Recent years have marked an exciting time in the field of anion channel research. More and more of the molecular identities of the functional anion channel groups have been discovered and this new knowledge has given us an expanded view of the importance of anion channels in both kidney physiology and human physiology. So too have we been forced to acknowledge the shifting landscape that has redefined many anion channels as exchangers, and accept that perhaps the characteristics that separate the two groups are far less significant than previously believed.But what hasn’t changed is the importance of anion channels in kidney function. In this chapter, we march through the growing list of identified anion channels focusing on those with relevance in the kidney, but also taking time to discuss those anion channels still defined only by their biophysical characteristics. We end with an in-depth analysis of two prominent kidney diseases where anion channels play a significant role in the disease phenotype.The importance of anion channel function was late to be recognized but is now universally acknowledged as a fundamental component of kidney function.
Keywords
anion; chloride; Bartter’s syndrome; polycystic kidney disease
Introduction
The mammalian kidney evolved to allow for the efficient secretion of metabolic waste without excess loss of water in terrestrial environments, for the maintenance of the ionic balance in the extracellular milieu, for the regulation of blood pressure, and for the recapture of essential solutes like proteins and sugars. These functions all deferentially depended on the conductance/transport of Cl − and other anions across individual cell membranes. A classic example is NaCl reabsorption in the distal nephron, where Na + , Cl − , and K + enter the cell across the apical membrane via the NKCC2 co-transporter, driven by the Na + gradient established by the Na + /K + -ATPase, and the Cl − gradient maintained by basolateral ClC-Kb Cl − channels. Mutations in these basolateral Cl − channels result in the severe salt-wasting characteristic of Type III Bartter’s syndrome. As more of the major Cl − channel family molecular identities are resolved, our understanding of the role of Cl − channels in kidney disease will increase proportionally, and hopefully shed light on some of the long-standing mysteries of kidney function.
Although anions and negatively charged organic solutes were appreciated as necessary to balance the charges accumulated by the cations, Na + , K + , and Ca 2+ , they were believed to be passive players. Cl − , by far the most abundant physiological anion, was believed to be balanced across the membrane, a belief supported by early studies of skeletal muscle, where the resting Cl − permeability was found to be very high, and thus Cl − was mostly known for its contribution to the “leak” current. Even as later studies demonstrated that Cl − was being actively transported in squid axons or secreted as HCl in the stomach, less interest was given to anion conductances. Initial patch-clamp studies of Cl − channels discovered that Cl − channels are not really even Cl − channels; their general promiscuity toward all anions makes them more accurately described as anion channels. The tide first began to change as neuroscientists found, and later cloned, the GABA- and glycine-gated Cl − channels that worked to inhibit synaptic transmission, proving that Cl − was not in equilibrium at rest and could be harnessed by cells to do work. But it was the cloning of two major classes of Cl − channels, the CLCs and CFTR, which lead to an explosion of interest in Cl − channels, and exponentially increased our understanding of their ubiquity, importance in human disease, and function in the mammalian kidney.
Anion channels are usually grouped into five major classes, based on how they are activated or regulated. There are the voltage-dependent Cl − channels (the CLCs); ligand-gated Cl − channels (the GABA and glycine receptor channels); cAMP-activated channels (CFTR); Ca 2+ -activated Cl − channels (the TMEM16 channels); and the volume-regulated anion channels (the VRAC channels). This chapter will examine the expression and function of these major classes of Cl − channels in the kidney, but will also discuss at length Cl − channels that are expressed ubiquitously or for which expression and functional information does not yet exist. The chapter dives right in to survey the major anion channel families and their relevance in the kidney, focusing on the CLC, CFTR, TMEM16, VRAC, and SLC26A7/9 Cl − channels. However, the chapter will also look at a number of less well-understood anion channel genes that have currently unclear physiological relevance. Lastly, we will delve deeply into Cl − channelopathies that affect the kidney both directly and indirectly, seeking insight into how Cl − channels contribute to normal kidney physiology.
What’s “New”?
Recent work on Cl − channels has paid disproportionate dividends for Cl − channel researchers, including advances in our understanding of Cl − channel structure, molecular identity, and discovery of new Cl − channels in familiar but surprising places. The most important advance in Cl − channel knowledge came in 2008, when three independent groups using three novel approaches all identified the TMEM16A gene as the molecular identity of the classic Ca 2+ -activated Cl − channel (CaCC). The TMEM16 family of genes has 10 human homologs that are expressed widely, including in the kidney. As more and more work is done describing their function, biophysical characteristics, and expression patterns, the more certain it becomes that TMEM16a is the classic CaCC. Second, crystal structures solved for bacterial orthologs of the voltage-dependent CLC Cl − channel family revealed via homology that a number of the human CLCs (ClC-3, 4, 5, 6, and 7) are not in fact channels, but H + /Cl − exchangers, a finding with ramifications in the kidney. And third, two members of a well-known anion transporter family, SLC26A7 and A9, appear to behave almost exclusively as anion channels, with no appreciable transport of bicarbonate. Which, taken in conjunction with the recent reclassification of a number of the CLC channels as exchangers, suggests a fine line between anion transporter and anion channel. Each of the recent advances mentioned above will be discussed in depth in the following chapter.
Major Classes of Identified Anion Channels
Similar to the study of other ion channels, extensive research into the biophysical and physiological characteristics and functions of Cl − channels preceded the identification of their molecular identity or their corresponding human genes. One reason that the molecular identification of Cl − channels lagged far behind that of cation channels is that there were no highly specific inhibitors that could be used for cloning Cl − channels in a manner similar to that done for many cation channels. However, with a considerable amount of effort, more and more of the Cl − channels recognized for their biophysical characteristics now have mammalian gene candidates, identifications of which have quickly expanded our knowledge of the roles of Cl − channels in cellular functions and in human disease. The molecular identification has also shown just how difficult identification of all the Cl − channels is. There is no homology between any of the identified gene families containing Cl − channels, and very little commonality in structure. Their demonstrated convergence of function, so different from the common homology of many of the families of cation channels, presents no easy bioinformatic road map to the discovery of Cl − channel identities. Instead, a narrowly focused approach has been used on each of the functional classes of Cl − channels to identify the known Cl − channel families ( Figure 31.1 ), a growing list that now includes the CLC voltage-dependent channels, CFTR, TMEM16/Anoctamins Ca 2+ -activated Cl − channels, SLC26A7/9, GABA and glycine receptor channels, VDAC, and a myriad of less well-studied groups including the bestrophins, intracellular CLICs, CLCA, and tweety (hTTYH3). The long search for molecular identities of the Cl − channels has lead to many ambiguous discoveries, dead ends, and great success stories. Below we review the Cl − channels with known molecular identities, and known physiological and kidney specific functions, but we will also discuss those genes that code for proteins that can conduct Cl − , but do not yet have known physiological functions.

CLC Cl − Channels
Our discussion about the specific families of Cl − channels begins with CLC Cl − channels ( Figure 31.2 ). Unlike CFTR, which was not immediately recognized as a Cl − channel and appeared to be a very divergent type of ABC transporter, the canonical ClC-0 shared close homology with proteins from a large gene family with members expressed across all phyla, including nine members expressed in humans. Two decades of subsequent work have revealed much about the CLC family, their biophysical properties, and their importance in human disease, yet many of the mammalian orthologs still only have vaguely defined physiological functions. However, the relevance of the CLCs in the human kidney is clear. Eight of nine human CLC orthologs are expressed in the kidney, and their dysfunction leads directly to Dent’s disease and Type III Bartter’s syndrome.

For years the mammalian CLCs were divided into two classes, based on cellular localization with ClC-1, 2, and ClC-Ka, b channels being expressed in the plasma membranes, and the remaining ClC-3, 4, 5, 6, and 7 all being expressed on intracellular membranes. Recent evidence has substantially shifted this paradigm of understanding, with the plasma membrane CLCs now simply classified as the voltage-dependent Cl − channel CLCs with the rest now classified as Cl − /H + exchangers. It turns out that the vast majority of all CLC family members across all phyla are Cl − /H + exchangers and not Cl − channels. For the mammalian CLC proteins this has meant a revision of functions and physiological roles for the previously designated intracellular CLCs. Intrinsically, the difference between a CLC Cl − channel and a CLC Cl − /H + exchanger is little more than a few strategically placed glutamate residues; extrinsically, their thermodynamic properties are completely different. These newly classified exchangers will be covered briefly below, but this section will mostly focus on the remaining CLC Cl − channels and their functions in the kidney.
The early work investigating CLC Cl − channels found they have very distinct biophysical properties, which immediately set them apart from the known cation channels and would later set them apart from all other known Cl − channels. ClC-0 can be described as voltage-dependent, pH-dependent, and dependent on the Cl − gradient across the pore, as can most of the CLC Cl − channels. The “kidney” Cl − channels, ClC-Ka and ClC-Kb, are additionally dependent on a smaller beta-subunit, barttin (BSND); a dependence with ramifications for NaCl reuptake in the thick ascending loop and Bartter’s syndrome. The halide selectivity sequence for ClC-0 is Cl − >Br − >I − , and in most physiological circumstances it forms functional homodimers, although heterodimers from ClC-0, 1, and 2 can be formed heterologously. ClC-0 single channel currents appeared at first glance to be two separate channels, albeit with identical conductances; however, even though the two conductances or protopores appeared to open and close independently, sporadically they both closed simultaneously and remained closed for prolonged periods before opening again. The co-expression of ClC-0 and ClC-2 heterodimers resulted in the same strange phenomenon, but this time the two protopore conductances were different. The difference in protopore conductances correlated with the varying protopore size between ClC-0, 1, and 2, being 10 pS, 1.5 pS, and 3 pS respectively. This prompted the conclusion that ClC Cl − channels function as dimers, with two separate protopores, each independently gated by a separate “fast” gate, but both gated simultaneously by a “slow” gate.
This hypothesis was proven correct after the crystallization of the prokaryotic CLC proteins from E. coli and Salmonella typhimurium . These prokaryotic CLCs formed dimers, with each subunit forming its own protopore. Each subunit consists of 18 membrane “embedded” domains, where many of the helices do not project completely through the bilayer. Interestingly, each subunit has structurally similar halves that “twist” in an antiparallel structure that forms an hourglass shaped protopore toward the center. Further work on the E. coli CLC, EcClC-1, crystal revealed that there are three Cl − -binding sites within the hourglass shaped pore, and in the outermost site the Cl − appears to compete with the carboxyl group of a negatively-charged glutamate. When this glutamate is replaced with a non-charged amino acid in the ClC-0 channel, the voltage-dependent gating properties are altered. This suggests that the central “gating” glutamate is key in the voltage- and Cl − -dependence of CLC gating, and represents the fast gate regulating each protopore. The possible protonation of the gating glutamate, thereby allowing Cl − to enter the pore, may also help explain the pH-dependence observed for many CLCs.
The reconstitution of EcClC-1 in lipid bilayers revealed that it is not a Cl − channel, but a Cl − /H + exchanger, where two Cl − ions are substituted for one H + . Further mutational studies discovered the H + exchange depended on both the gating glutamate, and a second glutamate near the cytosolic surface, but not in the protopore. These characteristics suggest that both protons and Cl − are gated by the “gating” glutamate, after which they travel separate permeation paths to the cytoplasm. This theory is supported by experiments where this second glutamate was mutated in the EcClC-1, making the protein exclusively a Cl − channel, not an exchanger. The human CLCs ClC-3, 4, 5, 6, and 7 all have this second glutamate marking them as exchangers, and mutating both glutamates in ClC-4 and 5 converted them into Cl − channels, demonstrating just how fine the line is between Cl − channel and exchanger.
The pharmacology of CLC Cl − channels, like many of the other anion channels, has been plagued by the lack of specific high affinity inhibitors. Although the mammalian CLCs are highly conserved, they do differ somewhat in their susceptibility to the classic Cl − channel inhibitors. ClC-0, ClC-1, and ClC-2 all differ in their affinity for 9-anthracene carboxylic acid (9-AC), with ClC-1 effectively blocked by 100 µM, whereas ClC-0 and ClC-2 require mM levels. The CLCs are also affectively inhibited by the clofibric acid derivatives 2-(-p-chlorophenoxy) propionic acid (CPP) and 2-(-p-chlorophenoxy) acetic acid (CPA). Stilibenes can also be weakly inhibitory and can block ClC-2, as can DPC and NPPB at 0.5 to >1 mM concentrations. ClC-2 is also inhibited by divalent cations like zinc and cadmium with IC 50 in the µM range as the other CLC Cl − channels can be. Because of their human disease relevance the kidney CLCs, Ka and Kb have been scrutinized more than the others. Specifically, inhibitors could regulate dieresis and possibly blood pressure, and therapeutic activators could aid patients with Bartter’s Syndrome. The close shared homology of the ClC-Ks obviously suggested similar sensitivities to inhibitors, but both CPP and DIDS are more affective at blocking CLC-Ka than Kb. Niflumic acid (NFA), another classic Cl − channel inhibitor, was actually shown to activate the CLC-Ks at low concentrations (50–100 µM), but inhibit them at higher concentrations, a finding that prompted Liantonio et al. to manipulate the CPP structure to recapitulate characteristics of the NFA molecule, in order to create a better inhibitor. They found that their rationally designed benzofuran carboxyl acid derivatives could inhibit both ClC-Ka and Kb with low µM IC 50 s. Their work, and the insight provided by the EcClC-1 crystal structure, make finding potential therapeutic activators and inhibitors of the CLC-Ks a real likelihood, therapies that could impact millions of patients.
Physiological Function of Mammalian CLC Cl − Channels
With the recent identification of the intracellular CLCs as exchangers, only four human CLCs remain for further in-depth discussion: ClC-1, ClC-2, and the CLC-Ks. ClC-1 (the human gene is CLCN1 ), is the only CLC that is not expressed in the kidney. It is a plasma membrane localized Cl − channel found almost exclusively in skeletal muscle. There it represents about 80% of the resting conductance of the muscle. When the muscle depolarizes along the T-tubule, and Ca 2+ is released from the sarcoplasmic reticulum, ClC-1 is further activated. The ClC-1 activation repolarizes the muscle cell and brings it back to its negative resting potential. Cl − and not K + , as in most other excitable cells, is used for repolarization because it is thought that in the microdomains of T-tubules extracellular K + would quickly build up, causing unsolicited depolarizations. The physiological importance of ClC-1 became clear after causal ClC-1 mutations were found first in myotonic mice and later in humans. The human form of the disease, myotonia or “muscle stiffness” is characterized by muscles that suffer prolonged contractions, exactly the phenotype expected if repolarization cannot occur after the initial depolarizing stimulus. Humans can have either the recessive form of the disease (Becker type) with complete ClC-1 functionality loss or the dominant form (Thomsen’s) with the retention of some ClC-1 function and less severe debilitation.
ClC-2
ClC-2 is broadly expressed in many different tissues, but is prominent in epithelium (including kidney), neurons, glia, and heart muscle. Unlike ClC-1, ClC-2 is inwardly rectifying and hyperpolarization activated. It can also be activated by cell swelling, but is clearly not the ubiquitous VRAC current, which differs in selectivity, voltage-dependence, rectification, and single channel conductance. Unfortunately, little is yet known about its function. Even the development of Clnc2 −/− knockout mice has provided only minimal hints at its broad functions. Clcn2 −/− mice did display some disease phenotypes; however, knockout mice displayed degeneration of photo receptors and seminiferous tubules in testicles. There is also some evidence that ClC-2 is involved in Cl − secretion in lung epithelium, and that it could provide an alternative source of Cl − secretion in patients with aberrant CFTR function. To that end, the FDA approved lubiprostone, the only CLC-targeted drug yet approved, for treatment in cystic fibrosis (CF). More recent work suggests that ClC-2 may be localized more on the basolateral membrane and not apical, at least in gut epithelium, and that lubiprostome may also activate CFTR or other apical Cl − channels, not necessarily just ClC-2. Unfortunately, Clcn2 −/− mice have no kidney phenotype, even though ClC-2 is believed to be expressed in the proximal tubule. It is possible that, like in the gut, they may be expressed on the basolateral membrane and involved in the reabsorption of Cl − along the nephron, but to what extent is unclear.
CLC Exchangers
Before progressing to the in-depth analysis of the remaining kidney CLC Cl − channels, it is necessary to briefly touch on the CLC intracellular exchangers, in particular ClC-5 because of its relevance in kidney function. Like most other CLCs, ClC-5 is a Cl − /H + exchanger that exchanges two Cl − for every H + . ClC-5 is localized to the proximal tubule and to the α-intercalated cells of the cortical collecting duct. ClC-5 is specifically localized to the endosomes in these cell types, alongside the v-type H + -ATPase. The human ClC-5 gene CLCN5 was identified independently, first through positional cloning in families with Dent’s disease, and confirmed through homology cloning. Dent’s disease is an X-linked renal tubular disorder characterized by low molecular weight proteinuria (LMWP), hypercalciuria, nephrocalcinosis, kidney stones, and renal failure. More than 40 mutations have been identified in human CLCN5 that cause Dent’s disease, with a wide range of severity. Recapitulating some of the disease-causing mutations in ClC-5 expressed in heterologous systems showed they almost universally resulted in reduction or loss of Cl − conductance (ClC-5 when overexpressed reaches the plasma membrane). This, along with its known localization to subapical endosomes alongside H + -ATPases, prompted the hypothesis that ClC-5 was functioning to provide Cl − influx to offset the positive charge build-up from the acidification of the endosomes. A Cl − shunt would prevent a slowdown of H + pumping into the endosomes from positive charge build-up. Importantly, the then presumed channel characteristics of ClC-5 didn’t match this function well, ClC-5 is outwardly rectifying, and most importantly is inhibited by acidic pH. The creation of a Clcn5 −/− knockout mouse allowed the testing of the role ClC-5 plays in endosomal acidification. The two models both reproduced the LWMP and defective apical endocytosis, seemingly supporting the hypothesis that ClC-5 aids in acidification. In addition, in vitro disruption of ClC-5 function caused reduced acidification of the endosomes. But with the discovery that ClC-5 is not in fact a Cl − channel, but a Cl − /H + exchanger it became far less clear how it could provide the shunt current for acidification, and how it could be influencing the endocytosis of low molecular weight proteins. Recent work by Noarino et al. addressed this question directly by comparing mouse models with either Clcn5 −/− knockout or the knockin of a mutated Clcn5 (Clcn5unc) that removed the “gating” glutamate, uncoupling the Cl − /H + exchange and thereby creating a ClC-5 Cl − channel, not exchanger. They found, as reported previously, endosomes from the Clcn5 knockout showed reduced acidification and defective endocytosis; however, the Clcn5unc knockin mice showed normal acidification, but still had defective endocytosis. These data strongly suggest that ClC-5 plays a role in acidification by acting as a charge shunt; however, as a Cl − /H + exchanger it plays a further yet to be explained role in endocytosis unrelated to its role as a shunt. Novarino et al. suggest the intra-endosome absolute Cl − concentration may be key in regulating endocytosis, not just the negative charge.
CLC-Ks
There are two mammalian CLC-K channels, named because of their localization to kidney tissues, although they are now also known to be expressed in the inner ear. Mammals possess two CLC-Ks, ClC-K1 and K2, and the human orthologs are termed ClC-Ka and -Kb, respectively. The CLC-Ks are highly conserved with the rodent homologs, ClC-K1 and K2, sharing 80% homology and the human pair being over 90% homologous. After the homology cloning of rodent ClC-K1 and -K2, they were heterologously expressed in Xenopus oocytes, but only ClC-K1 expression resulted in Cl − currents. These ClC-K1 currents displayed a linear I/V relationship, with little voltage-dependence. Like the rodent ClC-K2 that failed to produce currents, the heterologous expression of the human ClC-Ka and -Kb failed to yield any Cl − currents, prompting the supposition that a β-subunit may be necessary for function. The discovery of the subunit came tangentially from the successful positional cloning of a gene mutated in human type IV Bartter’s syndrome, BSND, which codes for the protein barttin. Barttin has two transmembrane domains, and both N- and C-termini located in the cytoplasm. The expression of barttin along with either of the human CLC-Ks resulted in robust Cl − currents with ion selectivity similar to that of other CLC Cl − channels, inhibited by low extracellular pH, and stimulated by extracellular Ca 2+62 . The main function of barttin appears to be to increase dramatically the amount of CLC-Ks that make it to the plasma membrane, although it also appears to subtly alter gating. Interestingly, the voltage-dependence described for other CLCs is absent in the CLC-Ks, a phenomenon that appears connected to the “gating” glutamate described in the EcClC-1 exchanger; the two ClC-K channels in humans lack the gating glutamate and instead have a valine at the same position. However, if the valine is mutated to a glutamate, strong voltage dependence is recapitulated.
Although human CLC-Ks share close homology and do have overlapping kidney expression patterns, there does appear to be some divergence in localization that is supported by a divergence in function as well. ClC-K1 (and presumably ClC-Ka) is expressed in the cortex, inner, and outer medulla, specifically localized in the thin ascending limb (tAL) of the loop of Henle; ClC-K2 is expressed in the cortex, inner, and outer medulla, specifically localizing to the thick ascending limb (TAL), distal convoluted tubule, and the α-intercalated cells of the collecting duct. Barttin expression is co-localized with the two CLC-Ks. All three proteins, ClC-Ka, b, and barttin, are also expressed together in the stria vascularis of the inner ear.
Function of the CLC-Ks
The discussion on function will concentrate on three areas, the tAL, the TAL, and the inner ear. These are not the only areas of the nephron where the CLC-Ks have significant function, but they are the areas most directly attributed to their roles in human disease. An additional discussion of Bartter’s syndrome and CLC-Kb will come at the end of the chapter.
Currently there are no described human diseases with an isolated defect in the ClC-Ka gene, CLCNKA . Although there has been one report of an association between salt-sensitive hypertension and single nucleotide polymorphisms in humans CLCNKA , it is not clear how the SNPs or resulting haplotypes would alter ClC-Ka function. This lack of recognized human diseases from mutant ClC-Ka may be due to functional redundancies or poor sampling, because evidence has accumulated suggesting that ClC-Ka plays an important role in the urine concentrating mechanism of the loop of Henle. As mentioned above, ClC-K1 (Ka) is localized to the tAL, the area of the nephron with the highest Cl − permeability, and early studies comparing the characteristics of heterologously expressed ClC-K1/barttin currents versus those described from in vitro perfusion studies found striking similarities. Subsequent work demonstrated that ClC-K1 was expressed in both the basolateral and apical membranes of tAL epithelium, and interestingly, dehydration appears to increase the tAL expression of ClC-K1 in rats. This loose connection between volume sensing and ClC-K1 expression in the tAL may be through the skg1 protein kinase. The SKG1 gene and protein expression increases when the cell volume diminishes and, in turn, can upregulate ClC-K1/barttin currents. Barttin has a PY site on its C-terminus which facilitates the binding of WW domain-containing ubiquitin ligases like Nedd4-2; sgk1 can phosphorylate Nedd4-2, preventing the ubquitination of barttin and increasing the half-life of ClC-K1/barttin at the plasma membrane. Upregulation of Cl − vectorial transport in the tAL in times of dehydration would work to increase the concentrating mechanism of the inner medulla, and allow for increased water reabsorption via Aquaporin-2 in the collecting duct principle cells. However, it wasn’t until the creation of the Clcnk1 −/− knockout mouse that the physiological significance of ClC-K1 was clearly demonstrated.
Clcnk1 −/− mice display no physical phenotypes, no renal organ phenotype or changes in plasma creatinine, Na + , K + or HCO 3 − . However, they do display a four- to five-fold increase in the volume of excreted urine and other symptoms similar to the human condition nephrogenic diabetes insipidus (NDI). The concentrating of urine depends on the reuptake of water in the collecting duct, a process which in turn depends on the osmolarity gradient across the inner medulla. The high interstitial osmolarity of the inner medulla depends on the reabsorption of NaCl − and the recycling of urea in the tAL. The dysfunction of any part of the complicated concentrating mechanism will lead to diabetes insipidus, qualified as “nephrogenic” if the application of vasopressin (AVP) has no effect on the water lost in urine. The Clcnk1 −/− mice demonstrated an increase in urinary AVP secretion, no tAL Cl − transport, and reduction in papillary osmolarity due to decreased accumulation of NaCl and urea in the inner medulla. These results clearly and unambiguously demonstrated that ClC-K1 (Ka) is solely responsible for the vectorial Cl − transport of the tAL, and that it constitutes an important part of the urine concentrating mechanism.
The function of ClC-K2 (Kb) became clear after the mapping of human mutations in the CLCNKB in patients with Bartter’s syndrome, a condition that includes renal salt-wasting. This discovery, coupled with the localization of ClC-Kb to the TAL, prompted a model of NaCl reapportion in the TAL, where the ClC-K2 (Kb) is responsible for the basolateral Cl − conductance out of the cell. More specifically, in TAL cells, the apical membrane contains the NKCC2 co-transporter (SLC12A1) and ROMK K + channels; Na + , Cl − , and K + all enter the cell via the NKCC2, K + exits again apically via the ROMK channel, Na + is pumped into the interstitium via the Na + /K + -ATPase, and the Cl − moves down its gradient through basolateral ClC-Kb channels into the interstitium. This model is well-supported by the human genetics underlying Bartter’s syndrome, and will be discussed further at the end of the chapter. In contrast, gain-of-function mutations have also been identified in the human CLCNKB , most notably the T481S polymorphism prevalent in both African and Caucasian populations, with minor allele frequencies of 0.22 and 0.12 respectively. The T481S mutation in ClC-Kb increases currents 20-fold, possibly by alterations to the channels open probability, and was thought therefore to lead to increased salt reabsorption and thus hypertension; however, studies have mostly shown that there is no association between the T481S mutation and hypertension.
More recently, ClC-Ka, ClC-Kb, and barttin have all been shown to be expressed outside the kidney, in the stria vascularis of the inner ear. This was discovered only after a connection between deafness in some patients suffering from Bartter’s syndrome was made to a mutation in the barttin protein. Barttin and the CLC-Ks are expressed on the basolateral membrane of the stria vascularis epithelium, where they function to reduce the Cl − concentration in the stria vascularis. The fluid of the stria media has a very high concentration of K + , and an unusually low concentration of Na + . The excess K + is necessary to depolarize the hair cells via mechanosensory apical K + channels. The high K + is established by the movement of K + out of the stria vascularis epithelium via apical KCNQ1/KCNE1 K + channels. The K + enters the stria vascularis cells via basolateral Na + /K + -ATPases and the NKCC1 transporter, which imports two Cl − for every K + . Excess Na + is pumped out via the Na + /K + -ATPase and the Cl − is extruded through basolateral CLC-K channels. Human mutations in either one or the other CLC-K does not result in deafness, only in the dysfunction of their common barttin subunit or in very rare cases an individual has a mutation in both CLC-K genes. This suggests redundancy in function that may also occur in the kidney and help explain why human disease does not result from CLCNKA mutations. Intriguingly, individuals carrying at least one copy of the T481S ClC-Kb gain-of-function mutation mentioned above display a lower hearing threshold than wild-type individuals.
CFTR
Cystic fibrosis (CF) is the most common autosomal recessive genetic disease in Caucasians, and is debilitating and fatal; thus, long before the cloning of the Cystic Fibrosis Transmembrane Conductance Regulator (CFTR) gene, CF had been the focus of extensive physiological study. It was apparent that lung epithelium from patients with CF was lacking cAMP-activated Cl − conductance, as well as dramatically increased apical Na + conductance. These seemingly irreconcilable phenotypes resulted in the naming of the “CF” gene as a regulator of conductances, not as a Cl − channel. In fact, even after the successful cloning of the CFTR gene in 1989, it wasn’t until the creation of a CFTR −/− knockout mouse in 1993 that CFTR was definitively described as a Cl − channel. The CFTR −/− knockout demonstrated that there are actually two Cl − conductances that are dysfunctional in CF patients and in the CFTR −/− mouse, and that the CFTR biophysical characteristics did not match the large outwardly rectifying currents (ORCC) that were for years believed to be the dominant Cl − conductance. Instead, CFTR regulated these ORCC currents. Later work demonstrated that CFTR could regulate other channels like the epithelial sodium channel ENaC and the ATP sensitive K + channel ROMK2.
CFTR ( Figure 31.3 ) is now known to be a member of the ABC (ATP-binding cassette) superfamily of transporters; a vast gene family found in all phyla, responsible for transporting everything from metabolic products, lipids, and sterols, to drugs and uric acid. CFTR is a member of the C branch of the ABC family (ABCC7), characterized by having two large multi-helix transmembrane domains (TMD) and two intracellular nucleotide-binding domains (NBD). CFTR, uniquely among the ABC proteins, also possesses an extra, highly-charged cytoplasmic domain, termed the regulatory or “R” domain. Perhaps not coincidentally, CFTR is also the only ABC transporter, among 48 expressed in humans, shown to conduct Cl − . Both the N- and C-terminus of the protein are cytosolic, and the C-terminus contains the amino acid sequence DTRL, a sequence that is known to interact with the “PDZ” domains often found on scaffolding and linker proteins upon which regulatory protein complexes can be built. CFTR is therefore a single channel among a large family of anion transporters, much like the CLC and SLC26A gene families, further demonstrating the relative uniqueness of proteins that are Cl − channels and how many appear to be derivatives of exchangers or transporters.

CFTR can most aptly be characterized as activated by cAMP-dependent phosphorylation, although the activation of the channel is actually a complicated and slow process. First, the R domain needs to be phosphorylated by the catalytic subunit of protein kinase A (PKA) (activated directly by cAMP-binding) ; second, ATP needs to be bound (and hydrolyzed) within the conserved walker A- and B-binding sites of the NBD domains. Once activated, the Cl − currents show little or no voltage-dependence, display a linear I/V relation, show no Ca + -dependence, and have an anion selectivity sequence of Br − >Cl − >I − >F − . Single channel properties are reflective of the whole cell current characteristics: linear I/V relationship under symmetrical Cl − concentrations; 10–11 pS single channel conductance; activation by catalytic subunit of PKA; and channel-gating coupled to ATP hydrolysis.
In a reccurring theme that may be the single common characteristic among the various identified Cl − channels, for many years there was no identified high affinity or specific CFTR inhibitors. Common Cl − channel inhibitors like glibenclamide, DPC, NPPB, and niflumic acid provided low affinity pore blocking, but DIDS and other stilbene derivatives have little effect. In the CF lung there would be little use for CFTR inhibitors therapeutically; however, CFTR expression has also been linked to fluid secretion in the kidney (see discussion of polycystic kidney disease below) and in the human gut. Specifically, toxin from Cholera increases cAMP levels and induces secretory diarrhea, a loss of fluids and salts that can be lethal. The severity and pervasiveness of cholera has led to a substantial commitment of time and money to finding specific inhibitors of the cAMP-dependent Cl − secretion of the gut epithelium. High-throughput screens of small drug-like molecules, using a fluorescent halide-based assay has yielded two classes of specific CFTR inhibitors. The first class includes members of the 2-thioxo-4-thiazolidinone chemical class, in particular a compound named CFTRinh-172 with an IC 50 of 300 nM. CFTRinh-172 inhibits by blocking the intracellular pore in a voltage-independent fashion and prolongs the channels closed time, is non-toxic at high concentrations, is excreted with little metabolism, and has been shown to effectively reduce Cholera -induced fluid loss by 90% in mouse models. The second class of inhibitors includes the glycine hydrazides, and specifically GlyH-101. It appears to block the CFTR pore from the extracellular side, is voltage- and Cl − concentration-dependent, and in a closed loop model of cholera, GlyH-101 reduced fluid secretion by 80%. Both classes and their lead compounds, CFTRinh-172 and GlyH-101, appear to represent therapeutic avenues for cholera and polycystic kidney disease; however, the assays used to find them also present the possibility of finding CFTR activators. The past decade has seen the discovery of a number of correctors and potentiators, small molecules targeted to bind directly to the CFTR molecule and aid in folding or in changing the gating characteristics. A number of these compounds have begun clinical trials for treating CF patients, and represent a valid avenue for chronic CF therapy.
Before progressing to a discussion of CFTRs specific role in the kidney, it is appropriate to introduce CFTR function first in the context of the human lung. CFTR was originally cloned as the gene responsible for cystic fibrosis, a disease characterized by chronic lung infection, deteriorating lung function, pancreatic exocrine insufficiency, male infertility, meconium ileus, and gastrointestinal complications. In each phenotype the dysfunction stems from the reduction in fluid secretion at each relevant epithelium. CFTR is normally apically localized, and when activated Cl − leaves the cells and enters the lumen or airway this drives paracellular Na + , leading to water movement into the lumen. In the lung, the loss of the CFTR Cl − conductance lowers the airway surface liquid volume (ASL), reduces the activity of the epithelial cilia, and reduces or abolishes the mucus clearance, leading to chronic infection.
Currently, over 1500 CFTR mutations have been found in CF patients, representing a spectrum of disease severity directly proportional to the level of residual CFTR function. Null mutations result in no protein being produced, whereas other mutations can result in minor changes to channel-gating or cell surface expression. By far the most common CFTR mutation is the deletion of phenylalanine residue at position 508 (Δ508), carried by 90% of CF patients, a mutation that causes misprocessing and trafficking, and results in no CFTR being expressed at the cell surface. Human CFTR mutations have been classified based on their severity into five classes. Class I mutants produce no CFTR, Class II includes the folding and processing mutants like Δ508, and Class III mutants produce CFTR that is trafficked to the membrane but have dysfunctional Cl − conductance; all three classes result in severe lung disease and pancreatic insufficiency. Class IV mutants display less pronounced conductance abnormalities and often have no lung phenotypes, and Class V mutants are often asymptomatic and diagnosed only after they are found to be infertile or present with idiopathic pancreatitis.
CFTR in the Kidney
CFTR expression is abundant in the human kidney. Early studies looking at CFTR mRNA or using RT-PCR found evidence for CFTR expression generally in the cortex and outer medulla. More specifically, CFTR was found all along the nephron, including proximal and distal tubules, thin and thick ascending limbs, and the collecting duct. Immunostaining of CFTR protein showed CFTR expression again in the proximal and distal tubules, the tAL, and the cortical and inner medullary collecting ducts. Functional studies using patch-clamp have identified CFTR-like currents in the proximal and distal tubules and the collecting ducts. CFTR is also known to be expressed in the embryonic kidney, with upregulation in the ureteric bud during the later stages of branching morphogenesis. Intriguingly, a large proportion of the overall CFTR expression in the kidney consists of a CFTR splice variant termed TNR-CFTR. The TNR-CFTR mRNA is missing the final 145 bp including exons 13 and 14, creating an early stop codon. The splicing may be regulated by snRNAs U11 and U12, which co-localize to the same areas of the kidney as the TNR-CFTR isoform. This truncation corresponds to a loss of the second TMD and NBD, as well as 7% of the R domain in the final protein. The TNR-CFTR is therefore a “half” transporter, similar to many other members of the ABC family, and like those other ABC transporters, the remaining half molecule is enough to give the protein function. When expressed in Xenopus oocytes the TNR-CFTR is a functional Cl − channel, is still activated my cAMP and PKA, and possesses many of the same biophysical traits as the wild-type CFTR. Its processing, however, seems less efficient, and little makes it to the plasma membrane, but instead it localizes to intracellular vesicles. More generally, TNR-CFTR is found mostly in the renal medulla, and has an interesting developmental expression pattern. In mice, the expression of TNR-CFTR increases throughout embryonic kidney development, reaching its highest level at birth. The functional significance of TNR-CFTR remains a complete mystery.
CFTR as a Kidney Cl − Channel
Although the expression levels of CFTR in kidney are comparable to those of the lung, there is no clear CF kidney phenotype, with the exception of increased risk of hyperoxaluria or insufficient citrate-induced kidney stones; a fact that many have taken as evidence that CFTR is not very important in salt handling in the kidney, and yet CFTR is known to play important roles in renal diseases like ADPKD. Many have attributed the lack of phenotypes to a redundancy in Cl − channels present in the kidney. Mouse CFTR −/− knockout models often show little or no lung CF phenotype, a discrepancy explained by the upregulation of Ca 2+ -activated Cl − channels (TMEM16s; ) to compensate for the loss of CFTR Cl − conductances. Likewise in the kidney, other types of Cl − channels could be compensating for the missing functional CFTR in CF patients. However, CFTRs functional role in the kidney could be more regulatory, thus CF renal phenotypes more subtle. Recent work looking at CFTR in the apical membrane of mouse cortical collecting duct principal cells demonstrates the difficulty in establishing CFTRs function as a Cl − channel and not as a regulator of other channels. Lu et al. definitively demonstrated CFTR Cl − channel activity on the apical membrane of mouse principal cells, and plausibly suggest that it could represent a short-circuit current meant to regulate Na + absorption and K + secretion. However, they concede that it is just as likely that phosphorylation of CFTR could act as a modulatory switch on ROMK activity, and thus exert an influence on K + secretion. To complicate matters further, the prevailing model of ion movement in principal cells has Cl − being reabsorbed mainly through a paracellular pathway, suggesting that for CFTR to have an effect it would need to represent a very large conductance, much larger than that measured by Lu et al. in mouse principal cells. In fact, there is ample evidence that in the kidney and elsewhere CFTRs main function may be to regulate other ion channels.
CFTR as a Regulator of ENaC
From before its initial molecular identification, CFTR has been shown to regulate an ORCC Cl − conductance and a Na + conductance. In the airway of CF patients (but not in the sweat ducts), increased Na + absorption via the epithelial sodium channel, ENaC, is observed due to loss of the normal CFTR-mediated inhibition; however, the mechanism of regulation remains unclear. Currently, there are multiple hypotheses to explain how CFTR regulates ENaC. One possibility is that intracellular Cl − regulates ENaC activity. This is supported by data showing that CFTR Cl − conductance is necessary for ENaC regulation, and in some but not all cell types replacing CFTR Cl − current with CLC-mediated Cl − currents can also inhibit ENaC currents. These data are conflicted by the lack of identified Cl − -binding sites on ENaCs intracellular domains, and by studies showing that alternative Cl − channels like CaCCs cannot inhibit ENaC.
A second theory proposes CFTR exerts its regulatory effects through intermediary proteins as part of a large protein complex. CFTR contains a PDZ-binding domain that enables it to interact with linking proteins like ezrin-binding phosphoprotein 50 (EB50) and NHERF1. NHERF1, through its PZD2 region, can bind YAP65 which recognizes the PY motif on the C-terminus of ENaC, creating a complex with both CFTR and ENaC. C-Yes, a c-Src kinase, is a known inhibitor of ENaC and can bind to YAP65. Thus, CFTR, in aiding the assembly of the protein complex, inhibits the activity of ENaC and the dissolution of the complex, e.g., from CFTR dysfunction or mutation, liberates ENaC from C-Yes inhibition. However, this possibility has not been demonstrated in heterologous expression systems.
A third possibility involves CFTR interacting directly with ENaC to cause inhibition. Patch-clamp studies of ENaC subunits and CFTR in proteoliposomes, presumably free of contaminating complex proteins, showed that CFTR directly affects the gating of ENaC single channels. FRET studies have demonstrated an association of less than 10 nM of the two proteins in vitro , and co-immunoprecipitation experiments show the two physically interact. However, with the exception of the single channel results, the evidence does not strictly rule out the possibility of CFTR and ENaC interacting indirectly as part of a protein complex.
The relationship between CFTR and ENaC in the kidney appears as conflicting as that found for the airways and seat ducts. Some work has shown that CFTR inhibits ENaC in the kidney, similar to the relationship found in airways. Experiments in M-1 cortical collecting duct cells showed that activation of PKA reduced the amiloride-sensitive apical Na + current. However, other studies have found CCD cells from mice carrying a mutation in ENaC, known to cause Liddles’s syndrome (deletion of the PY motif in the c-terminus as the β-subunit ENaC), display increased Na + absorption as compared to wild-type when treated with dD-arginine vasopressin. A similar increase is seen in the CFTR-mediated apical current, implying vasopressin causes increases in both conductances, an idea consistent with the cAMP-induced increases in both CFTR and ENaC seen in sweat ducts. Simply put, the evidence for CFTR regulating ENaC in the kidney is sparse, and it remains unknown whether or not it acts as an inhibitor, activator or neither. It may require a final determination of how CFTR regulates ENaC in airway tissue before a renewed focus can be brought on CFTR and ENaC in the kidney.
CFTR as a Regulator of ROMK
The inward rectifier potassium channel ROMK (Kir1.1) and its isoforms are expressed highly in the distal nephron, where it plays a major role in ATP sensitive K + secretion. Some of their defining traits are lack of sensitivity to the common K + channel blocker tetraethylammonium (TEA), and inhibition by ATP and sulfonylureas like glibenclamide. But when the ROMK2 isoform was expressed in the Xenopus oocytes, it was no longer sensitive to glibenclamide. Other ATP-sensitive K + channels require ABC transporter subunits, SUR1 and SUR2 (ABCC8 and ABCC9), for glibenclamide sensitivity, but neither SUR1 nor SUR2 is known to be expressed in the kidney. However, CFTR is expressed in the same distal nephron cells as ROMK channels, and when CFTR and ROMK2 are co-expressed in Xenopus oocytes, the glibenclamide sensitivity is restored. Subsequent work has demonstrated that CFTR is also necessary for ROMK’s sensitivity to Mg-ATP in CCD mouse cells. Lu et al. hypothesize that water diuresis and the resulting low cAMP-PKA would promote the CFTR–ROMK interaction, and so cytoplasmic ATP would inhibit excessive K + secretion. However, during antidiuresis when PKA levels are high, the phosphorylation of CFTR will free ROMK from ATP sensitivity and promote K + secretion. The specifics of the ROMK–CFTR interaction are unknown, but it is probably similar to the better-understood Kir6.x/SUR complex of pancreatic β-cells. Kir6.x-subunits form tetramers, with each subunit associated with a SURx subunit to create an eight unit complex. Although the CFTR regulation of ROMK appears functionally very important, there is no reported K + secretion phenotype in the distal nephron in CF patients.
CFTR as a Regulator of SLC26As
CFTR is also a demonstrated regulator of transporters from the SLC family of anion transporters. Earlier research showed that CFTR could interact with and activate SLC26A3 and A6, conferring cAMP sensitivity on these two transporters. In the pancreatic ducts, HCO 3 secretion is a critical component of the function of the secretory epithelium, a function disrupted in patients with severe CF. Wang et al. showed specifically that the SLC26A6 HCO 3 − /Cl − exchanger was critical for HCO 3 − secretion, and that its function was regulated by co-localized CFTR expression in the luminal membrane. Further work demonstrated that a conserved region of the SLC26A transporters, the STAS domain, interacted directly with the R domain of CFTR. The binding of the STAS and R domains activates SLC26A6, but also dramatically increase the Cl − conductance of CFTR by increasing the channels open probability six-fold. Interestingly, SLC26A6 is expressed throughout the kidney, but appears most prominently in the apical–luminal membrane of the proximal tubule where it is responsible for Cl − , HCO 3 − , oxalate, and formate exchange. CFTR expression is also reported in the proximal tubule, and although there is no current evidence of their interaction in the kidney it seems plausible.
CFTR more recently has also been demonstrated to be a regulator of one of the two true Cl − channel members of the SLC26A family, SLC26A9. Both SLC26A9 and CFTR co-localize to the apical membrane of human bronchial epithelial (HBE) cells, where cAMP leads to the co-activation of SLC26A9 and CFTR Cl − channels. The nature of the CFTR–SLC26A9 interaction is similar to the other CFTR–SLC interactions, the conserved STAS domain of SLC26A9 binds directly to the R domain of CFTR. However, there are conflicting reports as to the exact regulatory nature of the CFTR–SLC26A9 relationship. Bertrand et al. report that endogenous SLC26 currents in HBE cells are activated by functional CFTR; whereas Chang et al. report heterologous co-expression of an NBD+R domain CFTR construct with SLC26A9 in Xenopus oocytes results in the inhibition of SLC26A9 currents. What is clear is that the conserved STAS domain of the SLC26A channels and transporters strongly interacts with CFTR, an interaction that may extend to many of the SLC26A members. SLC26A9 is expressed mainly in the lung, but the other described SLC26A pure Cl − channel, SLC26A7, is expressed throughout the kidney, and in particular cells of the cortical collecting ducts, an area also high in CFTR expression. The role of a possible interaction between SLC26A7 and CFTR in the cortical collecting duct is an important area for future investigation.
CFTR and Endocytosis
A new area of CFTR research has begun investigating CFTRs role in acidification of endosomes of the proximal tubule. Generally, CF patients have shown increased renal clearance of some drugs including aminoglycosides, and more specifically a cohort of patients with at least one copy of the Δ508 CFTR mutation showed significantly higher levels of albuminuria and LMW proteinuria. CFTR −/− knockout mice have significantly increased excretion of LMW Clara Cell protein (CC16), and decreased uptake of radio-labeled I-β2microglobulin and aminoglycosides, similar to human CF patients. Significantly, the CFTR −/− mice also demonstrated decreased cubilin expression in the S3 segment of the proximal tubule, a finding correlating with the observed increase of cubilin excretion. Cubilin is a lipoprotein receptor and, along with megalin, is critical for the uptake of LMW proteins. In the CFTR −/− mouse, however, megalin is not affected, thus it appears CFTR is specifically reducing cubilin expression. Cubilin recycling to the apical membrane via endosomes is critical to maintain apical cubilin expression and for LWM protein uptake. The sorting and recycling of endosomes is pH-dependent. CFTR has been localized to the apical region in proximal tubule cells and specifically it co-distributes with ClC-5 and Rab5a in endosomes, where it is proposed to aid in providing a negative current shunt for the interior of the endosomes. CFTR would offset the build-up of positively-charged H + during acidification, a role similar to that proposed for the Cl − /H + exchanger ClC-5. The evidence of CFTRs involvement in receptor-mediated endocytosis from CF patient studies and CFTR −/− mice is compelling, but the mechanism remains unconfirmed, although it seems likely CFTR, along with ClC-5 and possibly other Cl − channels, all contribute to the pH regulation of the proximal tubule endosomes.
Ca 2+ -Activated Cl − Channels (CaCC): TMEM16/Anoctamin
For 30 years Ca 2+ -activated Cl − channels (CaCC) with similar biophysical characteristics have been studied in a wide range of tissue and cell types, including secretory epithelia, egg cells, cardiac, smooth, and skeletal muscles, neurons, olfactory and photoreceptors, hepatocytes, and beta cells. The classic CaCC characteristics are best described by the endogenous CaCCs of Xenopus oocytes or the CaCCs of acinar cells in the salivary gland ( Figure 31.4 ). These classic CaCC channels are activated by small amounts of cytosolic Ca 2+ (0.2–5 µM) either from extracellular influx or store release. Activation appears to occur through direct binding of Ca 2+ to the channel. When cytosolic Ca 2+ is limiting, CaCC shows an outward rectifying I/V relationship, but as cytosolic Ca 2+ increases the rectification diminishes. The open probability appears to be voltage-dependent and the single channel conductance is small 1–3 pS, although a variety of conductance sizes has been reported. Classic CaCCs, like other anion channels, are relatively non-selective, with Na + permeability being roughly 10% that of Cl − . Among anions the selectivity sequence is SCN − >NO 3 − >I − >Br − >Cl − >F − .
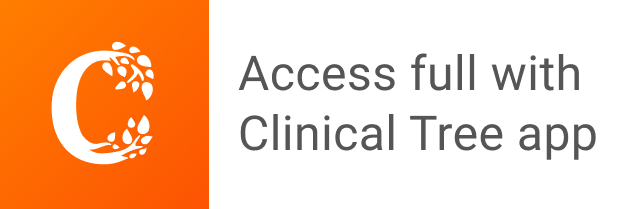