRRT was developed in the 1980s specifically for the treatment of critically ill patients with AKI who could not tolerate conventional IHD due to hemodynamic instability or when IHD could not control volume and metabolic derangements. The slower clearance and volume removal through CRRT make it the preferred modality in the ICU patient requiring vasopressors. CRRT allows for greater volume removal with better hemodynamic tolerance in patients who have high obligate fluid intake such as more than 4 L/d. Disadvantages for CRRT include the higher cost, increased nursing support, frequent clotting, and risk of underdosing of antibiotics and other medications (TABLE 40.2). Medication dosing in CRRT is not well studied.
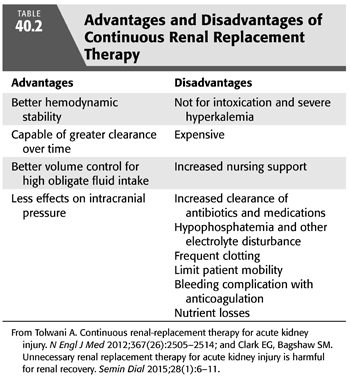
The blood circuit for CRRT is commonly a venovenous circuit through a double-lumen, large-bore catheter. There are four options for CRRT: continuous venovenous hemodialysis (CVVHD), continuous venovenous hemofiltration (CVVH), continuous venovenous hemodiafiltration (CVVHDF), and slow continuous ultrafiltration (SCUF) (14,15). FIGURE 40.1 shows the circuit components in the various forms of CRRT. TABLE 40.1 summarizes typical dialysis prescription for different forms of CRRT. The usual blood flow is around 200 to 300 mL/min. Increasing blood flow from 100 or 150 mL/min to 200 or 300 mL/min helps increase the efficiency of clearance and reduce the risk of clotting (16).
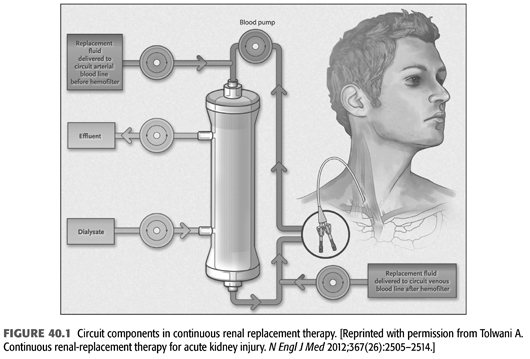
Continuous Venovenous Hemodialysis
The primary means of solute clearance is through diffusion. The dialysate flow is slower in comparison to IHD and runs in the opposite direction to the faster moving blood flow in a countercurrent course, allowing for maximal saturation of solutes of the outflow dialysis. Solute removal occurs by concentration gradient between blood and dialysate compartment across a semipermeable biosynthetic membrane otherwise known as the hemodiafilter. Substances such as urea, creatinine, and potassium diffuse down their concentration gradient from the blood to dialysate compartment during hemodialysis, while other solutes, such as bicarbonate, move from the dialysate to blood compartment. The rate of removal of solute through diffusion depends on the size. Smaller molecules diffuse more easily, and larger molecules diffuse slowly and are not as easily removed (14,15).
Continuous Venovenous Hemofiltration
The primary means of solute clearance is through convection. As the blood flows through the hemodiafilter, transmembrane pressure gradient between the blood and ultrafiltrate compartment forces plasma water to be filtered across the hemodiafilter (or also known as hemofilter). The small-sized solutes (such as urea and electrolytes) are removed in the same concentration as the plasma. The volume removed in the ultrafiltrate is replenished by a replacement (or substitution) fluid to prevent excessive fluid removal and volume depletion, thereby diluting the concentration of the plasma solutes. The replacement fluid is infused into the blood compartment either prefilter (also known as predilution), postfilter (also known as postdilution), or both. The rate of solute removal depends on the size of hemofilter pores and the convective force, which allows for larger molecules to be removed in contrast to hemodialysis (14,15).
The downside of using only postfilter replacement is the increased rate of clotting as the blood filters through the hemofilter and becomes increasingly concentrated at the end of the hemofilter with the removal of the ultrafiltrate. Therefore, replacement fluid is usually infused either as all prefilter or as a combination of prefilter and postfilter to reduce the risk of clotting. Common percentages for replacement fluid is 100% prefilter, 70% prefilter with 30% postfilter, and 50% prefilter with 50% postfilter. As the percentage of replacement fluid is increased postfilter, the risk of clotting rises, which can be ascertained by calculating the filtration fraction. Filtration fraction above 25% indicates a greater risk for clotting, and these calculations are described further in the next section of this chapter. On the other hand, when replacement fluid is given prefilter, the blood entering the filter will become diluted, reducing the maximum solute clearance. For instance, if prefilter replacement fluid is infusing at 40 mL/min and blood flow is at rate of 200 mL/min, the dilution factor will be 200/240 = 83% or there is a 17% dilution. Depending on the solute and dialysis prescription, prefilter replacement reduces clearance by 15% to 20% (17). On a milliliter for milliliter basis, CVVHD is more efficient, removing small-sized solutes in comparison to CVVH with prefilter replacement. However, middle-sized molecule solutes (500 Da to 60 kDa) such as β2-microglobulin are cleared more effectively with CVVH due convective clearance.
Continuous Venovenous Hemodiafiltration
This combines both CVVHD and CVVH, which is usually with prefilter replacement therapy. There are several types of CRRT machines available, and depending on the machine, it can provide different modalities.
Slow Continuous Ultrafiltration
This is used commonly in patients who need only volume removal but not solute clearance. There is no dialysis or replacement fluid in this mode of therapy; only ultrafiltrate is removed at a slow rate using a hemofilter.
Dialysate Solution
Dialysate and replacement solutions are very similar, and many of the dialysate solutions are being used as replacement fluid off-label. These solutions contain potassium, calcium, magnesium, and glucose in physiologic ranges. There are additional solutions such as varying potassium and calcium baths, which are available for special circumstances. For hyperkalemia, one can use 0 to 2 mEq/L potassium bath. As for the anion buffer, acetate, lactate, citrate, and bicarbonate have all been used, but bicarbonate is preferred (14). Lactate-based solutions are unfavorable because it can cause hyperlactatemia in critically ill patients with liver dysfunction and may be associated with higher episodes of hypotension in comparison to bicarbonate-based (15,18). Acetate-based dialysate fluid has been implicated with contributing to intradialytic hypotension with IHD. Acetate can induce production of nitric oxide, a vasodilator (19). However, recent study showed that acetate-containing solution does not seem to affect hemodynamics because lower amount of acetate is delivered in comparison to the amount delivered in IHD (15,20). Popular dialysate solutions have predominant concentration bicarbonate with low concentration of lactate, usually around 3 mmol/L. Lower levels of bicarbonate are recommended with citrate anticoagulation to avoid severe metabolic alkalosis since citrate is metabolized by liver to bicarbonate.
Vascular Access
Vascular access is absolutely necessary for RRT. However, it must be kept in mind that with ICU patients with AKI, there is the possibility of long-term dialysis, so maintaining the vasculature is important for future dialysis access. There are primarily three forms of access: dialysis catheters (DCs), arteriovenous fistula (AVF), and arteriovenous graft (AVG). In the various modes of CRRT, the DC is preferred. There is limited data on DCs used in the ICU as most of the data is actually from studies looking at central venous catheters (CVCs) and access in end-stage kidney disease (ESKD) patients. It is questionable if this data is applicable as there are significant differences between the catheters and a patient in the ICU versus a patient in the dialysis unit.
Blood flow through the catheter is a significant factor in determining the dialysis dose provided. This is particularly important in IHD since blood flow tends to be higher in comparison to CRRT, in which dosing is more dependent on the effluent volume. DC dysfunction as defined in various studies as a low blood flow of <150 to 200 mL/min with inadequate dialysis dose delivery and blood flow reduction of more than 20% even after attempts to maintain patency of the DC (21). Early DC dysfunction is usually due to improper catheter placement technique such as cannulating the wrong vessel, catheter tip malposition, or kinking of the catheter. Late DC dysfunction is usually due to thrombosis intrinsic (obstructive thrombosis of the catheter lumen) or extrinsic (thrombosis or stenosis of the blood vessel) to the catheter. The majority of DC catheter dysfunction occurs in the first 10 days but increases the longer the catheter remains inside (21,22). Countermeasures that can be used to increase the blood flow after catheter placement include repositioning the patient, flushing the line with saline, using an anticoagulation (citrate or heparin) dwell in the DC, and reversing the lines. Of course, accurate placement of the DC is important, and this has improved with the use of ultrasound guidance to image the targeted vessel throughout the procedure and x-ray to confirm placement. If all measures fails, then replacing the DC might be the only alternative to provide an adequate dialysis dose. This can be done over a guidewire.
Another complication of DC is infection, which is defined by two separate cultures separated by 120 minutes that grow the same phenotypic organism. One culture is drawn from the peripheral blood and the other from the CVC. The rates of catheter infections have dramatically declined due to various methods aimed at preventing CVC infections in the ICU. These methods include adequate hand hygiene, barrier precautions, prompt removal of catheter when it is no longer necessary, and advancements in dressings. There is a need for large randomized trials before the routine use of antimicrobial locks or antimicrobial catheters can be recommended (22,23).
The options of dialysis access site are the internal jugular (IJ) vein, the subclavian vein, and the femoral vein. The most recent Kidney Disease: Improving Global Outcomes (KDIGO) AKI guidelines present an ungraded recommendation for which vessels to use. In descending order from most to least desired site, right IJ vein, femoral vein, left IJ vein, and lastly, the subclavian veins. The reason for the left IJ vein and both subclavian veins are less desirable are due to the increased risk of the development of central vein stenosis (24). Traditionally, femoral vein access has been attributed to greater infection risk and to restrict patient mobility (23). This has more recently come under scrutiny. Parienti et al. (25) performed one of the first randomized controlled trials on catheter site insertion. They found that the risk for a catheter-related blood stream infection was lower for femoral lines if the body mass index (BMI) was <24.2 and for the IJ if BMI was >28. A meta-analysis reported that outside of patients with a high BMI that there was no significant increase in infection rates between the subclavian or IJ veins versus the femoral vein. The meta-analysis also stated that there was no difference in the deep vein thrombosis rates (26). Both of these conclusions of no significant difference in infectious or thrombosis rates were reported by a recent Cochrane review (27).
The catheter site does also play a role in catheter dysfunction rates and the dose of dialysis delivered to a patient. In the multicenter Cathedia Study, which included 736 patients, there were similar rates of first DC dysfunction between the IJ (11.1%) and femoral (10.3%) veins. It is important to note the side of the DC affected dysfunction rates: the right IJ was 6.6%, the femoral veins 10.3 %, and the left IJ was 19.5%. In regard to dialysis dose, if the desired blood flow is less than 200 mL/min, there is negligible difference between the IJ and femoral veins; however, if the blood flow is over 200 mL/min, the right IJ vein is preferred (28). Remember, the right IJ vein is the most direct and straight route to the superior vena cava (22).
In summary, the first choice of access should be the right IJ vein; remember, an advantage of IJ access is that the patient will be able to mobilize easier (24). The femoral veins should be considered particularly in patients with a lower BMI (<24), high likelihood of long-term dialysis, and if emergent dialysis is needed with inexperienced operator or no ultrasound available. The left IJ vein should be considered if the right IJ and femoral veins are not optimal choices. The subclavian vein is the last resort with the right side being preferable (23).
Anticoagulation
The CRRT extracorporeal circuit’s patency and filter lifespan are of critical importance to ensure that there is adequate solute clearance and filtration. A major hindrance to this is clotting in the extracorporeal circuit leading to a discontinuation of therapy (29). It has been shown that when 24 hours of CRRT are prescribed on average without anticoagulation, the patient receives therapy for about 16 to 18 hours. This interrupted treatment results in a higher cost, unnecessary blood loss, and an increased strain on nursing. Hence, prevention of clotting by various methods including anticoagulation is required for the patient on CRRT. In regard to anticoagulation, it is usually necessary for the patient on CRRT, and this is a distinct disadvantage of this modality of RRT. Studies have shown 5% to 50% of patients on anticoagulation with CRRT have bleeding complications. The desired anticoagulant should have certain qualities including providing optimal anticoagulation with minimal systemic side effects including bleeding. It should be readily available, able to be monitored, reversible, and inexpensive (30–32). In this section, we will discuss both nonanticoagulant and anticoagulant strategies to prevent clotting of the CRRT extracorporeal circuit.
The patient’s clinical status, characteristics of the extracorporeal circuit, and blood flow all affect the coagulation pathophysiology of the patient. The clinical status can have a procoaguable effect from the pathologic presence of proteins as in antiphospholipid syndrome or secondary to overt inflammation as in sepsis. It is thought that once blood comes into contact with the extracorporeal circuit, there is stimulation of the coagulation system. This includes activation of the intrinsic and extrinsic coagulation systems with thrombin generation, monocytes, and platelets. In an inflamed state, there is diminished activity of the endogenous anticoagulation system with decreased antithrombin, protein C, and tissue factor plasminogen inhibitor. This is due to decreased production and even direct lysis of anticoagulants. Once the clotting starts, usually there is fibrinolysis, but this is impaired as well with the increased activity of plasminogen activator inhibitor. The endothelium’s antithrombogenic properties are also lost due to inflammation and oxidative stress. Other causes of clotting are blood flow stasis, hemoconcentration, turbulent blood flow, and contact between blood and air (30,32).
Vascular access obviously affects blood flow. We have gone into further detail elsewhere about vascular access but will briefly review the type and location of access. There is no significant difference in filter life when comparing the IJ and femoral sites (33,34). However, there is less infectious risk and recirculation with an internal IJ. Femoral catheters tend to kink more particularly in the obese patient. IJ catheters can generate high access pressures when a patient is agitated or coughing. There is some data that show the right side for both catheters are associated with less catheter dysfunction (34). The actual catheter itself should optimally be short and have a wide inner diameter. In addition, staff managing the CRRT extracorporeal circuit should respond to pump alarms in a timely manner, be able to recognize when a catheter is kinked, and properly rinse the filter to minimize the blood-air contact which initiates clotting (32). We will discuss filtration fraction later in this chapter.
We will primarily discuss the use of heparin and citrate for anticoagulation. There are other potential agents available that are not routinely used. These include heparinoids and platelet-inhibiting agents. We will not cover these agents in this section.
Unfractionated Heparin Anticoagulation
Unfractionated heparin (UFH) is the most commonly used anticoagulant. UFH’s mechanism potentiates the effects of antithrombin III by a thousandfold, inhibiting thrombin activity and factor Xa. The half-life of UFH is about 90 minutes to 3 hours depending on kidney function. UFH has advantages including physician familiarity, reversibility with protamine, relative short half-life, and ease of monitoring. There are significant disadvantages such as dosing variability, heparin-induced thrombocytopenia (HIT), heparin resistance, and risk of hemorrhage. The incidence of bleeding has a wide range of 10% to 50%. UFH when used is usually as a bolus of 2,000 to 5,000 IU at 30 IU/kg. This is followed by a continuous infusion in the arterial arm of 5 to 10 IU/kg/h to maintain an activated partial thromboplastin time (aPTT) of 34 to 45 seconds or an aPTT of 1.5 to 2.0 times the normal level (31,35).
The reversal agent or antagonist for UFH is protamine. When used in CRRT, protamine is given postfilter at a ratio of 100 between prefilter heparin (IU) and protamine (mg). The protamine–heparin complex is released into the body and broken down by the reticuloendothelial system. The side effects of protamine include hypotension, thrombocytopenia, leukopenia, cardiac depression, and anaphylaxis. Regional anticoagulation using heparin with protamine reversal is cumbersome due to the difficulty in calculating how much protamine is actually necessary. There is also variability in prolonging filter life. Therefore, heparin anticoagulation is commonly applied without the use of protamine. Low molecular weight heparin (LMWH) is not used routinely for CRRT anticoagulation. This is due to an increased risk of bleeding, cost with need to monitor anti-Xa activity, and risk of HIT, and lack of clearance in kidney disease (31). However, even if one is able to perform anti-Xa monitoring, it is not entirely reliable in predicting bleeding complications (10).
Heparin-Induced Thrombocytopenia
Once there is a suspicion for HIT, all heparinoids should be stopped as soon as possible and replaced with alternative anticoagulation. Thrombocytopenia occurs in up to 60% critically ill patients, and when CRRT is initiated, a drop in platelet count by as much as 50% has been reported. The diagnosis of HIT is based on detection of platelet factor 4 antibodies (10,36,37). Another functional assay used as a diagnostic test is the serotonin releases assay. The alternative anticoagulation used in the clinical setting of HIT with CRRT is a direct thrombin inhibitor. The preferred direct thrombin inhibitor of choice in renal dysfunction without liver failure is argatroban and in those with liver failure, bivalirudin. These agents would treat both the procoagulable state of HIT and provide anticoagulation for the CRRT. An alternative anticoagulation strategy to prevent HIT is to avoid heparin-based agents all together and use citrate anticoagulation (37–39).
Citrate Anticoagulation
Citrate has multiple advantages, namely, avoidance of systemic anticoagulation and no association with HIT. It has been shown to have better filter survival times and less bleeding when compared to heparinoids (40–42). Disadvantages of citrate anticoagulation are metabolic abnormalities, increased complexity, and the lack of universal availability. Citrate solutions and protocols have been reviewed recently. Citrate prevents clotting in CRRT by chelating ionized calcium in the extracorporeal circuit. Calcium is required for multiple steps in the coagulation cascade. There is also thought to be additional antihemostatic and anti-inflammatory properties of citrate. Citrate is infused in the arterial limb (prefilter) of the CRRT proportional to the blood flow. Citrate itself is not measured, but instead, the postfilter ionized calcium is used. The citrate infusion is titrated so that the postfilter ionized calcium is <0.35 mmol/L. This level of ionized calcium is associated with optimal anticoagulation in the extracorporeal circuit and correlates with a citrate concentration of 4 to 6 mmol/L (40,42,43). Citrate has a low molecular weight, high sieving coefficient, and high diffusion/convective clearance so that a major portion of the citrate–calcium complexes is lost in the effluent (44). This would lead to systemic hypocalcemia if not for the calcium infusion postfilter to replace the lost calcium. Calcium chloride or calcium gluconate is used for the calcium infusion. Citrate–calcium complexes that are not lost in the effluent but instead return to the systemic circulation are metabolized to bicarbonate by the skeletal muscle, kidney, and liver. Each citrate–calcium complex yield three molecules of bicarbonate and returns the ionized calcium to the patient (40,42).
Metabolic Abnormalities with Citrate Anticoagulation
The most common metabolic complications of regional citrate anticoagulation are hypocalcemia and metabolic alkalosis (45). We will discuss monitoring of electrolytes in the next section. Intravenous infusion of calcium postfilter is given and adjusted on an ongoing basis to prevent hypocalcemia. In general, with blood-flow rates of about 200 mL/min and effluent rates of 25 to 30 mL/kg/h, calcium supplementation starts at 2 to 3 mmol/h. One has to be cognizant of the replacement fluid/dialysate containing calcium as this could affect the rate of citrate and calcium infusions. The goal for the systemic ionized calcium is 0.9 to 1.2 mmol/L. The other possible etiology of hypocalcemia with citrate infusion is ineffective metabolism and subsequent citrate accumulation seen in liver failure or tissue hypoperfusion (shock). If citrate is not metabolized, then calcium is not released from the citrate–calcium complex. The solutions for this include reducing the citrate infusion, enhancing citrate removal in the effluent (by increasing the replacement fluid or dialysate rate), and switching from citrate- to bicarbonate-based replacement fluid or dialysate. Hypercalcemia also occurs with citrate anticoagulation if there is an excessive replacement with intravenous calcium (40,42).
Another effect of inadequate citrate metabolism is anion gap metabolic acidosis. The management of this is similar as mentioned previously for hypocalcemia in the same clinical setting. Metabolic acidosis is also caused by an imbalance of buffer (citrate and bicarbonate) being delivered to the patient and that being lost in the effluent. One can increase the amount of buffer by increasing the citrate infusion rate or by decreasing the loss in the effluent by reducing the replacement fluid or dialysate rates. Other options are intravenous bicarbonate infusion or increasing the amount of bicarbonate in the replacement fluid or dialysate.
Metabolic alkalosis as we have mentioned is the second most common complication. This is usually secondary to an excess buffer load or not appropriately matching the CRRT solutions with citrate. An excess buffer load is a result of a higher blood-flow rate, which subsequently infuse citrate at a higher rate. One option is to reduce the amount of citrate entering the circuit by decreasing blood flow or by aiming for a higher ionized calcium goal (i.e., lower citrate level). The other option is to increase the citrate lost in the effluent by increasing the replacement or dialysate flow rates. An important caveat is that there are other sources of citrate in the intensive care setting. Citrate is the preservative for blood products, and so the patient receiving multiple transfusions is also receiving an increased amount of citrate. Another example is acetone in total parenteral nutrition. The other mechanism of metabolic alkalosis is inappropriately high bicarbonate in the replacement fluid or dialysate in a patient receiving citrate. The conversion of the citrate that reaches systemic circulation to bicarbonate must be accounted for when considering the replacement fluid or dialysate. For instance, when starting a patient on citrate who was not previously on anticoagulation with CRRT, one must lower the bicarbonate in the CRRT fluids being used. Another approach to prevent metabolic alkalosis is to use hyperchloremic solutions such as normal saline pre- or postfilter (40,42,45).
Other electrolytes potentially affected include magnesium and sodium. Magnesium is also chelated by citrate but usually does not need replacement as magnesium is found in the dialysate or the replacement fluids. Hypernatremia can result from not adjusting to the sodium load that occurs with citrate anticoagulation. To prevent hypernatremia, it is important to reduce the sodium concentration in the dialysate or the replacement fluids. Hyponatremia is seen if there is an excess of hypotonic fluid being given to the patient or a technical error where the citrate has not been given (45).
Citrate Toxicity and Monitoring
Citrate accumulation and toxicity risks include the patient with liver dysfunction, with severe tissue hypoperfusion (shock), or a large citrate load (multiple blood products). The liver is the main site of metabolism for citrate. Clinically, this is seen with worsening metabolic acidosis and hypocalcemia potentially leading to hypotension and arrhythmias. Typically, electrolytes are monitored frequently when a patient is on CRRT by as much as every 4 to 6 hours. More frequent monitoring could be required for the patient with an increased risk for citrate toxicity (40,42). Citrate itself is not routinely measured but instead the ratio of total-to-ionized calcium (calcium ratio) is used as a surrogate for the citrate level. The accumulation of citrate results in an increased infusion of calcium postfilter leading to a disproportionate rise in total calcium as compared to ionized calcium. The critical cutoff for the calcium ratio is >2.5 indicating that the patient is at increased risk for metabolic complications and citrate toxicity (46). Management of citrate accumulation and toxicity revolves around reducing exposure and increasing the clearance of citrate. This can be done by reducing the blood-flow rate and amount of citrate infused aiming again for higher calcium targets. On the other hand, the clinician can increase clearance by increasing convective or dialysis forces (43).
In high-risk patients, such as those with liver failure, citrate may be used but with caution. Liver indices are not always reliable in predicting metabolic clearance. Better predictors of the liver’s ability to metabolize citrate include a prothrombin time ≥33 seconds and a lactic acid level ≥3.4 mmol/L (47,48). Laboratory monitoring should be more frequent as much as every 2 hours and should include the calcium ratio and the pH. We have mentioned citrate toxicity management by decreasing the exposure and increasing the clearance of citrate in this section already. Other available measures to manage metabolic acidosis and hypocalcemia are the systemic infusion of bicarbonate and/or calcium as needed. If acidosis is present, we advise to correct the calcium first as bicarbonate infusion can result in a further drop in ionized calcium level (43).
In summary in deciding which modality of anticoagulation to use for CRRT, it is best to consider the time interval it will be required for, the bleeding risk of the individual patient, and the therapeutic agents available. The clinician must also be aware of the intensity of monitoring and the use of resources required with anticoagulation in CRRT. In the patient that cannot have anticoagulation, it is possible to maintain CRRT with other strategies as we have described (49).
Clearance for Continuous Renal Replacement Therapy
The “dose” of RRT administered is determined by the effluent flow, which comprises of ultrafiltrate in CVVH, the spent dialysate in CVVHD, and both in CVVHDF. It is commonly reported as the effluent flow rate in milliliters per kilogram per body weight. The recommended effluent flow dose is at least 20 to 25 mL/kg of body weight per hour in order to achieve adequate solute clearance (14,50,51). There are often frequent interruptions in CRRT such as procedures and clotting of hemofilter; therefore, effluent flow may need to be increased to account for lost time. In addition, clotting and protein deposition on hemofilter can reduce actual solute clearance (14).
Calculations
Where K is the clearance, QE is the effluent rate, CE is the effluent concentration, and CB is the blood concentration. K is expressed in mL/min (EQUATION 40.1) (52).

where Qd is dialysate fluid rate, QR is replacement fluid rate, Qnet is the net fluid removal rate, and Quf is the total ultrafiltration volume. All are reported in mL/min. The effluent rate, which is also known as “dose” of CRRT, can be expressed in mL/h or as mL/kg/h. The recommended “dose” of RRT is at least 20 to 25 mL/kg/h (EQUATIONS 40.2 and 40.3) (50–53).


where σ is the sieving coefficient. For small molecules, the sieving coefficient approximates to 1. Using urea as the solute, equilibrium is achieved between CE and CB since the effluent rate is much smaller than the blood-flow rate and the solute size for urea is small (60 Da) (EQUATION 40.4). For instance, effluent rate is usually ranging 17 to 50 mL/min versus blood-flow rate around 200 to 300 mL/min. Therefore, the sieving coefficient for urea is 1. Another determinant of the sieving coefficient is the pore size of the hemodiafilter. TABLE 40.3 shows an example of the sieving coefficient of different molecules (54).

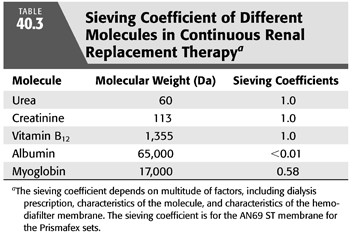
Diluting factor is used in calculating K for CVVH with prefilter replacement, which reduces the maximum solute clearance per unit volume of replacement fluid in comparison to postfilter replacement (EQUATION 40.5). With the infusion of prefilter replacement fluid, the blood entering the hemofilter becomes diluted and as a result, the effluent has a lower concentration of solutes than plasma (52).

where FF is filtration fraction, Qp is plasma flow expressed in mL/min, and QB is blood flow expressed in mL/min (EQUATIONS 40.6–40.8).



FF is the ratio of the ultrafiltrate to the plasma flow entering the hemofilter (FIGURE 40.2). The goal filtration fraction is <25% to reduce the chances of clotting. When FF is ≥25%, there is a greater chance of clotting. Raising the blood flow or reducing the ultrafiltration rate decreases the risk of clotting. Conversely, lowering the blood flow or increasing the ultrafiltration rate increases the risk of clotting. Furthermore, adding prefilter replacement lowers the filter fraction because the prefilter replacement fluid dilutes the plasma flow entering the hemofilter (49). To calculate FF, the prefilter replacement fluid is added to the denominator (EQUATION 40.9).
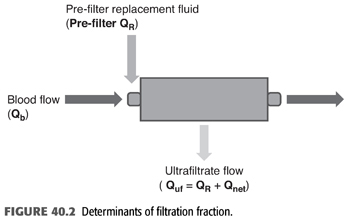

Example 1: Continuous Venovenous Hemodialysis
70-kg patient with CVVHD at QB 100 mL/min, Qd 1,500 mL/h. Net fluid removal by CVVHD was 2 L. Hematocrit is 30%; CE/CB or σ for urea = 1.
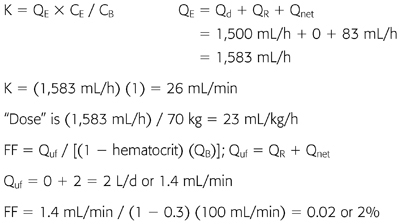
Example 2: Continuous Venovenous Hemofiltration with Postfilter Replacement
70-kg patient with CVVH at QB 100 mL/min, QR 1,500 mL/h (postfilter). Net fluid removal by CVVH was 0. Hematocrit is 30%; CE/CB or σ for urea = 1.
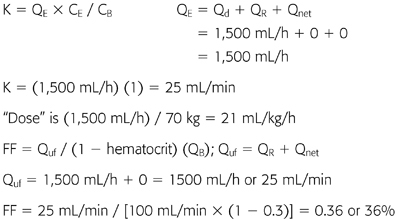
Example 3: Continuous Venovenous Hemofiltration with Prefilter Replacement
70-kg patient with CVVH at QB 100 mL/min, QR 1,500 mL/h (prefilter). Net fluid removal by CVVH was 0. Hematocrit is 30%; CE/CB or σ for urea = 1.
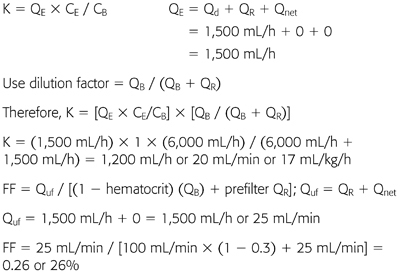
Example 4: Continuous Venovenous Hemodiafiltration
70-kg patient with CVVHDF at QB 100 mL/min, QR 1,500 mL/h (prefilter), Qd 1,500 mL/h. Net fluid removal by CVVHDF was 0. Hematocrit is 30%; CE/CB or σ for urea = 1.
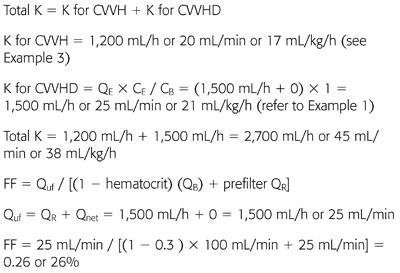
CLINICAL ISSUES OF RENAL REPLACEMENT THERAPY IN THE INTENSIVE CARE UNIT
There has been substantial advancement in regard to the optimal dosing of RRT through large multicenter, randomized clinical trials. However, the optimal timing of initiation of dialysis remains elusive, and the preferred modality of RRT in the ICU is somewhat controversial. This section of the chapter reviews through the literature discussing the issues surrounding the clinical applications of RRT in the ICU.
Indications for and Timing of Initiation of Renal Replacement Therapy
When first introduced into clinical practice in the 1940 to 1950s, RRT was mainly used to treat late and severe symptoms of kidney disease. Studies published during the 1960s to 1970s showed a worse mortality if RRT was started when blood urea nitrogen (BUN) exceeded 163 to 200 mg/dL versus BUN 93 to 150 mg/dL (55–57). The concept of “prophylactic” dialysis for AKI was first introduced by Teschan et al. (58) more than 50 years ago. They recommended starting dialysis before obvious clinical symptoms or biochemical derangements occur and they theorized that postponing dialysis can cause “retention of dialyzable substances which interfere with the functions of many cells and tissues,” resulting in irreversible damage (58). However, it is difficult to conclude the benefits of preemptive dialysis from older studies since many were observational studies, the study population was younger compared to current, and lastly, many would consider what was claimed to be early start with BUN ranging 90 to 150 mg/dL as rather late for initiation.
The indications for and optimal timing of initiation of RRT in patients with AKI has remained uncertain. There is little debate about initiation of RRT when there is severe volume overload, hyperkalemia, refractory metabolic acidosis, and overt uremic manifestations such as pericarditis. However, when there is no classic indication for RRT initiation, it is debatable whether starting dialysis “preemptively” will improve outcome. Some of the reasons to start dialysis preemptively are to improve volume control, restore acid–base homeostasis quicker, avoid situations for emergent “rescue” dialysis, and remove small middle-sized molecules. TABLE 40.4 outlines conventional, preemptive, and nonrenal indications for RRT.
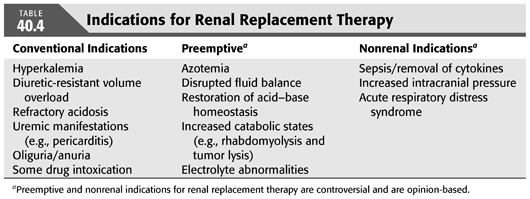
In this regard, it is worth reviewing key recent studies investigating the role of preemptive dialysis. Using the data from the Program to Improve Care in Acute Renal Disease (PICARD) cohort, which was a multicenter observational retrospective study, Liu et al. (59) analyzed 243 patients who required dialysis for AKI and subdivided the group by the level of BUN when dialysis was initiated. The higher degree of azotemia group was defined as BUN >76 mg/dL, and lower azotemia group was BUN ≤76 mg/dL. After multivariate adjustment for comorbid diseases and for propensity for initiation of RRT, late RRT was associated with a twofold risk of death at 60 days since the diagnosis of AKI (59). In another study, Vaara et al. (60) examined 239 patients who were started on RRT in the ICU using the prospective observational cohort from the Finnish Acute Kidney Injury Study Group. Patients were divided into those who were started on RRT for classic indications versus preemptive initiation. The classic group was further subdivided into delayed, which meant RRT was initiated >12 hours since the indication, versus urgent, which meant that RRT was initiated <12 hours. The crude 90-day mortality rate was 68% for the classic-delayed group, 39% for classic-urgent, and 30% for the preemptive group. The crude 90-day mortality rate was 49.3% for the non-RRT match equivalent to the preemptive group. Although initial studies reported decreased mortality with earlier initiation of renal support, the results are obscured by multiple limitations such as nonrandomization of groups and probable differences in the indications for initiation. More importantly, these studies did not include the analysis of patients with AKI who did not receive RRT because they either recovered renal function or died (60).
There are a few small-sized randomized controlled trials investigating the optimal timing of RRT. Study by Bouman et al. (61) was a prospective study of 106 critically ill patients with AKI randomized into three groups: early low-volume CVVH at average dose 20 mL/kg/h, early high-volume CVVH at average dose 48 mL/kg/h, and late low-volume CVVH at average dose 19 mL/kg/h. Early group started CVVH within 12 hours of oliguria after optimization of hemodynamics or measured creatinine clearance <20 mL/min on a 3-hour timed collection. The late group started CVVH when BUN was >112 mg/dL, hyperkalemia (K >6.5 mEq/L), or presence of pulmonary edema. There was no observed difference in 28-day survival (74.3%, 68.8%, 75%, respectively, p = 0.8) and renal recovery rate. Of the 36 patients who were randomized to the late low-volume group, 4 recovered kidney function and 2 died. This finding suggests that preemptive RRT may be unnecessary in some patients. Nevertheless, the study was underpowered to detect survival differences, and the overall high survival rate of these patients suggests that the study population is not representative of an average ICU.
Another study was an open label, randomized controlled trial of 208 patients with severe AKI in Western India. Patients were randomized to either early-start with serum creatinine >7 mg/dL or BUN 70 mg/dL versus usual-start for clinical indications as determined by the treating nephrologists (62). For the usual-start group, the mean starting BUN was 101 mg/dL and creatinine 10.4 mg/dL. There was no difference in-hospital mortality or risk of chronic dialysis at 3 months. Early-start group had 90-day mortality of 21% versus 12% for the usual-start, with relative risk ratio of 1.67 [95% confidence interval (CI) 0.88 to 3.17, p = 0.2]. Overall, dialysis dependence was reported in about 5% of the patients. The time to renal recovery of kidney function was significantly longer in the early-start group by 2 days. Limitations of the study include small sample size and that the study group was not representative of a typical ICU population. About half of the study population had tropical infections and obstetric complications. However, it is worth noting that 17% of patients in the usual-start group recovered kidney function before initiation of RRT (62).
Meta-analysis of 15 studies by Karvellas et al. (63) concluded that early initiation of RRT in ICU patients with AKI may have a favorable impact on survival. It should be recognized that the findings of this study were based on prospective and retrospective studies of varying quality and heterogeneity and that there were only two randomized controlled trials. Observational data showing improved outcomes may reflect inclusion of patients with a lesser degree of organ injury, whose outcomes would have been better regardless of treatment strategy. Some patients with severe AKI recovered kidney function, and if these patients were included in the “late” group, then the mortality for late group would be less. Furthermore, the argument that initiation of RRT early during the ICU stay is associated with increased survival may not be related to timing of initiation but rather due to the characteristics of patients who develop AKI early in the ICU stay compared to those who develop AKI several days or weeks after ICU admission. In addition, several of these studies used BUN as a surrogate marker for the duration of AKI (63). Yet, BUN is an imperfect surrogate marker for time and uremia because urea generation is not constant between patients or even within an individual patient over time.
While in some patients, preemptive RRT may improve outcome, it has been argued that a strategy of early initiation of RRT might expose patients to the risk of dialysis in those who would recover renal function with conservative therapy alone and may subject patients to further renal damage, delaying renal recovery (64). Repeated episodes of transient intradialytic hypotension can prolong AKI and reduce the chance of renal recovery (65). Although IHD may have higher likelihood of hemodynamic instability (65), episodes of hypotension can also occur with CRRT and SLED (2,66). Additionally, blood contact with dialyzer membrane may incite an inflammatory response that can exacerbate AKI, but this inflammatory stimulus was mostly caused by cellulose dialyzer membranes, which are now infrequently used (67).
Other potential risks of preemptive but unnecessary RRT in patients who would have had renal recovery are shown in TABLE 40.5. Patients are exposed to the risk of complications related to vascular access, subtherapeutic levels of essential medications, and electrolyte derangements. Medication dosing in AKI during RRT has been inadequately examined in particular for CRRT and SLED (64). Limited knowledge about proper dosing of antibiotics for CRRT can lead to suboptimal levels of antibiotics, which are potentially lifesaving in septic shock. Another complication of RRT is depletion of essential nutrients and electrolytes such as phosphorus. Hypophosphatemia is a common occurrence in patients on CRRT and has been linked to prolong recovery from critical illness (68).
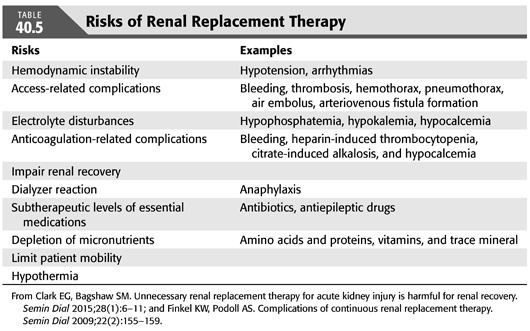
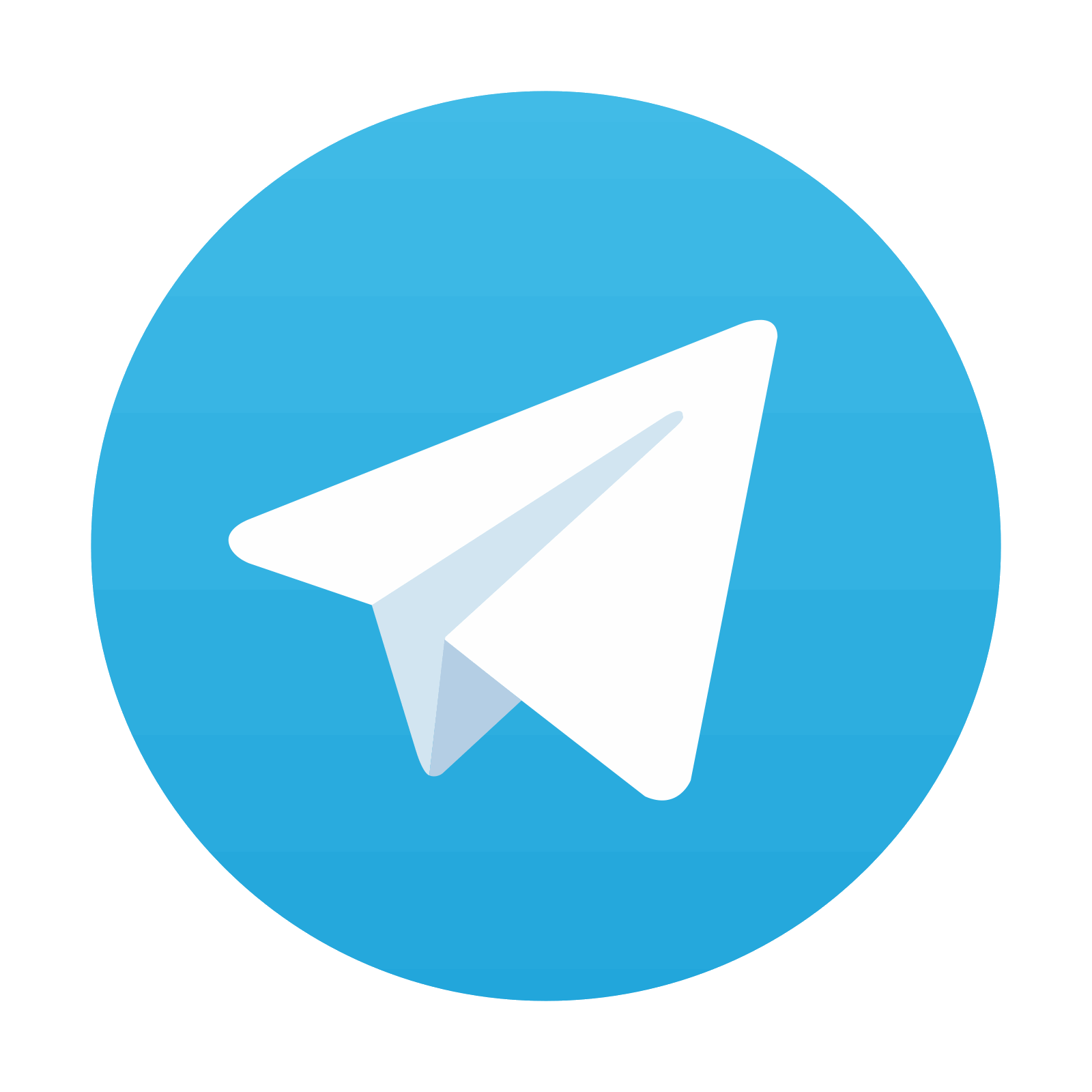
Stay updated, free articles. Join our Telegram channel
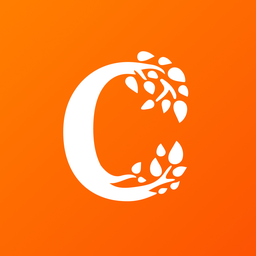
Full access? Get Clinical Tree
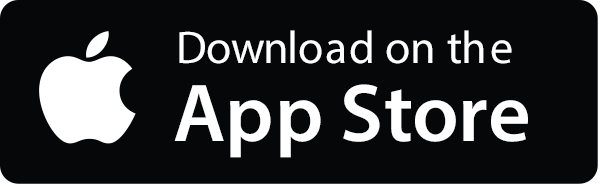
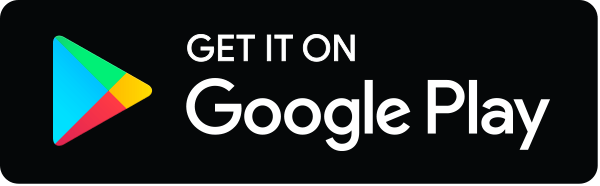