Chapter Outline
GROSS FEATURES, 42
THE NEPHRON, 44
Renal Corpuscle, 45
Endothelial Cells, 46
Glomerular Basement Membrane, 48
Visceral Epithelial Cells, 49
Mesangial Cells, 51
Parietal Epithelial Cells, 52
Peripolar Cells, 53
Juxtaglomerular Apparatus, 53
Juxtaglomerular Granular Cells, 53
Extraglomerular Mesangium, 53
Macula Densa, 54
Autonomic Innervation, 54
Tubuloglomerular Feedback, 55
Proximal Tubule, 55
Pars Convoluta, 55
Pars Recta, 60
Thin Limbs of the Loop of Henle, 61
Distal Tubule, 63
Thick Ascending Limb, 63
Distal Convoluted Tubule, 65
Connecting Tubule, 68
COLLECTING DUCT, 69
Cortical Collecting Duct, 70
Outer Medullary Collecting Duct, 73
Inner Medullary Collecting Duct, 74
INTERSTITIUM, 75
LYMPHATICS, 80
INNERVATION, 80
Knowledge of the complex structure of the mammalian kidney provides a basis for understanding the multitude of functional characteristics of this organ in both healthy and diseased states. In this chapter, an overview of the renal organization is presented through gross anatomical observations and ultrastructural information. Examples of the expression of selected molecules, such as channels, transporters, and regulatory proteins, are also provided, although this topic is covered in detail in later chapters.
Gross Features
The kidneys are paired retroperitoneal organs normally situated one on each side of the vertebral column. In the human, the upper pole of each kidney lies at a level corresponding to the twelfth thoracic vertebra, and the lower pole lies corresponding to the third lumbar vertebra. The right kidney is usually slightly more caudal in position than the left. The weight of a human kidney ranges from 125 to 170 g in the adult male and from 115 to 155 g in the adult female. The human kidney is approximately 11 to 12 cm in length, 5.0 to 7.5 cm in width, and 2.5 to 3.0 cm in thickness. When renal magnetic resonance imaging (MRI) is performed, the ranges of normal reference values (mean ± 2 SD) for male and female kidney lengths are 10.7 to 14.3 and 9.5 to 13.9 cm, respectively, and the ranges for male and female kidney volumes are 132 to 276 and 87 to 223 mL, respectively. Located on the medial or concave surface of each kidney is a slit, called the hilum, through which the renal pelvis, the renal artery and vein, the lymphatics, and a nerve plexus pass into the sinus of the kidney. The organ is surrounded by a thin tough fibrous capsule, which is smooth and easily removable under normal conditions.
In the human, as in most mammals, each kidney is normally supplied by a single renal artery, although the presence of one or more accessory renal arteries is not uncommon. The renal artery enters the hilar region and usually divides to form an anterior and a posterior branch. Three segmental or lobar arteries arise from the anterior branch and supply the upper, middle, and lower thirds of the anterior surface of the kidney ( Figure 2.1 ). The posterior branch supplies more than half of the posterior surface and occasionally gives rise to a small apical segmental branch. However, the apical segmental or lobar branch arises most commonly from the anterior division. No collateral circulation has been demonstrated between individual segmental or lobar arteries or their subdivisions. The kidneys often receive aberrant arteries from the superior mesenteric, suprarenal, testicular, or ovarian arteries. True accessory arteries that arise from the abdominal aorta usually supply the lower pole of the kidney. The arterial and venous circulations in the kidney are described in detail in Chapter 3 and are not discussed further in this chapter.

Two distinct regions can be identified on the cut surface of a bisected kidney: a pale outer region, the cortex, and a darker inner region, the medulla ( Figure 2.2 ). In humans, the medulla is divided into 8 to 18 striated conical masses, the renal pyramids. The base of each pyramid is positioned at the corticomedullary boundary, and the apex extends toward the renal pelvis to form a papilla. On the tip of each papilla are 10 to 25 small openings that represent the distal ends of the collecting ducts (ducts of Bellini). These openings form the area cribrosa ( Figure 2.3 ). A renal pyramid and the corresponding cortex are referred to as a renal lobus. In contrast to the human kidney, the kidney of the rat and of many other laboratory animals has a single renal pyramid with its overlying cortex and is therefore termed “unipapillate.” Otherwise, these kidneys resemble the human kidney in their gross appearance. In humans, the renal cortex is about 1 cm in thickness, forms a cap over the base of each renal pyramid, and extends downward between the individual pyramids to form the renal columns of Bertin (see Figures 2.2 and 2.4 ). From the base of the renal pyramid, at the corticomedullary junction, longitudinal elements termed the “medullary rays of Ferrein” extend into the cortex. Despite their name, the medullary rays are actually considered a part of the cortex and are formed by the collecting ducts and the straight segments of the proximal and distal tubules.



In humans, the renal pelvis is lined by transitional epithelium and represents the expanded portion of the upper urinary tract. In rodents, the epithelium closely resembles that of the terminal part of the collecting duct. Two and sometimes three protrusions, the major calyces, extend outward from the upper dilated end of the renal pelvis. From each of the major calyces, several minor calyces extend toward the papillae of the pyramids and drain the urine formed by each pyramidal unit. In mammals possessing a unipapillate kidney, the papilla is directly surrounded by the renal pelvis. The ureters originate from the lower portion of the renal pelvis at the ureteropelvic junction, and in humans they descend a distance of approximately 28 to 34 cm to open into the fundus of the bladder. The mean size of ureters in adults is 1.8 mm, with a maximum width of 3 mm considered normal. The papilla, the walls of the calyces, pelvis, and ureters contain smooth muscle that contracts rhythmically to propel the urine to the bladder.
The Nephron
The nephron is often referred to as the functional unit of the kidney. Although the average nephron number in adult humans is approximately 900,000 to 1 million per kidney, numbers for individual kidneys range from approximately 200,000 to more than 2.5 million, contrasting with the approximately 30,000 nephrons in each adult rat kidney. The essential components of the nephron include the renal or malpighian corpuscle (comprising the glomerulus and Bowman’s capsule), the proximal tubule, the thin limbs, the distal tubule, and the connecting tubule ( Figure 2.5 ). The origin of the nephron is the metanephric blastema. Although there has not been universal agreement on the origin of the connecting tubule, it is now generally believed also to derive from the metanephric blastema. The collecting duct system, which includes the initial collecting tubule/duct, the cortical collecting duct (CCD), the outer medullary collecting duct (OMCD), and the inner medullary collecting duct (IMCD), is not considered part of the nephron, because it arises embryologically from the ureteric bud. However, all of the components of the nephron and the collecting duct system are functionally interrelated. An alternative structural/functional separation and a way to circumvent confusion in the literature are to use the terms (1) the renal corpuscle and (2) the renal tubular system.

Several populations of nephrons are recognizable in the kidney with varying length of the loop of Henle (see Figure 2.5 ). The loop of Henle is composed of the straight portion of the proximal tubule (pars recta), the thin limb segments, and the straight portion of the distal tubule (thick ascending limb [TAL], or pars recta). The length of the loop of Henle is generally related to the position of its parent renal corpuscle in the cortex. Most nephrons originating from superficial and midcortical locations have shorter loops of Henle that bend within the inner stripe of the outer medulla close to the inner medulla. A few species, including humans, also possess cortical nephrons with extremely short loops that never enter the medulla but turn back within the cortex. Nephrons originating from the juxtamedullary region near the corticomedullary boundary have long loops of Henle with long descending and ascending thin limb segments that enter the inner medulla. Many variations exist, however, between the two basic types of nephrons, depending on their relative positions in the cortex. Three-dimensional (3D) reconstruction studies of the rat and mouse kidney have provided insight into the anatomical distribution of various nephrons. These studies have highlighted that the ratio of long and short loop nephrons varies among species, with humans and rodents having a larger number of short looped than long looped nephrons. Owing to these anatomical differences, caution should be exercised in interpretation of micropuncture data for understanding the urinary concentrating mechanism, because the majority of this data arises from studies on short-looped nephrons.
On the basis of the segmentation of the renal tubules, the medulla can be divided into an inner zone and an outer zone, with the outer zone further subdivided into an inner stripe and an outer stripe (see Figure 2.5 ). The inner medulla contains both descending and ascending thin limbs and large collecting ducts, including the ducts of Bellini. In the inner stripe of the outer medulla, TALs are present in addition to descending thin limbs and collecting ducts. The outer stripe of the outer medulla of the human kidney contains the terminal segments of the pars recta of the proximal tubule, the TALs (pars recta of the distal tubule), and collecting ducts. By contrast, the renal cortex contains the renal corpuscles, segments of proximal tubules, and distal tubules as well as collecting ducts, but does not contain thin limbs of Henle’s loop. The division of the kidney into cortical and medullary zones and the further subdivision of the medulla into inner and outer zones are of considerable importance in relating renal structure to the ability of an animal to form maximally concentrated urine.
Renal Corpuscle
The initial part of the nephron is the renal corpuscle, which is composed of a capillary network lined by a thin layer of endothelial cells (glomerulus), a central region of mesangial cells with surrounding matrix material, the visceral epithelial layer of Bowman’s capsule and the associated basement membrane, and the parietal layer of Bowman’s capsule with its basement membrane ( Figures 2.6 through 2.8 ). The Bowman’s space, or the urinary space, is a narrow cavity between the two epithelial cell layers. Although renal corpuscle is anatomically correct when used to refer to the portion of the nephron composed of the glomerular tuft and Bowman’s capsule, glomerulus is employed throughout this chapter because of its common use. At the vascular pole, the visceral epithelium is continuous with the parietal epithelium. This is where the afferent arteriole enters and the efferent arteriole exits the glomerulus. The parietal layer of Bowman’s capsule continues into the epithelium of the proximal tubule at the so-called urinary pole. The average diameter of a glomerulus is approximately 200 µm in the human kidney and 120 µm in the rat kidney. However, the number of glomeruli and their size varies significantly with age, gender, birth weight, and renal health. The average glomerular volume is 3 to 7 million µm 3 in humans and 0.6 to 1 million µm 3 in rats. Rat juxtamedullary glomeruli are larger than glomeruli in the superficial cortex; this is not the case in the human kidney.



The main function of the glomerulus is production of an ultrafiltrate from plasma. The fenestrated endothelium, the peripheral glomerular basement membrane (GBM), and the slit pores between the foot processes of the visceral epithelial cells form the filtration barrier between the blood and the urinary space ( Figure 2.9 ). In the human kidney, mean area of filtration surface per glomerulus is 0.203 mm 2 , and in the rat kidney, 0.184 mm 2 . Although the glomerular capillary wall functions as a “sieve” to allow passage of small molecules, it restricts the passage of larger molecules, such as albumin. The glomerular capillary wall possesses both size-selective and charge-selective properties. To cross the capillary wall, a molecule must pass sequentially through the fenestrated endothelium, the GBM, and the epithelial slit diaphragm. The fenestrated endothelium, possessing a negatively charged glycocalyx, excludes formed elements of the blood and is important for determining access of proteins to the GBM.

Endothelial Cells
The glomerular capillaries are lined by a thin fenestrated endothelium (see Figures 2.9 and 2.10 ). These endothelial cells form the initial barrier to the passage of blood constituents from the capillary lumen to Bowman’s space. Under normal conditions, the formed elements of the blood, including erythrocytes, leukocytes, and platelets, do not gain access to the subendothelial space.

The endothelial cell nucleus lies adjacent to the mesangium, with the remainder of the cell irregularly attenuated around the capillary lumen (see Figure 2.8 ). The endothelium contains pores or fenestrae that range from 70 to 100 nm in diameter in human (see Figure 2.10 ). Thin protein diaphragms extend across these fenestrae, and filamentous sieve plugs have been observed in the fenestrae. The function of these plugs remains to be fully established, and it is not known whether they represent a significant barrier to the passage of molecules. However, adult glomerular endothelial cells have been reported to lack these diaphragms, and instead diaphragmed fenestra are present predominantly in the embryo, where they may compensate for the functional immaturity of the embryonic glomerular filtration barrier. Electron-dense filamentous material in the fenestrae and a thick filamentous surface layer on the endothelial cells have also been demonstrated. Nonfenestrated, ridgelike structures termed cytofolds are found near the cell borders.
An extensive network of intermediate filaments and microtubules is present in the endothelial cells and microfilaments surrounding the endothelial fenestrations. The endothelial cells synthesize polyanionic glycosaminoglycans and glycoproteins, representing a glycocalyx, that coats the surfaces of the glomerular endothelial cells, providing a negative charge. The endothelial cell glycocalyx contributes to the charge-selective properties of the glomerular capillary wall and constitutes an important part of the filtration barrier.
The surfaces of glomerular endothelial cells express receptors for vascular endothelial growth factor (VEGF). VEGF is synthesized by the glomerular visceral epithelial cells and is an important regulator of microvascular permeability. VEGF increases endothelial cell permeability and induces the formation of endothelial fenestrations. Gene deletion studies in mice have demonstrated that VEGF is required for normal differentiation of glomerular endothelial cells. VEGF is also important for endothelial cell survival and repair in glomerular diseases characterized by endothelial cell damage. Thus, VEGF produced by the visceral epithelial cells plays a critical role in the differentiation and maintenance of glomerular endothelial cells and is an important regulator of endothelial cell permeability.
Glomerular Basement Membrane
The GBM is composed of a central dense layer, the lamina densa, and two thinner, more electron-lucent layers, the lamina rara externa and the lamina rara interna (see Figure 2.9 ). The latter two layers measure approximately 20 to 40 nm in thickness. The layered configuration of the GBM results in part from the fusion of endothelial and epithelial basement membranes during development. Although in the rat the width of the GBM has been found to be 132 nm, the width of the human GBM has consistently been reported to be more than 300 nm with a slightly thicker basement membrane in men (373 nm) than in women (326 nm). Like other basement membranes in the body, the GBM is composed primarily of collagen IV, laminin, entactin/nidogen, and sulfated proteoglycans. In addition, the GBM contains specific components, such as laminin 11, distinct collagen IV α chains, and the proteoglycans agrin and perlecan. As reviewed by Kashtan, six isomeric chains, designated α1 through α6 (IV), constitute the type IV collagen family of proteins. Of these six chains, α1 through α5 have been identified in the normal GBM. Mutations in the genes encoding α3, α4, and α5 (IV) chains are known to cause Alport’s syndrome, a hereditary basement membrane disorder associated with progressive glomerulopathy.
The exact contribution of the GBM to the glomerular filtration barrier remains somewhat controversial. Ultrastructural tracer studies provided evidence that the GBM constitutes both a size-selective and a charge-selective barrier. Caulfield and Farquhar demonstrated the existence of anionic sites in all three layers of the GBM. Additional studies revealed a lattice of anionic sites with a spacing of approximately 60 nm ( Figure 2.11 ) throughout the lamina rara interna and lamina rara externa. The anionic sites in the GBM consist of glycosaminoglycans rich in heparan sulfate. Removal of the heparan sulfate side chains by enzymatic digestion resulted in an increase in the in vitro permeability of the GBM to ferritin and to bovine serum albumin, suggesting that glycosaminoglycans might play a role in establishing the permeability properties of the GBM to plasma proteins (see Figure 2.11 ). However, in vivo digestion of heparan sulfates with heparanase in rats did not result in proteinuria. Furthermore, neither mice genetically engineered to lack agrin and perlecan heparin sulfate side chains, nor collagen XVIII–deficient mice, demonstrate significant proteinuria. Moreover, studies in the isolated GBM failed to demonstrate charge selectivity in vitro . Nevertheless, a variety of genetic findings in humans and knockout studies in mice suggest that the GBM significantly contributes to the functional properties of the glomerular filtration barrier. The strongest evidence for a specific role of the GBM in the filtration barrier is the finding that mice deficient in laminin β2, a major component of the GBM, have massive proteinuria, as do patients with mutations in this gene. Importantly, in the laminin β2 knockout mice, proteinuria is associated with increases in the permeability of the GBM that precede the onset of any abnormalities in the podocyte.

Visceral Epithelial Cells
The visceral epithelial cells, also called podocytes, are terminally differentiated cells that do not replicate anymore. The podocytes are the largest cells in the glomerulus (see Figure 2.6 ) and are characterized by long cytoplasmic processes that extend from the main cell body. The primary processes divide into secondary and tertiary processes and finally into the individual foot processes, or pedicels, that come into direct contact with the lamina rara externa of the GBM (see Figures 2.8 and 2.9 ). By scanning electron microscopy, it is apparent that adjacent foot processes are derived from different podocytes ( Figure 2.12 ). The podocytes contain well-developed Golgi complexes and are capable of endocytosis. Lysosomes are frequently observed, and their heterogeneous content most likely reflects the uptake of proteins and other components from the ultrafiltrate. Large numbers of microtubules, microfilaments, and intermediate filaments are present in the cytoplasm, and actin filaments are especially abundant in the foot processes. Despite a prominent motility in vitro, podocytes in the healthy glomerulus in vivo are rather stationary and maintain fixed positions of their cell bodies and microprojections as shown by intravital time-lapse microscopy of zebrafish larvae and mouse kidneys. However, podocyte motility drastically increases in the mice with glomerular damage induced by unilateral ureteral ligation and doxorubicin (Adriamycin) nephropathy.

In a healthy glomerulus, the distance between adjacent foot processes near the GBM varies from 25 to 60 nm (see Figure 2.9 ). This gap, referred to as the filtration slit or slit pore, is bridged by a thin membrane called the filtration slit membrane or slit diaphragm, which is located approximately 60 nm from the GBM. A continuous central filament with a diameter of approximately 11 nm can be seen in the filtration slit diaphragm. Detailed studies of the slit diaphragm in the rat, mouse, and human glomerulus have revealed that the 11 nm wide central filament is connected to the cell membrane of the adjacent foot processes by regularly spaced cross-bridges approximately 7 nm in diameter and 14 nm in length, giving the slit diaphragm a zipper-like configuration ( Figure 2.13 ). The slit diaphragm has the morphologic features of an adherens junction, and the tight junction protein ZO-1 has been localized to the sites where the slit diaphragm is connected to the plasma membrane of the foot processes.

The molecular components of the slit diaphragm and their role in determining the selective properties of the filtration barrier are now well established. The slit diaphragm is formed by a complex of the transmembrane proteins nephrin, NEPH1-3, podocin, Fat1, VE-cadherin and P-cadherin. Mutations in nephrin and podocin cause inherited nephrotic syndrome, and knockout of nephrin, NEPH1, and podocin causes proteinuria in mice. In addition, mutations in linker proteins that connect the slit diaphragm to the actin cytoskeleton, such as CD2AP and Nck, also cause proteinuria.
In many diseases associated with proteinuria, the interdigitating foot processes are replaced by rather broad adhesions of the cells to their GBM. This process is commonly referred to as foot process fusion or effacement . As reviewed by Kriz and associates, foot process effacement is a rather complex structural response that involves profound cytoskeletal rearrangements that may finally prevent detachment of the podocyte from the GBM after injury.
Mesangial Cells
The mesangial cells and their surrounding matrix constitute the mesangium, which is separated from the capillary lumen by the endothelium (see Figures 2.6 and 2.8 ). Light and electron microcopy studies have provided detailed descriptions of the mesangium. The mesangial cell is irregular in shape, with a dense nucleus and elongated cytoplasmic processes that can extend around the capillary lumen and insinuate themselves between the GBM and the overlying endothelium (see Figure 2.8 ). In addition to the usual complement of organelles, mesangial cells possess an extensive array of microfilaments composed at least in part of actin, α-actinin, and myosin. The contractile mesangial cell processes appear to bridge the gap in the GBM encircling the capillary, and bundles of microfilaments interconnect opposing parts of the GBM, an arrangement that is believed to prevent capillary wall distension secondary to elevation of the intracapillary hydraulic pressure.
The mesangial cell is surrounded by a matrix that is similar to but not identical with the GBM; the mesangial matrix is more coarsely fibrillar and slightly less electron dense. Several cell surface receptors of the β-integrin family have been identified on the mesangial cells, including α1β1, α3β1, and the fibronectin receptor, α5β1. These integrins mediate attachment of the mesangial cells to specific molecules in the extracellular mesangial matrix and link the matrix to the cytoskeleton. The attachment to the mesangial matrix is important for cell anchorage, contraction, and migration; ligand-integrin binding also serves as a signal transduction mechanism that regulates the production of extracellular matrix as well as the synthesis of various vasoactive mediators, growth factors, and cytokines.
As proposed by Schlondorff, the mesangial cell may have some specialized features of pericytes and possesses many of the functional properties of smooth muscle cells. In addition to providing structural support for the glomerular capillary loops, the mesangial cell has contractile properties and is thought to play a role in the regulation of glomerular filtration. The local generation of autacoids, such as prostaglandin E 2 (PGE 2 ), by the mesangial cell, may provide a counterregulatory mechanism to oppose the effect of vasoconstrictors.
Mesangial cells exhibit phagocytic properties and participate in the clearance of macro molecules from the mesangium, as evidenced by the uptake of tracers such as ferritin, colloidal carbon, and aggregated proteins. Mesangial cells are also involved in the generation and metabolism of the extracellular mesangial matrix. Because of both their distinct anatomic localization and their production of various vasoactive substances (e.g., nitric oxide [NO]), growth factors (e.g., VEGF, platelet-derived growth factor [PDGF], transforming growth factor [TGF]), and cytokines and chemokines (interleukins [ILs], chemokine [C-X-C motif] ligand 1 [CXCLs], chemokine [C-C motif] ligand [CCLs]), mesangial cells are also perfectly suited to mediate an extensive crosstalk to both endothelial cells and podocytes to control and maintain glomerular function. As such, the mesangial cells also importantly contribute to a number of glomerular diseases, including immunoglobulin A (IgA) glomerulonephritis and diabetic nephropathy.
Parietal Epithelial Cells
The parietal epithelium, which forms the outer wall of Bowman’s capsule, is continuous with the visceral epithelium at the vascular pole. The parietal epithelial cells are squamous in character, but at the urinary pole there is an abrupt transition to the taller cuboidal cells of the proximal tubule, which has a well-developed brush border ( Figures 2.14 and 2.15 ). The parietal epithelium of the capsule was described in detail by Jørgensen. The cells are 0.1 to 0.3 µm in height, except at the nucleus, where they increase to 2.0 to 3.5 µm. Each cell has a long cilium and occasional microvilli up to 600 nm in length. Cell organelles are generally sparse and include small mitochondria, numerous vesicles of 40 to 90 nm in diameter, and the Golgi apparatus. Large vacuoles and multivesicular bodies are rare. The thickness of the basement membrane of Bowman’s capsule varies from 1200 to 1500 nm. The basement membrane is composed of multiple layers, or lamellae, that increase in thickness with many disease processes. At both the vascular pole and the urinary pole, the thickness of Bowman’s capsule decreases markedly.


The parietal epithelial cell functions as the final permeability barrier for the urinary filtrate. In experimental glomerulonephritis, this barrier is compromised, and macromolecules can leak into the space between the parietal cell and the basement membrane of Bowman’s capsule and subsequently into the periglomerular space. There is also evidence that the parietal epithelial cell can transdifferentiate into podocytes and can even repopulate the glomerular tuft after extensive podocyte loss. However, as reviewed by Shankland and associates, abberant proliferation of parietal epithelial cells may also contribute to renal scarring or the formation of glomerular crescents in certain renal disease processes, such as rapidly progressive glomerulonephritis.
Peripolar Cells
Ryan and colleagues have described a peripolar cell that is located at the origin of the glomerular tuft in Bowman’s space and interposed between the visceral and parietal epithelial cells. The peripolar cells are especially prominent in sheep, but they have also been identified in other species, including humans, and have been localized predominantly in glomeruli in the outer cortex. The functional significance of these peripolar cells is unclear.
Juxtaglomerular Apparatus
The juxtaglomerular apparatus is located at the vascular pole of the glomerulus, where a portion of the distal nephron comes into contact with its parent glomerulus. It represents a major structural component of the renin-angiotensin system and contributes to the regulation of glomerular arteriolar resistance and glomerular filtration. The juxtaglomerular apparatus has a vascular component and a tubular component. The vascular component is composed of the terminal portion of the afferent arteriole, the initial portion of the efferent arteriole, and the extraglomerular mesangial region. The tubular component is the macula densa, which is the terminal portion of the TAL and which is in contact with the vascular component. The extraglomerular mesangial region, which has also been referred to as the polar cushion (polkissen) or the lacis, is bounded by the cells of the macula densa, the specialized regions of the afferent and efferent glomerular arterioles, and the mesangial cells of the glomerular tuft (the intraglomerular mesangial cells). Within the vascular component of the juxtaglomerular apparatus, two distinct cell types can be distinguished: the juxtaglomerular granular cells, also called epithelioid or myoepithelial cells, and the agranular extraglomerular mesangial cells, which are also referred to as lacis cells.
Juxtaglomerular Granular Cells
The granular cells are located primarily in the walls of the afferent and, to a lesser extent, the efferent arterioles. They exhibit features of both smooth muscle cells and secretory epithelial cells and therefore have been called myoepithelial cells . They contain myofilaments in the cytoplasm and, except for the presence of granules, are indistinguishable from the neighboring arteriolar smooth muscle cells. They also exhibit features suggestive of secretory activity, including a well-developed endoplasmic reticulum and a Golgi complex containing small granules with a crystalline substructure.
The juxtaglomerular granular cells are characterized by the presence of numerous membrane-bound granules of variable size and shape ( Figure 2.16 ), which are thought to contain the aspartic protease rennin. In addition to these so-called specific granules, lipofuscin-like granules are commonly observed in the human kidney.

The juxtaglomerular granular cells express both renin and angiotensin II, with activities being highest in the afferent arteriole. Immunogold electron microscopy revealed that renin and angiotensin II colocalize in the same granules. Enzyme histochemical and immunocytochemical studies also demonstrated the presence of lysosomal enzymes, including acid phosphatase and cathepsin B, in renin-containing granules, suggesting that these granules may represent modified lysosomes. During renal development, a widespread expression of renin in the developing intrarenal arteries is seen. It later disappears from the larger arteries and arterioles and becomes restricted to the granular cells in the end portion of the afferent arteriole. However, in response to extracellular volume depletion, renin expression may again extend into more proximal arterial portions, suggesting that the renal arterial smooth muscle cells retain their ability to produce renin and can be recruited for renin secretion, depending on functional demand.
Extraglomerular Mesangium
Located between the afferent and efferent arterioles in close contact with the macula densa (see Figure 2.16 ), the extraglomerular mesangium is continuous with the intraglomerular mesangium and is composed of cells that are similar in ultrastructure to the mesangial cells. The extraglomerular mesangial cells possess long, thin cytoplasmic processes that are separated by basement membrane material. Under normal conditions, these cells do not contain granules; however, juxtaglomerular granular cells are occasionally observed in the extraglomerular mesangium. The extraglomerular mesangial cells are in contact with the arterioles and the macula densa, and gap junctions are commonly observed between the various cells of the vascular portion of the juxtaglomerular apparatus. Gap junctions have also been described between extraglomerular and intraglomerular mesangial cells, suggesting that the extraglomerular mesangium may serve as a functional link between the macula densa and the glomerular arterioles. Moreover, there is evidence that mesangial cell damage and selective disruption of gap junctions eliminate the tubuloglomerular feedback response.
Macula Densa
The macula densa is a specialized region of the distal tubule adjacent to the hilum of the parent glomerulus (see Figure 2.16 ). Only those cells immediately adjacent to the hilum are morphologically distinctive from the surrounding cells of the TAL and are composed of columnar cells with apically placed nuclei. With electron microscopy, the cell base is seen to interdigitate with the adjacent extraglomerular mesangial cells. Although mitochondria are numerous, their orientation is not perpendicular to the base of the cell, and they are rarely enclosed within foldings of the basolateral plasma membrane. The position of the Golgi apparatus is lateral to and beneath the cell nucleus. In addition, other cell organelles, including lysosomes, autophagic vacuoles, ribosomes, and profiles of smooth and granular endoplasmic reticulum, are located principally beneath the cell nucleus. The basement membrane of the macula densa is continuous with that surrounding the granular and agranular cells of the extraglomerular mesangial region, which in turn is continuous with the matrix material surrounding the mesangial cells within the glomerular tuft. Macula densa cells lack the lateral cell processes and interdigitations that are characteristic of the TAL. Ultrastructural studies have provided evidence that the width of the lateral intercellular spaces in the macula densa varies with the physiologic status of the animal.
Autonomic Innervation
The function of the juxtaglomerular apparatus is controlled by the autonomic nervous system. Electron microscopy demonstrated synapses between granular and agranular cells of the juxtaglomerular apparatus and autonomic nerve endings. Nerve endings, principally adrenergic in type, were observed to be in contact with approximately one third of the cells of the efferent arteriole and with somewhat less than one third of the cells of the afferent arteriole, while the frequency of innervation of the tubule component of the juxtaglomerular apparatus was far less. Consistent with the existence of neuroeffector junctions on renin-positive granular cells of the juxtaglomerular apparatus, renin secretion was shown to be modulated by renal sympathetic nerve activity.
Tubuloglomerular Feedback
The cells of the macula densa sense changes in the luminal concentrations of sodium and chloride, presumably via absorption of these ions across the luminal membrane by the Na + -K + -2Cl − cotransporter, of which the NKCC2B and NKCC2A variants are expressed in the macula densa. This sensing initiates the tubuloglomerular feedback response (see Chapter 3 ) by which signals generated by acute changes in sodium chloride concentration are transferred via the macula densa cells to the glomerular arterioles to control the glomerular filtration rate. Signals from the macula densa, generated in response to changes in luminal sodium and chloride, are also transmitted to the renin-secreting cells in the afferent arteriole. Renin synthesis and secretion by the juxtaglomerular granular cells are also controlled by several other factors, including neurotransmitters of the sympathetic nervous system, glomerular perfusion pressure (presumably through arteriolar baroreceptors), and mediators in the macula densa. There is increasing evidence that the macula densa control of renin secretion is mediated by NO, cyclo-oxygenase products such as PGE 2 , and adenosine. In addition to its inhibition of renin secretion, adenosine appears to serve as a mediator of the tubuloglomerular feedback response evoked by an increased NaCl concentration at the macula densa.
Proximal Tubule
The proximal tubule consists of an initial convoluted portion, the pars convoluta, which is a direct continuation of the parietal epithelium of Bowman’s capsule, and a straight portion, the pars recta, which is located in the medullary ray (see Figure 2.5 ). The length of the proximal tubule varies with species; for instance, it is 10 mm in rabbits, 8 mm in rats, 4 to 5 mm in mice, and 14 mm in humans.
In the rat and the rhesus monkey, three morphologically distinct segments—S1, S2, and S3—have been identified. The S1 segment is the initial portion of the proximal tubule; it begins at the glomerulus and constitutes approximately two thirds of the pars convoluta (see Figures 2.14 and 2.15 ). The S2 segment contains the final third of the pars convoluta and the initial portion of the pars recta. The S3 segment is the remainder of the proximal tubule, located in the deep inner cortex and the outer stripe of the outer medulla. These segments can be distinguished morphologically because of their structurally unique cells ( Figures 2.17 through 2.19 ). Cells in the S1 segment have a tall brush border and a well-developed vacuolar-lysosomal system. The basolateral plasma membrane forms extensive lateral invaginations, and lateral cell processes extending from the apical to the basal surface interdigitate with similar processes from adjacent cells. Elongated mitochondria are located in the lateral cell processes in proximity to the plasma membrane. The ultrastructure of cells in the S2 segment is similar to that in the S1 segment; however, the brush border is shorter, the basolateral invaginations are less prominent, and the mitochondria are smaller. Numerous small lateral processes are located close to the base of the cell. The endocytic compartment is less prominent than in the S1 segment, with the number and size of the lysosomes varying among species and between males and females.



In cells from the S3 segment, the length of the brush border differs with species and can be rather short (in humans) or long (in rats). Lateral cell processes and invaginations are essentially absent, and mitochondria are small and randomly distributed within the cell. Species variation is also observed in the vacuolar-lysosomal compartment in the S3 segment. In rats and humans, endocytic vacuoles and lysosomes are small and sparse, whereas in rabbits, large endocytic vacuoles and numerous small lysosomes are present. Peroxisomes are present throughout the proximal tubule, with progressively increased quantities toward the S3 segment. There are contrasts to the described morphology in different species. In rabbits, the S2 segment represents a transition between the S1 and S3 segments, whereas in mice there is no structural segmentation along the proximal tubule. In the nondiseased human kidney, only the pars convoluta and the pars recta have been positively identified and described. To facilitate comparisons, the remainder of this chapter differentiates the proximal tubule into the convoluted and the straight portions, rather than the S1, S2, and S3 segments.
Pars Convoluta
Cells of the pars convoluta are structurally complex ( Figure 2.20 ). Large primary ridges extend laterally from the apical to the basal surfaces of the cells. Large lateral processes, often containing mitochondria, extend outwards from the primary ridges and interdigitate with similar processes in adjacent cells (see Figure 2.20 ). At the luminal surfaces of the cells, smaller lateral processes extend outwards from the primary ridges to interdigitate with those of adjacent cells. Small basal villi that do not contain mitochondria are found along the basal cell surfaces ( Figure 2.21 ). These extensive interdigitations result in a complex extracellular compartment, referred to as the basolateral intercellular space (see Figures 2.21 and 2.22 ), that is separated from the tubule lumen (apical cell surface) by the zonula occludens or tight junctions . In parallel with a high-resistance pathway across the apical and basal plasma membranes of the proximal tubule cell, a low-resistance shunt pathway is also present. Thus, the proximal tubule is often referred to as “a leaky epithelium” with low transepithelial resistance and high paracellular transport. Claudins, a diverse family of tight junction proteins with various ion permeability properties that are expressed throughout the renal tubule system, mediate the paracellular permeability properties of the tight junction (see Chapter 6 ). Claudin-2 and claudin-10 are highly expressed in proximal tubule cells, and claudin-2 forms high-conductance cation pores permitting large amounts of paracellular Na + transport. Interestingly, claudin-2, which is frequently expressed in leaky epithelia, has been shown to be water permeable. Below the tight junction lies the beltlike intermediate junction or zonula adherens, which is followed by several desmosomes distributed randomly at variable distances beneath the intermediate junction. In mammalian and invertebrate renal proximal tubules, gap junctions are present in small numbers and can provide a pathway for the movement of ions between cells and for cell-cell communication via a family of proteins known as connexins . The lateral intercellular space of each pars convoluta cell is open toward the basement membrane, which separates the cell from the peritubular interstitium and capillaries. The thickness of the basement membrane gradually decreases along the proximal tubule, in the rhesus monkey, for example, from approximately 250 nm in the S1 segment to 145 nm in the S2 segment to 70 nm in the S3 segment.



The lateral cell processes of pars convoluta cells combined with extensive invaginations of the plasma membrane increase both the intercellular space and surface area of the basolateral plasma membrane. Studies in rabbits have demonstrated that the area of the lateral surface equals that of the luminal surface and amounts to 2.9 mm 2 per mm of tubule. Elongated mitochondria are located in the lateral cell processes in close proximity to the plasma membrane (see Figure 2.22 ), where the sodium-potassium adenosine triphosphatase (Na + -K + -ATPase) is located. Mitochondria are often observed as rod-shaped and tortuous, but many mitochondria are branched and connected with one another. A system of smooth membranes called the paramembranous cisternal system, thought to be in continuity with the smooth endoplasmic reticulum, is often observed between the plasma membrane and mitochondria. The function of the paramembranous cisternal system is not known. Pars convoluta cells contain large quantities of smooth and rough endoplasmic reticulum, and free ribosomes are abundant in the cytoplasm. A well-developed Golgi apparatus, composed of smooth-surfaced sacs or cisternae, coated vesicles, uncoated vesicles, and larger vacuoles, is located above and lateral to the nucleus in the midregion of the cell ( Figure 2.23 ). In addition, an extensive system of microtubules is located throughout the cytoplasm of proximal tubule cells.

Pars convoluta cells have well-developed brush borders at their luminal surfaces that are formed by numerous finger-like projections of the apical plasma membranes, the microvilli . The brush border serves to increase the apical cell surface, by 36-fold in rabbit kidneys. Each microvilli contains 6 to 10 actin filaments of approximately 6 nm in diameter that extend downwards into the apical region of the cell for variable distances. A network of filaments containing myosin and spectrin, called the terminal web, is located in the apical cytoplasm just beneath and perpendicular to the microvilli. Each pars convoluta cell has a well-developed endocytic-lysosomal apparatus that is involved in the reabsorption and degradation of macromolecules from the ultrafiltrate. The endocytic compartment consists of an extensive system of coated pits, small coated vesicles, apical dense tubules, and larger endocytic vacuoles without a cytoplasmic coat ( Figure 2.24 ). The coated pits are invaginations of the apical plasma membrane at the base of the microvilli and contain clathrin and megalin, proteins that are involved in receptor-mediated endocytosis. The cytoplasmic coat of the small vesicles is similar in ultrastructure to the coat that is present on the cytoplasmic side of the coated pits.

A large number of lysosomes with variable size, shape, and ultrastructural appearance are present in cells of the pars convoluta ( Figure 2.25 ). Lysosomes are membrane-bound, heterogeneous organelles that contain a variety of acid hydrolases, including acid phosphatases, and various proteases, lipases, and glycosidases. Lysosomes are involved in the degradation of material absorbed by endocytosis (heterophagocytosis), and they often contain multiple electron-dense deposits that are believed to represent reabsorbed substances such as proteins (see Figure 2.25 ). Lysosomes also play a role in the normal turnover of intracellular constituents by autophagocytosis, and autophagic vacuoles containing fragments of cell organelles are often seen in pars convoluta cells. Lysosomes containing nondigestible substances are called residual bodies, which can empty their contents into the tubule lumen by exocytosis. Multivesicular bodies (MVBs), which are part of the vacuolar-lysosomal system, are often observed in the cytoplasm of proximal convoluted tubule cells. MVBs were originally thought to be involved in membrane retrieval and/or membrane disposal, but later studies suggest that MVBs may provide an exit route for a variety of endocytically retrieved plasma membrane proteins (of both basolateral and apical membrane origin) and could also function as a signaling mechanism to downstream nephron segments. The extensive vacuolar-lysosomal system of proximal tubule cells plays an important role in the reabsorption and degradation of albumin and low-molecular-weight plasma proteins from the glomerular filtrate. Under normal conditions the vacuolar-lysosomal system is most prominent in the pars convoluta, but in proteinuric states large vacuoles and extensive lysosomes can be observed in the latter portions of the proximal tubule.

Pars Recta
The pars recta is the terminal portion of the S2 segment and the entire S3 segment. Pars recta morphology varies considerably between species, (e.g., in the rat, the microvilli of the brush border measure up to 4 µm in length, whereas in the rabbit and human kidney they are much shorter). The epithelium of the pars recta S3 segment is simpler than that of the S1 and S2 segments. Invaginations of the basolateral plasma membrane are virtually absent, mitochondria are small and randomly scattered throughout the cytoplasm, and the intercellular spaces are smaller and less complex (see Figures 2.21 and 2.26 ). These morphologic characteristics are in agreement with studies demonstrating that Na + -K + -ATPase activity and fluid reabsorption are significantly less in the pars recta than in the pars convoluta. In contrast to cells of the pars convoluta, the vacuolar-lysosomal system is less prominent in cells of the S3 segment, although in both rabbits and humans, many small lysosomes with electron-dense membrane-like material can still be observed. Peroxisomes are common in the pars recta. In contrast to lysosomes, peroxisomes are irregular in shape, are surrounded by a 6.5-nm-thick membrane, and do not contain acid hydrolases. Peroxisomes within the pars recta vary considerably in appearance among species. In the rat, small, circular profiles can be observed just inside the limiting membrane, and rod-shaped structures often project outward from the organelle. In addition, a small nucleoid is often present in peroxisomes from the pars recta. The functional significance of peroxisomes in the kidney is not known with certainty; however, they are believed to be involved in lipid metabolism and fatty acid oxidation. They have a high content of catalase, which is involved in the degradation of hydrogen peroxide, and of various oxidative enzymes, including l-α-hydroxy-acid oxidase and d -amino acid oxidase. Interestingly, mistargeting of the mutated peroxisomal enzyme EHHADH (enoyl-CoA hydratase– l -3-hydroxyacyl-CoA dehydrogenase) to proximal tubule mitochondria disrupts mitochondrial metabolism and leads to renal Fanconi’s syndrome.

The proximal tubule plays a major role in the reabsorption of Na + , HCO 3 − , Cl − , K + , Ca 2+ , PO 4 3− , water, and organic solutes such as vitamins, glucose, and amino acids. The aforementioned ultrastructural specializations of the proximal tubule cells aid in these transport processes. The transcellular transport of these substances occurs via specific transport proteins that have polarized expression in proximal tubule cells (see Chapter 6 , Chapter 7 , Chapter 8 , Chapter 9 ). Of note, the rate of fluid absorption from the proximal tubule to the peritubular capillaries is influenced by the hydraulic and oncotic pressures across the tubule and capillary wall. Changes in these parameters cause significant ultrastructural changes in the proximal tubule, especially in the configuration of the lateral intercellular spaces.
Thin Limbs of the Loop of Henle
For an extensive review of the structure and function of the thin limbs of the loop of Henle, see the review by Pannabecker. The thin limbs of the loop of Henle connect the proximal and distal tubules of the nephron. The transition from the proximal tubule to the descending thin limb of the loop of Henle is abrupt ( Figures 2.27 and 2.28 ) and marks the boundary between the outer and inner stripes of the outer medulla. Short-looped nephrons originating from superficial and midcortical glomeruli have a short descending thin limb located in the inner stripe of the outer medulla. Close to the hairpin turn of the short loops of Henle, the thin limb continues into the TAL. Long-looped nephrons originating from juxtamedullary glomeruli have a long descending thin limb that extends into the inner medulla and a long ascending thin limb that continues into the TAL. The transition from the thin to the thick ascending limb forms the boundary between the outer and inner medulla (see Figure 2.5 ). Nephrons arising in the extreme outer cortex may possess short cortical loops that do not extend into the medulla. Variations on this basic organization have been highlighted in studies of different species. The histotopographic organization of the renal medulla has been studied in several laboratory animals, including three-dimensional (3D) reconstruction studies of the nephron. These studies have highlighted the complexity of organization of the medulla and are discussed in detail in Chapter 10 .


Ultrastructural studies have shown that the cells of the initial part of the descending thin limb of Henle have extensive interdigitation with one another, whereas the cells of the ascending thin limb near the transition with the TAL, and thin limb cells in the inner medulla, are less complex in configuration. Four morphologically distinct segments of the thin limb of Henle, composed of four types of epithelia (types I through IV), have been described and classified on the basis of their ultrastructure and location within the medulla ( Figure 2.29 ). Type I epithelium is found exclusively in the descending thin limb of short-looped nephrons. Type II epithelium forms the descending thin limb of long-looped nephrons in the outer medulla and gives way to type III epithelium in the inner medulla. Type IV epithelium forms the bends of the long loops and the entire ascending thin limb to the transition into the TAL at the boundary between the inner and outer medulla. Type I epithelium is extremely thin and has few basal or luminal surface specializations, the latter in the form of microvilli (see Figure 2.29 ). There is a virtual absence of lateral interdigitations with adjacent cells, and cellular organelles are relatively sparse. Tight junctions between cells are intermediate in depth with several junctional strands, suggesting a tight epithelium. Type II epithelium is taller and exhibits considerable species differences. In the rat, mouse, Psammomys obesus, and hamster, the type II epithelium is complex and characterized by extensive lateral and basal interdigitations and a well-developed paracellular pathway ( Figure 2.30 ). The tight junctions are extremely shallow and contain a single junctional strand, characteristics of a “leaky epithelium.” The luminal surface is covered by short blunt microvilli, and cell organelles, including mitochondria, are more prominent than in other segments of the thin limb. In the rabbit the type II epithelium is less complex ; lateral interdigitations and paracellular pathways are less prominent, and tight junctions are deeper. In comparison with type II epithelium, type III epithelium is lower (thinner) and has a simpler structure. The cells do not interdigitate, the tight junctions are intermediate in depth, and fewer microvilli cover the luminal surface. Type IV epithelium (see Figure 2.29 ) is generally low and flattened and possesses relatively few organelles. It is characterized by an absence of surface microvilli but has an abundance of lateral cell processes and interdigitations. The tight junctions are shallow, characteristic of a leaky epithelium, and as such type IV epithelium has prominent paracellular pathways.


The basement membrane of the thin limb segments varies greatly in thickness from species to species and in many animals is multilayered. There is structural heterogeneity along the thin limb of the loop of Henle in the rat, rabbit, and P. obesus . Segmental as well as species differences were found in the number of strands and the depth of the tight junctions. The most striking finding in these ultrastructural studies was the extremely high density of intramembrane particles in both the luminal and the basolateral membranes of type II epithelium. In the rat, type II epithelium has significant levels of Na + -K + -ATPase activity, whereas little activity is detectable in other segments of the rat thin limb or in any segment of the rabbit thin limb. Permeability studies of isolated perfused descending thin limbs from different species have demonstrated that type II epithelium has a higher permeability to Na + and K + in the rat and hamster than in the rabbit, supporting the described differences in ultrastructure and biochemical properties of type II epithelium among species.
It is generally accepted that the permeability properties of the thin limb epithelium are important for the maintenance of a hypertonic interstitium. The role of the thin limb in the maintenance of a hypertonic medullary interstitium and in the dilution and concentration of the urine via countercurrent multiplication is discussed in detail in Chapter 10 .
Distal Tubule
The distal tubule is composed of morphologically distinct segments: the TAL (pars recta), the macula densa, the distal convoluted tubule (DCT) (pars convoluta), and the connecting tubules. Some studies showed that the cortical TAL extends beyond the vicinity of the macula densa and forms an abrupt transition with the DCT at a slightly more distal position. These data, combined with the observation that TAL proteins are observed by immunohistochemical methods even slightly distal to the macula densa, suggest that the macula densa is a specialized region within the TAL.
Thick Ascending Limb
The TAL represents the initial portion of the distal tubule and can be divided into a medullary and a cortical segment (see Figure 2.5 ). In long-looped nephrons, there is an abrupt transition from the thin ascending limb to the TAL, which marks the boundary between the inner medulla and the inner stripe of the outer medulla. In short-looped nephrons, the transition to the TAL can occur shortly before the hairpin turn, but this is not the case in all species. From its transition from the thin limb, the TAL extends upward through the outer medulla and the cortex to the glomerulus belonging to the nephron of origin, where the macula densa is formed. At the point of contact with the extraglomerular mesangial region, only the immediately contiguous portion of the wall of the tubule actually forms the macula densa. The transition from TAL to the DCT occurs shortly after the macula densa. The cells forming the medullary segment in the inner stripe of the outer medulla measure approximately 7 to 8 µm in height. As the tubule ascends toward the cortex, cell height gradually decreases to approximately 5 µm in the cortical TAL of the rat and to 2 µm in the terminal part of the cortical TAL of the rabbit. Welling and colleagues reported an average cell height of 4.5 µm in the cortical TAL of the rabbit kidney.
The cells of the TAL are characterized by extensive invaginations of the basolateral plasma membrane and interdigitations between adjacent cells. The lateral invaginations often extend two thirds or more of the distance from the base to the luminal border of the cell. This arrangement is most prominent in the TAL of the inner stripe of the outer medulla ( Figure 2.31 ). Numerous elongated mitochondria are located in lateral cell processes, and their orientation is perpendicular to the basement membrane. The mitochondria resemble those in the proximal tubule but contain very prominent granules in the matrix. Other subcellular organelles in this segment of the nephron include a well-developed Golgi complex, multivesicular bodies and lysosomes, and abundant quantities of smooth and rough endoplasmic reticulum. Numerous small vesicles are commonly observed in the apical portion of the cytoplasm. The cells are attached to one another via tight junctions that are 0.1 to 0.2 µm in depth in the rat. Intermediate junctions are also present, but desmosomes appear to be lacking.

Scanning electron microscopy of the TAL of the rat kidney has revealed the existence of two distinct surface configurations of the luminal membrane. Some cells have a rough surface because of the presence of numerous small microprojections, whereas others have a smooth surface that is largely devoid of microprojections except along the apical cell margins ( Figure 2.32 ). Like all other cells from the parietal layer of Bowman’s capsule to the terminal collecting duct (except intercalated cells), TAL cells possess a primary cilium. The rough-surfaced cells possess more extensive lateral processes radiating from the main cell body than do the smooth-surfaced cells. In contrast, small vesicles and tubulovesicular profiles are more numerous in the apical region of the smooth-surfaced cells. A predominance of cells with the smooth-surface pattern is observed in the medullary segment. As the thick limb ascends toward the cortex, the number of cells with a rough surface pattern increases, and luminal microprojections and apical lateral invaginations become more prominent. Consequently, the surface area of the luminal plasma membrane is significantly greater in the cortical TAL than in the medullary TAL.

The TAL is involved in active transport of NaCl from the lumen to the surrounding interstitium. Because this epithelium is almost impermeable to water, the reabsorption of salt contributes to the formation of a hypertonic medullary interstitium and the delivery of a dilute tubule fluid to the DCT (see Chapter 6 ). In studies by Good and colleagues evidence is provided that in addition to NaCl reabsorption, the TAL is involved in HCO 3 − reabsorption in the rat. Finally, the TAL is involved in the transport of divalent cations such as Ca 2+ and Mg 2+ . The reabsorption of NaCl in both the medullary and the cortical segments of the TAL is mediated by a Na + -K + -2Cl − cotransport mechanism, which is inhibited by loop diuretics such as furosemide and bumetanide. The bumetanide-sensitive Na + -K + -2Cl − cotransporter (BSC-1 or NKCC2) is expressed in the cortical and medullary TAL, where it localizes to the apical plasma membrane domains.
The energy for reabsorptive processes in the TAL is provided by the Na + -K + -ATPase that is located in the basolateral plasma membrane. Biochemical and histochemical studies have demonstrated that Na + -K + -ATPase activity is greatest in the segment of the TAL from the inner stripe of the outer medulla, which also has a larger basolateral membrane area and more mitochondria than does the remainder of the TAL. In agreement with these observations, physiologic studies using the isolated perfused tubule technique have demonstrated that NaCl transport is greater in the medullary segment than in the cortical segment of the TAL. However, the cortical segment can create a steeper concentration gradient and can therefore achieve a lower NaCl concentration and a lower osmolality in the tubule fluid. Thus, an excellent correlation exists between the structural and functional properties of the TAL.
Distal Convoluted Tubule
The DCT measures approximately 1 mm in length and extends to the connecting tubule, which connects the nephron with the collecting duct. The cells of the DCT resemble those of the TAL but are considerably taller ( Figure 2.33 ). By light microscopy, the cells appear tall and cuboid, and they contain numerous mitochondria. The cell nuclei occupy an apical position just beneath the luminal plasma membrane. Scanning electron microscopy has demonstrated that the luminal surface of the DCT differs substantially from that of the TAL ( Figure 2.34 ; compare with Figure 2.32 ). The DCT is covered with numerous small microprojections or microplicae. The individual cells each possess one centrally placed primary cilium on the apical surface. The epithelium of the DCT is characterized by extensive invaginations of the basolateral plasma membrane and by interdigitations between adjacent cells similar to the arrangement in the TAL. Transmission electron microscopy reveals numerous elongated mitochondria that are located in lateral cell processes and are closely aligned with the plasma membrane. They are oriented perpendicular to the basement membrane and often extend from the basal to the apical cell surface ( Figure 2.35 ). The junctional complex in this segment of the nephron is composed of a tight junction, which is approximately 0.3 µm in depth and an intermediate junction. Lysosomes and multivesicular bodies are observed but are certainly less common than in the proximal tubule. The Golgi complex is well developed, and its location is lateral to the cell nucleus. The cells contain numerous microtubules and abundant quantities of rough- and smooth-surfaced endoplasmic reticulum and free ribosomes. Numerous small vesicles are located in the apical region of the cells. Investigators working with micropuncture techniques arbitrarily defined the distal tubule as the region of the nephron that begins just after the macula densa and extends to the first junction with another renal tubule. With that definition, however, the distal tubule can be formed by as many as four different types of epithelia. In general, the “early” distal tubule corresponds largely to the DCT and, in some species, the short segment of the TAL that extends beyond the macula densa, whereas the “late” distal tubule actually represents the connecting tubule and the first portion of the collecting duct, which is sometimes referred to as the initial collecting tubule ( Figure 2.36 ). (A more detailed discussion of the anatomy of this region of the renal tubule can be found in the next section, which describes the connecting tubule.)
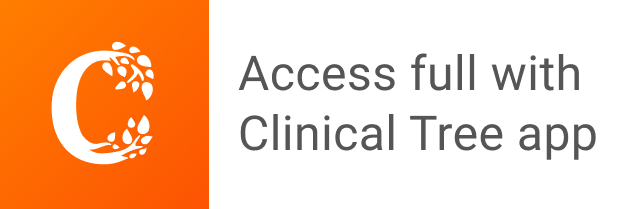