Chapter Outline
PHYSIOLOGY, 390
Sodium Balance, 391
Effective Arterial Blood Volume, 393
Regulation of Effective Arterial Blood Volume, 394
SODIUM BALANCE DISORDERS, 421
SPECIFIC TREATMENTS BASED ON THE PATHOPHYSIOLOGY OF CONGESTIVE HEART FAILURE, 451
Inhibition of the Renin-Angiotensin-Aldosterone System, 451
β-Blockade, 452
Nitric Oxide Donor and Reactive Oxygen Species/Peroxynitrite Scavengers, 452
Endothelin Antagonists, 452
Natriuretic Peptides, 453
Neutral Endopeptidase Inhibitors and Vasopeptidase Inhibitors, 453
Vasopressin Receptor Antagonists, 454
SPECIFIC TREATMENTS BASED ON THE PATHOPHYSIOLOGY OF SODIUM RETENTION IN CIRRHOSIS, 455
Pharmacologic Treatment, 455
Transjugular Intrahepatic Portosystemic Shunt, 457
Renal Replacement Therapy, 457
Liver Transplantation, 458
Sodium (Na + ) and water balance and their distribution among the various body compartments are essential for the maintenance of fluid homeostasis, particularly intravascular volume. Disturbances of either or both of these components have serious medical consequences, are relatively frequent, and are among the most common conditions encountered in hospital clinical practice. In fact, abnormalities of Na + and water balance are responsible for, or associated with, a wide spectrum of medical and surgical admissions or complications. The principal disorders of Na + balance are manifested clinically as hypovolemia or hypervolemia, whereas disruption in water balance can be diagnosed only in the laboratory as hyponatremia or hypernatremia. Although disorders of Na + and water balance are often interrelated, the latter are considered in a separate chapter. In this chapter, the physiologic and pathophysiologic features of Na + balance are discussed. Because Na + is restricted predominantly to the extracellular compartment, this chapter also addresses perturbations of extracellular fluid (ECF) volume homeostasis.
Physiology
Approximately 60% of adult body mass is composed of solute-containing fluids that can be divided into extracellular and intracellular compartments. Because water flows freely across cell membranes in accordance with the prevailing osmotic forces on either side of the membrane, the solute/water ratios in the intracellular fluid (ICF) and ECF are almost equal. However, the solute compositions of the ICF and ECF are quite different, as shown in Figure 15.1 . The principal ECF cation is sodium; minor cations are potassium (K + ), calcium, and magnesium. In contrast, potassium is the major ICF cation. The accompanying anions in the ECF are chloride, bicarbonate, and plasma proteins (mainly albumin), whereas electroneutrality of the ICF is maintained by phosphate and the negative charges on organic molecules. The difference in cationic composition of the two compartments is maintained by a pump leak mechanism consisting of sodium-potassium adenosine triphosphatase (Na + -K + -ATPase), which operates in concert with sodium and potassium conductance pathways in the cell membrane.

The free movement of water across the membrane ensures that the ECF and ICF osmolalities are the same. However, the intracellular volume is greater because the amount of potassium salts inside the cell is larger than that of sodium salts outside the cell. The movement of water is determined by the “effective osmolality,” or tonicity, of each compartment, so that if tonicity of the ECF rises—for example, as a result of excess Na + —water will move from the ICF to ECF to restore tonicity. On the other hand, addition of solute-free water leads to a proportionate decrease in both osmolality and tonicity of all body fluid compartments (see Chapter 16 for a detailed discussion). The restriction of Na + to the ECF compartment by the pump leak mechanism, in combination with maintenance of the osmotic equilibrium between ECF and ICF, ensures that ECF volume is determined mainly by total body Na + content.
The same mechanisms also govern the partitioning of fluid between the two compartments and are crucial for the preservation of near constancy of ECF and ICF volume in the presence of variations in dietary intake and extrarenal losses of Na + and water. To maintain constancy of the ECF and ICF and thereby safeguard hemodynamic stability, cell volume, and solute composition, even minute changes in these parameters can be detected by a number of sensing mechanisms. These sensory signals lead to activation of neural and hormonal factors, which, in turn, cause appropriate adjustments in urinary Na + and water excretion and, hence, restoration of fluid balance ( Figure 15.2 ). Constancy of ECF volume ensures a high degree of circulatory stability, whereas constancy of ICF volume protects against significant brain cell swelling or shrinkage.

Sodium Balance
Na + balance is the difference between intake (diet or supplementary fluids) and output (renal, gastrointestinal, perspiratory, and respiratory). In healthy humans in steady state, dietary intake is closely matched by urinary output of Na + . Thus, a person consuming a chronically low-Na + diet (20 mmol/day, or ≈1.2 g/day) excretes, in the steady state, a similar quantity of Na + in the urine (minus extrarenal losses). Conversely, on a high-Na + diet (200 mmol/day, or 12 g/day), approximately 200 mmol of Na + is excreted in the urine. Any perturbation of this balance leads to activation of the sensory and effector mechanisms outlined in the following discussions. In practice, any deviation in ECF volume in relation to its capacitance is sensed and translated, under the influence of neural and hormonal factors, into the appropriate change in Na + excretion, principally through the kidneys but also, to a much lesser degree, through stool and sweat.
According to the traditional two-compartmental model, body sodium balance and partitioning of extracellular fluid volume (ECFV) is based solely on exchangeable and osmotically active Na + . For normal functioning of the afferent sensing and efferent effector mechanisms that regulate ECF volume, the integrity of the intravascular and extravascular subcompartments of the ECF is crucial (see Figure 15.1 ). Although the composition and concentration of small, noncolloid electrolyte solutes in these two subcompartments are approximately equal (slight differences are due to the Gibbs-Donnan effect), the concentration of colloid osmotic particles (mainly albumin and globulin) is higher in the intravascular compartment. The balance between transcapillary hydraulic and colloid osmotic (oncotic) gradients (Starling forces) favors the net transudation of fluid from the intravascular to interstitial compartment. However, this is countered by movement of lymphatic fluid from the interstitial to intravascular compartment via the thoracic duct. The net effect is to restore and maintain the intravascular subcompartment at 25% of the total ECF volume (corresponding to 3.5 L of plasma); the remaining 75% is contained in the interstitial space (equivalent to 10.5 L in a 70-kg man; see Figure 15.2 ). The constancy of ECF volume and the appropriate partitioning of the fluid between intravascular and interstitial subcompartments are crucial for maintaining hemodynamic stability. In particular, intravascular volume in relation to overall vascular capacitance is a major determinant of left ventricular filling volume and, hence, cardiac output and mean arterial pressure.
The traditional two-compartment model of volume regulation, according to which the intravascular and interstitial spaces are in equilibrium, has been recently challenged. It now appears that Na + can be bound to and stored on proteoglycans in interstitial sites, where it becomes osmotically inactive; accordingly, a novel mechanism of volume regulation has been elucidated. In rats fed a high-salt diet, this uniquely bound Na + was found to induce a state of subcutaneous interstitial hypertonicity and systemic hypertension. Machnik and colleagues have offered compelling experimental evidence that this hypertonicity is sensed by macrophages, which then produce vascular endothelial growth factor C (VEGF-C), an angiogenic protein. In turn, VEGF-C stimulates increased numbers and density of lymphatic capillaries. In parallel work, using cultured macrophage cell lines subjected to osmotic stress, Go and associates have demonstrated activation of a transcription factor, tonicity-responsive enhancer–binding protein (TonEBP). This factor is known to activate osmoprotective genes in other hypertonic environments, such as the renal medulla. Moreover, analysis of the VEGF-C promoter revealed two TonEBP binding sites and, in subsequent experiments, parallel upregulation of TonEBP and VEGF-C was observed. The effect of TonEBP on VEGF-C was shown to be specific, inasmuch as small interfering RNA for TonEBP and deletion of the murine TonEBP gene, but not nonspecific small interfering RNA, inhibited the VEGF-C upregulation and increased blood pressure. Furthermore, macrophage depletion or inhibition of VEGF-C signaling led to exacerbation of high-salt diet–induced hypertension. Also, an antibody that blocks the lymph-endothelial VEGF-C receptor, VEGFR-3, selectively inhibited macrophage-driven increases in cutaneous lymphatic capillary density, led to skin chloride (Cl − ) accumulation, and induced salt-sensitive hypertension. Mice overexpressing soluble VEGFR3 in epidermal keratinocytes exhibited hypoplastic cutaneous lymph capillaries and increased Na + , Cl − , and water retention in skin and salt-sensitive hypertension. A high-salt diet also led to elevated skin osmolality above plasma levels. In addition, in humans with relatively resistant hypertension, elevated levels of VEGF-C were found, which is consistent with a potential role of this growth factor in the redistribution of excess volume to the intravascular space and exacerbation of hypertension.
Interestingly, mice skin arterioles isolated from animals fed a high-salt diet compared to those on a normal salt diet exhibited increased contractile sensitivity to concentrations of angiotensin II (Ang II) from 10 −10 M upward and to norepinephrine at high doses (10 -5 to 10 -4 M). This salt sensitivity was not observed in muscle arterioles. Finally, a unique human study involving astronauts on the Mars expedition, who received diets with fixed salt intake that varied between 6 and 12 g daily, each for 35 days, was recently reported (reviewed in Reference 14). At each level of salt in the diet, the astronauts reached overall equilibrium between intake and output, as measured in 24-hour urine collections, within the expected 6 days. In parallel, there were the expected early changes in body weight, ECF water, and inverse relationship with the urine aldosterone level. However, changes in total body Na + only occurred after 7 days, and blood pressure reached a new steady state after 3 weeks. Moreover, on the 12-g salt diet, blood pressure continued to rise over a further 4 weeks, with an initial rise and then subsequent fall in body weight and ECF water. During this period, urine aldosterone levels did not change, whereas total body Na + decreased back to original levels, despite the maintained high salt intake. From these data, it appears that intrinsic rhythms with a periodicity of 30 days or more exist for aldosterone and Na + retention, independent of salt intake. Taken together, all these results clearly demonstrate that the skin contains a hypertonic interstitial fluid compartment in which macrophages exert homeostatic and blood pressure regulatory control by local organization of interstitial electrolyte clearance via TonEBP and VEGF-C/VEGFR-3–mediated modification of cutaneous lymphatic capillary function. This compartment may be associated with increased vasoreactivity in precapillary arterioles, the major resistance vessel of rat skin, which could increase peripheral resistance and contribute independently of the kidney to higher blood pressure in salt-sensitive hypertension.
Figure 15.3 summarizes the novel three-compartment model of Na + balance. The reader is also referred to an excellent recent review of this fascinating subject.

Effective Arterial Blood Volume
To understand the mechanisms regulating ECF volume, it is important to appreciate that what is sensed is the effective arterial blood volume (EABV). This can be defined as the part of the ECF in the arterial blood system that effectively perfuses the tissues. More specifically, in physiologic terms, what is sensed is the threat to arterial pressure induced by the EABV that perfuses the arterial baroceptors in the carotid sinus and glomerular afferent arterioles. Any change in perfusion pressure (or stretch) at these sites evokes appropriate compensatory responses. EABV is often, although not always, correlated with actual ECF volume and is proportional to total body Na + . This means that the regulation of Na + balance and the maintenance of EABV are closely related functions. Na + loading generally leads to EABV expansion, whereas loss leads to depletion. However, in several situations, EABV and actual blood volume are not well correlated (see Table 15-5 ). For example, in heart failure (HF), a primary decrease in cardiac output leads to lowered pressure in the perfusion of the baroceptors; that is, reduced EABV is sensed. This leads to renal Na + retention and ECF volume expansion. The net result is a state of increased plasma and total ECF volume, in association with reduced EABV.
The increase in plasma volume is partially appropriate in that intraventricular filling pressure rises and, by increasing myocardial stretching, leads to improved ventricular contractility, thereby raising cardiac output and restoring systemic blood pressure and baroceptor perfusion. However, this response is also maladaptive in that the elevated intraarterial pressure promotes fluid movement out of the intravascular space and into the tissues, which leads to peripheral and pulmonary edema. In HF, EABV is dependent on cardiac output; in other disease settings, however, these two parameters may be dissociated. Dissociation occurs in the presence of an arteriovenous fistula when cardiac output rises in proportion to the blood flow through the fistula. However, the flow through the fistula shunts blood away from the capillaries perfusing the tissues, and therefore the EABV does not rise in conjunction with the rise in cardiac output. Similarly, a fall in systemic vascular resistance—which, together with cardiac output, is a determinant of blood pressure—leads to reductions in blood pressure and EABV.
Another situation in which cardiac output and EABV change in opposite directions is advanced cirrhosis with ascites. ECF volume expands because of the ascites, and plasma volume is increased as a result of fluid accumulation in the splanchnic venous circulation, in which the vessels are dilated but flow is sluggish. Although cardiac output may increase modestly as a result of arteriovenous shunting, marked peripheral vasodilation leads to a fall in systemic vascular resistance, with reductions in EABV and blood pressure. In the presence of reduced EABV, renal perfusion is impaired; under the influence of hormones, such as renin, norepinephrine, and antidiuretic hormone (or arginine vasopressin [AVP])—released in response to the perceived hypovolemia—further Na + and water retention ensue (see later section, “ Efferent Limb: Effector Mechanisms for Maintaining Effective Arterial Blood Volume ”).
To summarize, EABV is an unmeasured index of tissue perfusion that usually, but not always, reflects actual arterial blood volume. Therefore, EABV can be viewed as a functional parameter of organ perfusion. The diagnostic hallmark of reduced EABV is evidence of renal sodium retention, manifested as a urinary sodium (U Na ) level less than 15 to 20 mmol/L.
This relationship holds true with the following exceptions. If renal Na + wasting occurs because of diuretic therapy or intrinsic tubular disease or injury, then U Na is relatively high, despite low EABV. Conversely, the presence of selective renal or glomerular ischemia (e.g., as a result of bilateral renal artery stenosis or acute glomerular injury) will be misinterpreted as indicative of poor renal perfusion and is associated with renal Na + retention (low U Na ).
Regulation of Effective Arterial Blood Volume
Regulation of EABV can be divided into two stages, afferent sensing and efferent effector mechanisms. A number of mechanisms for sensing low EABV exist, all of them primed to stimulate renal Na + retention.
Afferent Limb: Sensing of Effective Arterial Blood Volume
Volume sensors are strategically situated at critical points in the circulation ( Table 15.1 ). Each sensor reflects a specific characteristic of overall circulatory function so that atrial and ventricular sensors sense cardiac filling, arterial sensors respond to cardiac output, and renal, central nervous system (CNS), and gastrointestinal (GI) tract sensors monitor perfusion of the kidneys, brain, and gut, respectively. The common mechanism whereby volume is monitored is by physical alterations in the vessel wall, such as stretch or tension. How exactly this occurs is still not fully elucidated, but the process of mechanosensing probably is dependent on afferent sensory nerve endings in the vessel wall and activation of endothelial cells. Signal transduction mechanisms in endothelial cells include stretch-activated ion channels, cytoskeleton-associated protein kinases, integrin-cytoskeletal interactions, cytoskeletal-nuclear interactions, and generation of reactive oxygen species. In addition, mechanical stretch and tension of blood vessel walls, as well as the frictional forces of the circulation or shear stress, can lead to alterations in gene expression that are mediated by specific recognition sites in the upstream promoter elements of responsive genes. These signals induce efferent effector mechanisms that lead to modifications in renal Na + excretion, appropriate to the volume status.
Sensors of Cardiac Filling |
|
Sensors of Cardiac Output |
Carotid and aortic baroceptors |
Sensors of Organ Perfusion |
|
Sensors of Cardiac Filling
Atrial Sensors.
The pioneering experiments of Henry and associates and Goetz and colleagues in conscious dogs provided a clear demonstration that increased atrial wall tension leads to diuresis and natriuresis. The role of the atria in volume regulation in humans has been elucidated in experiments involving head-out water immersion (HWI) and exposure to head-down tilt or nonhypotensive lower body negative pressure (LBNP). During HWI, the increased hydrostatic pressure of the water on the lower limbs leads to redistribution of the intravascular fluid from the peripheral to central circulation. The resulting increase in central blood volume causes a rise in cardiac output, which in turn produces a brisk increase in Na + and water excretion in an attempt to restore euvolemia. In contrast, LBNP results in a redistribution of blood to the lower limbs, thereby reducing central venous and cardiac filling pressures without affecting arterial pressure, heart rate, or atrial diameter. The resulting retention of Na + and water occurs without any change in renal plasma flow rate (RPF).
Central hypervolemia may not be the only mechanism of HWI-induced Na + and water diuresis. The external hydrostatic pressure of the water also reduces the hydrostatic pressure gradient across the capillary wall in the legs, leading to a net transfer of fluid from the interstitial to intravascular compartment. The resulting hemodilution causes a fall in the colloid osmotic pressure. The hemodilution effect may actually predominate, inasmuch as its abolition by placement of a tight inflated cuff (80 mm Hg) during HWI abrogates the natriuresis. Regardless of which effect is dominant, a combination of hemodilution and central hypervolemia, through atrial stretch, induces neural and humoral changes that bring about the subsequent diuresis and natriuresis.
Neural Pathways.
Two types of neural receptors in the atrium have been described, type A and type B. They are thought to be branching ends of small medullated fibers running in the vagus nerve. Only type B receptor activity is increased by atrial filling and stretch; type A receptors are not affected. The signal is then thought to travel along cranial nerves IX and X to the hypothalamic and medullary centers, where a series of responses is initiated—inhibition of AVP release (left atrial signal), a selective decrease in renal but not lumbar sympathetic nerve discharge, and decreased tone in peripheral precapillary and postcapillary resistance vessels. Conversely, reduction in central venous pressure and atrial volume, as illustrated by LBNP, stimulates renal nerve activity, as assessed by renal norepinephrine spillover and plasma norepinephrine concentration.
The effects just described occur in response to acute atrial stretch, whereas chronic atrial stretch leads to adaptation and downregulation of the neural responses. This phenomenon has been described in rhesus monkeys exposed to 10-degree, head-down tilt. In this model, natriuresis after saline infusion occurs at lower central venous and, hence, lower cardiac filling pressures. Cardiac nerves appear to be essential only for the restoration of Na + balance in states of repletion, but not for the renal response to acute volume depletion. For example, after human cardiac transplantation, a natural model of cardiac denervation, the expected suppression of the renin-angiotensin-aldosterone (RAAS) system in response to chronic volume expansion is not observed.
Humoral Pathways.
Cardiac denervation does not abolish the natriuresis and diuresis during atrial distension. This implies that additional factors other than cardiac nerves are involved in the response to volume repletion. The discovery of a factor in atrial extracts with strong natriuretic and vasodilatory activity led to the isolation and characterization of natriuretic peptides of cardiac origin. The NP family is comprised of atrial NP (ANP), brain NP (BNP), C-type NP (CNP), Dendroaspis NP (DNP), and urodilatin. Although their structures are quite similar, each is encoded by different genes and has distinct, albeit overlapping, functions. The actions of NPs and their interaction with other hormone systems are discussed in detail later (see section, “ Efferent Limb: Effector Mechanisms for Maintaining Effective Arterial Blood Volume ” and Chapter 11 , Chapter 12 , Chapter 13 , Chapter 14 ). This section is confined to a discussion of the afferent mechanisms of NP stimulation.
From studies in animals and humans, it has become abundantly clear that any acute increment in atrial stretch or pressure causes a brisk release in ANP. Every 1-mm Hg rise in atrial pressure results in an approximate rise in ANP of 10 to 15 pmol/L. The process involves the cleavage of the prohormone, located in preformed stores in atrial granules, to the mature 28–amino acid C-terminus peptide in a sequence-specific manner by corin, a transmembrane serine protease. Release of the hormone appears to occur in two steps, the first a Ca 2+ -sensitive K + channel–dependent release of ANP from myocytes into the intercellular space and then a Ca 2+ -independent translocation of the hormone into the atrial lumen. The afferent mechanism for ANP release is activated by intravascular volume expansion and by supine posture, HWI, saline administration, exercise, Ang II, tachycardia, and ventricular dysfunction. Conversely, volume depletion induced by Na + restriction, furosemide administration, or LNBP-mediated reduction in central venous pressure causes a fall in plasma ANP concentration.
The signal transduction pathways, whereby atrial stretch is translated into ANP release, are yet to be fully elaborated. However, a study has shown that mice with a mutation in the acid-sensing ion channel 3 gene or in which the channel was pharmacologically inhibited have a blunted response in blood volume expansion–induced urine flow.
In contrast to the effects of acute changes in atrial pressure on ANP release, the role of this peptide in the long-term regulation of plasma volume appears to be modest, at best. For example, although incremental oral salt loading was associated with correspondingly higher baseline plasma ANP levels, only intravenous (not oral) salt loading led to increased ANP levels. Moreover, in humans subjected to intravenous or oral salt loading, no correlation could be found between changes in ANP levels and the degree of natriuresis. The contrasting relationships among acute and chronic Na + loading, plasma ANP levels, and natriuresis have been elegantly demonstrated in ANP gene knockout mice. These mice display a reduced natriuretic response to acute ECFV expansion in comparison with their wild-type counterparts. However, no differences in cumulative Na + and water excretion were observed between the knockout and wild-type mice after a high- or low-Na + diet for 1 week. The only difference between the two types of mice was a significant increase in mean arterial pressure. Further experiments using disruptions of the genes for ANP or its receptor, guanylate cyclase A (GC-A), have shown the importance of this system in the maintenance of normal blood pressure and in modulating cardiac hypertrophy.
In contrast to ANP, the other members of the NP family appear not to be involved in the physiologic regulation of Na + excretion. Thus, results of gene disruption studies involving BNP, CNP, or the guanylate cyclase B (GC-B) receptor indicated that these proteins exert local paracrine-autocrine cyclic guanosine monophosphate (cGMP)–mediated effects on cellular proliferation and differentiation in various tissues. In summary, of the various NPs, only ANP appears to have a direct role in sensing volume in the atria.
Ventricular and Pulmonary Sensors.
Volume sensors have been found in the ventricles, coronary arteries, main pulmonary artery and bifurcation, and juxtapulmonary capillaries in the interstitium of the lungs, but not in the intrapulmonary circulation. These sensors have generally been considered as mediating reflex changes in heart rate and systemic vascular resistance through modulation of the sympathetic nervous system (SNS) and of ANP. This also appears to be true for the coronary baroceptor reflex described in anesthetized dogs, by which changes in coronary artery pressure lead to alterations in lumbar and renal sympathetic discharge and a coronary artery response much slower than that of the carotid and aortic baroceptors. However, some evidence, also in dogs, has suggested that ventricular and pulmonary sensors may also detect changes in blood volume through increased left ventricular pressure, which causes a reflex inhibition of plasma renin activity (PRA).
Sensors of Cardiac Output
The sensors described so far are situated in low-pressure sites, where they sense the fullness of the circulation and are probably more important for defending against excessive volume expansion and the consequent congestive manifestations of cardiac failure. The arterial (high-pressure) sensors, on the other hand, are geared more toward detecting low cardiac output or systemic vascular resistance, which manifest as underfilling of the vascular tree (i.e., EABV depletion threatening arterial pressure ) and as signaling the kidneys to retain Na + . These high-pressure sensors are found in the aortic arch, carotid sinus, and renal vessels.
Carotid and Aortic Baroceptors.
Histologic and molecular analysis of the carotid baroceptor has revealed a large content of elastic tissue in the tunica media, which makes the vessel wall highly distensible in response to changes in intraluminal pressure, thereby facilitating transmission of the stimulus intensity to sensory nerve terminals. A change in the mean arterial pressure induces depolarization of these sensory endings, which results in action potentials. Transient receptor potential vanilloid receptors may mediate this process. Afferent signals from the baroceptors are integrated in the nucleus tractus solitarius (NTS) of the medulla oblongata, which leads to reflex changes in systemic and renal sympathetic nerve activity (RSNA) and, to a lesser degree, release of AVP. A role for endocannabinoids has been postulated in baroceptor reflex modulation. In this regard, a significant increase in the endocannabinoid anandamide in the NTS was observed after an increase in blood pressure. Also, anandamide microinjections into the NTS induced prolonged baroreflex inhibition of RSNA. The cannabinoid effect appears to be mediated by activation of 5-hydroxytryptamine type 1A (5-HT1A) receptors. These results, along with other studies, support the hypothesis that endogenous anandamide can modulate the baroreflex through cannabinoid CB(1) receptor activation within the nucleus tractus solitarius. Conversely, hypovolemia-induced activation of NTS (A1) adenosine receptors may serve as a negative feedback regulator of sympathoinhibitory reflexes integrated in the NTS. An important additional function of the carotid baroceptors is maintenance of adequate cerebral perfusion. The aortic baroceptor appears to behave in a way similar to the carotid baroceptor. Finally, there is evidence in dogs for an interaction between pulmonary arterial and carotid sinus baroceptor reflexes.
Sensors of Organ Perfusion
Renal Sensors.
The kidney not only is the major effector target responding to signals that indicate the need for adjustments in Na + excretion, but also has a central role in the afferent sensing of volume homeostasis by virtue of the local sympathetic innervation. However, despite considerable knowledge concerning the mechanisms of renal sensing of EABV, the molecular identity and exact cellular location of the renal sensor or sensors remain elusive. The integral relationship between afferent and efferent renal sympathetic activities and the central arterial baroceptors was highlighted by Kopp and colleagues. They showed that a high-Na + diet increases afferent RSNA, which then decreases efferent RSNA and leads to natriuresis. Using dorsal rhizotomy to induce afferent renal denervation in rats maintained on a high-Na + diet, they demonstrated increased mean arterial pressure that was dependent on impaired arterial baroreflex suppression of efferent RSNA activity. Animals fed a normal-Na + diet displayed no changes in arterial baroceptor function. Kopp and coworkers concluded that arterial baroreflex function contributes to increased efferent RSNA, which, in the absence of intact afferent RSNA, would eventually lead to Na + retention and hypertension. The role of RSNA in Na + regulation is discussed further later in this chapter (see section, “ Neural Mechanisms: Renal Nerves and Sympathetic Nervous System ”).
An additional level of renal sensing depends on the close anatomic proximity of the sensor and effector limbs to one another: Volume changes may be sensed through alterations in glomerular hemodynamics and renal interstitial pressure. These alterations result simultaneously in adjustments in the physical forces governing tubular Na + handling (see section, “ Efferent Limb: Effector Mechanisms for Maintaining Effective Arterial Blood Volume ”).
The kidneys, along with other organs, have the ability to maintain a constant blood flow and constant glomerular filtration rate (GFR) at varying arterial pressures. This phenomenon, termed autoregulation, operates over a wide range of renal perfusion pressures (RPPs). Autoregulation of renal blood flow (RBF) occurs through three mechanisms—the myogenic response, tubuloglomerular feedback (TGF), and a third mechanism. In the myogenic response, changes in RPP are sensed by smooth muscle elements that serve as baroreceptors in the afferent glomerular arteriole and dynamically respond by adjusting transmural pressure and tension across the arteriolar wall. An example of this can be seen in Ang II–infused rats on a high salt intake. These animals show reduced dynamic autoregulation of RBF, an effect mediated, at least in part, by superoxide. The myogenic response is attenuated by endothelial nitric oxide synthase (eNOS)–dependent production of nitric oxide.
The second mechanism, TGF, is operated by the juxtaglomerular apparatus, which is comprised of the afferent arteriole and, to a lesser extent, the cells of the macula densa in the early distal tubule. The juxtaglomerular apparatus is also important because of its involvement in the synthesis and release of renin. The physiologic release of renin from the cells of the juxtaglomerular apparatus is controlled by three pathways, all of which are driven by EABV status. First, renin release is inversely related to RPP and directly related to intrarenal tissue pressure. When RPP falls below the autoregulatory range, renin release is further enhanced. Second, renin secretion is influenced by solute delivery to the macula densa. Increased NaCl delivery past the macula densa leads to inhibition of renin release, whereas a decrease has the opposite effect. Sensing at the macula densa is mediated by NaCl entry through the Na + -K + -2Cl − cotransporter (NKCC2), which leads to alterations in intracellular Ca 2+ together with the production of prostaglandin E 2 (PGE 2 ), adenosine, and, subsequently, renin release. Third, changes in renal nerve activity influence renin release. Renal nerve stimulation increases renin release through direct activation of β-adrenergic receptors on juxtaglomerular cells. This effect is independent of major changes in renal hemodynamics. Sympathetic stimulation also affects intrarenal baroreceptor input, composition of the fluid delivered to the macula densa, and renal actions of Ang II so that renal nerves may serve primarily to potentiate other regulatory signals.
The nature of the third mechanism of RBF autoregulation is still unclear, but Seeliger and associates, using a normotensive Ang II clamp in anesthetized rats, were able to abolish the resetting of autoregulation during incremental shaped RPP changes. Under control conditions, the initial TGF response was dilatory after total occlusions but constrictive after partial occlusions. The initial third mechanism response was a mirror image of TGF, it was constrictive after total occlusions but dilatory after partial occlusions. The angiotensin clamp suppressed the TGF and turned the initial third mechanism response after total occlusions into dilation. Seeliger and coworkers reached the following conclusions: (1) pressure-dependent renin angiotensin system (RAS) stimulation was a major factor behind hypotensive resetting of autoregulation; (2) TGF sensitivity depended strongly on pressure-dependent changes in RAS activity; (3) the third mechanism was modulated, but not mediated, by the RAS; and (4) the third mechanism acted as a counterbalance to TGF. They proposed that their findings might be related to feedback between the connecting tubule and glomerulus. TGF is discussed later (see section, “ Integration of Changes in Glomerular Filtration Rate and Tubular Reabsorption ”).
Central Nervous System Sensors.
Certain areas in the CNS appear to act as sensors to detect alterations in body salt balance. This was suggested originally by results of experiments in rats, in which intracerebral injection of hypertonic saline led to reduced renal nerve activity and natriuresis. Subsequently, DiBona showed that administration of Ang II into the cerebral ventricles and changes in dietary Na + modulate baroreflex regulation of RSNA. Similarly, stimulation of neurons located in the paraventricular nucleus and in a region extending to the anteroventral third ventricle led to ANP release, inducing Ang II blockade and inhibition of salt and water intake. Conversely, disruption of these neurons, as well as of the median eminence or neural lobe, led to decreased ANP release and impaired response to volume expansion. Overall, despite the substantial evidence for CNS sensing of ECF volume status, the exact nature, mode of operation, and relative importance of this aspect of sensing remains unclear.
Gastrointestinal Tract Sensors.
Under normal physiologic conditions, Na + and water reach the ECF by absorption in the GI tract. Therefore, it is not surprising that sensing and regulatory mechanisms of ECF volume have been found in the GI tract itself. The evidence for this phenomenon comes from experiments that showed more rapid natriuresis after an oral salt load than after a similar intravenous load. Moreover, infusions of hypertonic saline into the portal vein led to greater natriuresis than similar infusions into the femoral vein. These findings were consistent with the presence of Na + -sensing mechanisms in the splanchnic or portal circulation, or both. In fact, these mechanisms appear to be located primarily in the portal system and are probably important in the pathogenesis of the hepatorenal syndrome (HRS; see later).
Hepatoportal Receptors.
The two main neural reflexes, termed the hepatorenal and hepatointestinal reflexes, originate from receptors in the hepatoportal region. They transduce portal plasma Na + concentration into hepatic afferent nerve activity; before a measurable increase in systemic Na + concentration occurs, the hepatointestinal reflex attenuates intestinal Na + absorption via the vagus nerve, and the hepatorenal reflex augments Na + excretion. These reflexes have been observed in rats and rabbits, as well as in humans, and have been shown to be impaired in the chronic bile duct ligation model of cirrhosis and portal hypertension. In addition, the hepatic artery shows significant autoregulatory capacity, dilating when perfusion pressure falls and constricting when pressure rises, thereby maintaining hepatic arterial blood flow over a wide range of perfusion pressures. Moreover, there is extensive crosstalk between the portal and systemic circulations. For example, when portal blood flow decreases, the hepatic artery dilates; this is indicative of the presence of a sensor in the hepatic artery, which responds to changes in the contribution of the portal vein to total hepatic blood flow. Clues to the mechanism of hepatic autoregulation come from models of reduced portal venous blood flow and acute hepatic injury, in which reduced Na + excretion was abolished by administration of an A 1 adenosine receptor (A1AR) antagonist; thus, these receptors probably have a role in the hepatorenal reflex.
The observation that intraportal infusion of bumetanide or furosemide suppresses the response of hepatic afferent nerve activity to intraportal hypertonic saline suggests that the NKCC2 may be involved in sensing portal Na + concentration. In addition to hepatoportal Na + -sensing chemoreceptors, the liver also contains mechanoreceptors (baroreceptors). Increased intrahepatic hydrostatic pressure has been shown to be associated with enhanced RSNA and renal Na + retention in various experimental models. For example, when increased intrahepatic pressure was induced by thoracic caval constriction in dogs, raising venous pressure led to a positive Na + balance, which was inhibited by liver denervation. A clinical model for increased intrahepatic pressure is the Budd-Chiari syndrome, and it is in situations such as this that hepatic volume-sensing mechanisms probably play a role in renal Na + retention (see section, “ Specific Treatments Based on the Pathophysiology of Sodium Retention in Cirrhosis ”).
Intestinal Natriuretic Hormones: Guanylin Peptides.
As described previously, the natriuretic response of the kidneys to a Na + load is more rapid when the load is delivered orally than when the same load is administered intravenously. The different responses are observed without accompanying differences in plasma aldosterone. This observation led to the idea that the gut produces a substance that signals the kidneys to excrete excess Na + . The discovery of the guanylin family of cGMP-regulating peptides (intestinal natriuretic hormones) has shed light on this phenomenon. Of the four currently known guanylin peptides, guanylin and uroguanylin have been best studied. They are small (15 to 16 amino acids), heat-stable peptides with intramolecular disulfide bridges similar to the bacterial heat-stable enterotoxins that cause traveler’s diarrhea and are found in mammals, birds, and fish.
Both guanylin and uroguanylin are synthesized as prepropeptides, primarily in the intestine. The former, produced mainly by the ileum through the proximal colon, circulates as proguanylin; the latter, which is expressed principally in the jejunum, circulates in its active form. A physiologically important difference between guanylin and uroguanylin lies in their sensitivity to proteases. Because of a tyrosine residue at the ninth amino acid, guanylin is sensitive to protease digestion in the kidneys, which leads to its inactivation, whereas uroguanylin can be locally activated by the same proteases. After an oral salt load, guanylin and uroguanylin released in the intestine lead to increased intestinal secretion of Cl − , HCO 3 − , and water and to inhibition of Na + absorption.
In the kidneys, Na + , K + , and water excretion is increased, without any change in RBF or GFR and independently of RAAS, AVP, or ANPs. The signaling pathway of guanylin peptides in the intestine involves binding to and activation of the receptor guanylate cyclase C (GC-C), one of the eight guanylate cyclases. GC-C is a transmembrane protein, composed of 1050 to 1053 amino acids, that is present in the intestinal brush border. Propagation of the signal occurs through the second messenger cGMP, which inhibits Na + / H + exchange and activates protein kinase G II and protein kinase A. These in turn activate the cystic fibrosis transmembrane conductance regulator (CFTR), leading to Cl − secretion. CFTR then activates the Cl − /HCO 3 − exchanger, which leads to HCO 3 − secretion.
The best evidence for a link between the gut and kidneys comes from mice lacking the uroguanylin gene, which display an impaired natriuretic response to oral salt loading but not to intravenous NaCl infusion. However, because plasma pro-uroguanylin levels do not rise but urinary uroguanylin levels do increase after a high-salt meal, locally released peptide by the kidneys could still play a role in uroguanylin-associated natriuresis. In the kidneys, both GC-C–dependent and GC-C–independent signaling pathways for guanylin peptides exist, inasmuch as knockout of GC-C in mice does not affect the high-salt diet–induced increase in uroguanylin. From experiments on cell lines and isolated tubules, it appears that uroguanylin acts on the proximal tubule and principal cells of the cortical collecting duct.
In proximal cell lines, guanylin peptides increase cGMP and decrease cyclic adenosine monophosphate (cAMP), which leads to inhibition of Na + /H + exchange and Na + -K + -ATPase; such events are consistent with decreased Na + reabsorption in this segment. Crosstalk between guanylin peptides and ANPs may also occur in the proximal tubule. In the principal cell, uroguanylin activation of a G protein–coupled receptor results in phospholipase A 2 –dependent inhibition of the renal outer medullary potassium (ROMK) channel, which leads to depolarization and a reduced driving force for Na + reabsorption. There is also evidence that guanylin may cause cell shrinkage in the inner medullary collecting duct (IMCD), which is suggestive of water secretion from this segment and a role in water diuresis. Together, these data are highly suggestive of a role at least for uroguanylin, as a natriuretic hormone, in adjusting U Na excretion to balance the levels of NaCl absorbed via the GI tract. However, recent work has cast substantial doubt on this GI renal signaling axis for Na regulation. The importance of this system in the control of renal Na + excretion in humans awaits further clarification.
A final point is that although multiple receptors are clearly involved in the regulation of EABV, their functions appear to be considerably redundant. For example, cardiac or renal denervation in nonhuman primates and chronic aldosterone administration do not significantly affect the maintenance of Na + balance.
Efferent Limb: Effector Mechanisms for Maintaining Effective Arterial Blood Volume
The maintenance of Na + homeostasis is achieved by adjustment of renal Na + excretion according to the body’s needs. Like the mechanisms sensing changes in EABV, there are a number of pathways that enable the required adjustments in renal Na + excretion. The adjustments are made by integrated changes in GFR and tubular reabsorption, so that changes in one component lead to appropriate changes in the other to maintain Na + homeostasis. In addition, tubular reabsorption is regulated by local peritubular and luminal factors and by neural and humoral mechanisms ( Table 15.2 ).
Glomerular Filtration Rate and Tubular Reabsorption |
|
Neural Mechanisms |
|
Humoral Mechanisms |
|
Integration of Changes in Glomerular Filtration Rate and Tubular Reabsorption
In humans, normal GFR leads to the delivery of approximately 24,000 mmol of Na + /day for downstream processing by the tubules. More than 99% of the filtrate is reabsorbed; only a tiny amount escapes into the final urine. Therefore, it is clear that even minute changes in the relationship between filtered load and fraction of Na + absorbed can exert a profound cumulative influence on net Na + balance. However, even marked perturbations in GFR are not necessarily associated with drastic alterations in U Na excretion; thus, overall Na + balance is usually preserved. Such preservation results from appropriate adjustments in two important protective mechanisms—TGF, in which changes in tubular fluid Na + inversely affect GFR, and glomerulotubular balance, whereby changes in tubular flow rate resulting from changes in GFR directly affect tubular reabsorption.
Tubuloglomerular Feedback
A remarkable feature of nephron architecture is that after emerging from Bowman’s capsule and descending deep into the medulla, each tubule returns to its parent glomerulus. Guyton and associates envisioned a functional relationship between the tubule and glomerulus; this concept led to a wealth of experimental evidence supporting the existence of TGF (see also Chapter 3 ). TGF operates by changes in tubular fluid Na + at the macula densa (the point of contact between the specialized tubular cells of the cortical thick ascending limb of Henle adjacent to the extraglomerular mesangium), which elicit adjustments in glomerular arteriolar resistance. The system is constructed as a negative feedback loop in which an increase in NaCl concentration leads to increases in afferent arteriolar resistance and a consequent fall in the GFR. This, in turn, leads to an increase in proximal reabsorption and a reduction in distal delivery of solute. Thus, NaCl delivery to the distal nephron is maintained within narrow limits.
The complexities of TGF and detailed mechanisms of changes in epithelial function in response to luminal NaCl composition were unraveled initially by elaborate micropuncture studies that clearly established the tubular-glomerular link and, subsequently, by imaging and electrophysiologic techniques in isolated perfused tubule/glomerulus preparations. However, the signaling mechanisms linking changes in tubular composition with altered glomerular arteriolar tone became evident only much later through experiments in gene-manipulated mice. The primary detection mechanism of TGF appears to be uptake of salt by means of the NKCC2, located in the apical membrane of macula densa cells. The evidence comes from TGF inhibition by inhibitors of the cotransporter, furosemide and bumetanide, and by deletions in mice of the A or B isoform of NKCC2, both of which are expressed in macula densa cells. In fact, complete inactivation of the NKCC2 gene leads to the severe salt-losing phenotype of antenatal Bartter syndrome. Similarly, inhibition or deletion of the ROMK channel in mice abolishes TGF.
The next step in the juxtaglomerular cascade is less clear. One possibility is direct coupling of NKCC2-dependent NaCl uptake to the mediation step. Results of studies in the isolated perfused rabbit juxtaglomerular apparatus have indicated that depolarization, alkalinization, and various ionic compositional changes occur after increased NaCl uptake; thus, one or more of these changes could trigger the signal. A second possibility is that signal propagation is the consequence of transcellular NaCl transport and Na + – K + – ATPase–dependent basolateral extrusion. Experiments using double-knockout mice, in which the α 1 -subunit of Na + – K + – ATPase was made sensitive and the α 2 -subunit resistant to the pump inhibitor, ouabain, clearly indicate an important role for Na + – K + – ATPase in supporting TGF and that adenosine triphosphate (ATP) consumption is required for the process.
In contrast, there is strong evidence that ATP release and degradation, rather than consumption, may be the link in the chain connecting NaCl changes in the macula densa with alteration of glomerular arteriolar tone. According to the current working model, after NaCl uptake and transcellular transport, ATP is released from macula densa cells and undergoes stepwise hydrolysis and dephosphorylation by ecto-ATPases and nucleotidases to adenosine diphosphate, adenosine monophosphate, and then adenosine, which, in a paracrine manner, causes A1AR–dependent afferent arteriolar constriction. Although the evidence for ATP breakdown is as yet incomplete, evidence for the effects of adenosine as a mediator of TGF is very strong. For example, isolated perfused mouse afferent arterioles exposed to adenosine display vigorous vasoconstriction, an effect not seen in A1AR–deficient mice. As shown by overexpression and conditional knockout of the receptor, this A1AR effect is primarily on afferent arteriolar smooth muscle cells, although A1AR effects on extravascular, perhaps mesangial, cells appear to contribute to the TGF response. The response is mediated by inhibitory G protein (G i )–dependent activation of phospholipase C, release of Ca 2+ from intracellular stores, and subsequent entry of Ca 2+ through L-type Ca 2+ channels. Cellular adenosine uptake is likely to be involved in the TGF response because targeted deletion of the type 1 equilibrative nucleoside transporter (ENT1) led to significant attenuation of the response. Of particular interest is the fact that the vasodilatory adenosine A 2 receptor is more abundant than the A1AR in the renal vasculature, and continuous exogenous application of adenosine to mouse kidneys is indeed vasodilatory. However, the generation of adenosine in the confines of the juxtaglomerular interstitium and its exclusive delivery to the afferent arteriole, where A1AR expression predominates, ensures the appropriate response for TGF.
Other factors, both co-constrictors and modulators, appear to be involved in TGF. Ang II has been shown to act as an important cofactor in the vasoconstrictive action of adenosine. In this regard, deletions of the Ang II receptor or angiotensin-converting enzyme (ACE) in mice were found to abolish TGF. The effect may result from nonresponsiveness to adenosine in the absence of an intact RAS. By contrast, aldosterone has recently been shown to blunt TGF through the activation of mineralocorticoid receptors on macula densa cells. This effect may be mediated by superoxide, which in turn may also be upregulated by Ang II via the NOX2 and NOX4 isoforms of NADPH (nicotinamide adenine dinucleotide phosphate) oxidase. The high levels of neuronal nitric oxide synthase (nNOS) expression in macula densa cells are indicative of a role for nitric oxide in TGF. Nitric oxide is thought to counterbalance Ang II–induced efferent arteriolar vasoconstriction and to modulate renin secretion by the juxtaglomerular apparatus. Consistent with this concept is the finding that chronic absence of functional nNOS in macula densa cells is associated with enhanced vasoconstriction in the subnormal flow range, probably as a result of proportional increases in preglomerular and postglomerular tone. In addition, increased delivery of fluid to the macula densa induces nitric oxide release from these cells.
Inhibition of the nitric oxide system by nonselective blockers of nitric oxide synthase (NOS) results in an exaggerated TGF response that leads to even further renal vasoconstriction, Na + and water retention, and arterial hypertension. Also, TGF responses are absent in mice with concurrent deficiencies in nNOS and the A1AR, which implies that nNOS deficiency does not overcome deficient A1AR signaling. Moreover, nitric oxide modulation of TGF can be mediated by ecto 5′-nucleotidase, the enzyme responsible for adenine formation. Furthermore, nitric oxide, via eNOS, modulates the afferent arteriolar myogenic response. Finally, aldosterone-induced modulation of TGF appears to involve interactions between nitric oxide and superoxide. Together, these data suggest that A1AR signaling is primary and that nNOS and eNOS, as well as superoxide, play modulatory roles in TGF.
Apart from the RAAS, other hormonal systems and secondary messengers appear to be involved in TGF. For example, stimulation of the glucagon-like peptide 1 receptor leads to an increased GFR and reduced proximal tubular reabsorption. Moreover, high salt intake–induced activation of AMP-activated protein kinase leads to an enhanced TGF response and increased delivery of Na + to the end of the proximal tubule. Furthermore, acute saline expansion leads to an increased single-nephron glomerular filtration rate (SNGFR) and distal nephron flow rate, an effect independent of the Ang II receptor.
The afferent arteriolar A1AR may not be the sole mediator of TGF. Activation by adenosine of the adenosine A 2 receptor has been shown to dilate mouse cortical efferent receptors. The effect appeared to be mediated by the low-affinity adenosine A 2b receptor via increased levels of eNOS. It is remarkable that this highly specific effect occurs despite the presence of A1AR in the efferent arteriole. Apparently, therefore, the relative abundance of the various adenosine receptor subtypes in afferent and efferent arterioles ultimately allows fine-tuning of TGF by concerted changes in glomerular vascular tone. On the other hand, purine receptors do not seem to affect TGF.
Connexin 40, which plays a predominant role in the formation of gap junctions in the vasculature, also participates in the autoregulation of RBF by the afferent arteriole and, therefore, in TGF. Connexin-40 knockout mice displayed impaired steady-state autoregulation to a sudden step increase in RPP. A marked reduction in TGF in connexin 40 knockout mice was thought to be responsible. Connexin 40–mediated RBF autoregulation occurred by paracrine signaling between tubular and vascular cells to the afferent arteriole, independently of nitric oxide. Other endogenous modulators of TGF include the eicosanoid 20-hydroxyeicosatetraenoic acid (20-HETE), which modifies the myogenic afferent arteriolar and TGF responses, and the heme oxygenase pathway via carbon monoxide and cGAMP generation, which inhibits TGF through inhibition of depolarization and Ca 2+ entry in macular densa cells.
In addition to hormones of the RAAS, sex hormones also appear to regulate TGF. Testosterone in rats leads to upregulation of TGF by generation of superoxide dismutase, whereas enhanced Ang II receptor activity attenuates Ang II–dependent resetting of TGF activity in female rats. A final point in the complexity of TGF is that there is evidence for three sites in addition to the macula densa that are in contact with the efferent arteriole—the terminal cortical thick ascending limb of Henle, the early distal tubule, and the connecting tubule. In particular, perimacular cells and oscillatory cells of the early distal tubule may be involved in the intracellular Ca 2+ signaling required for adenosine-induced afferent vasoconstriction. On the other hand, the effect of the connecting tubule on the afferent arteriolar tone appears to be modulatory in that elevations in luminal NaCl and cellular Na + entry via the epithelial sodium channel (ENaC) lead to afferent arteriolar dilation through the release of prostaglandins and epoxyeicosatrienoic acids. Moreover, connecting tubule glomerular feedback has been shown to antagonize TGF, at least in the acute setting.
Glomerulotubular Balance
Several factors are involved in the phenomenon of glomerulotubular balance (GTB), which describes the ability of proximal tubular reabsorption to adapt proportionally to the changes in filtered load.
Peritubular Capillary Starling Forces.
Researchers have studied the natriuretic response to ECFV expansion by examining the effects of acute infusions of saline or albumin in experimental animals and in humans. Therefore, their relevance to the chronic regulation of ECF sodium balance is questionable. Nevertheless, the findings from these studies led to the notion that alterations in hydraulic and oncotic pressures (Starling forces) in the peritubular capillary play an important role in the regulation of Na + and water transport, especially in the proximal nephron. The peritubular capillary network is anatomically connected in series with the glomerular capillary bed of cortical glomeruli through the efferent arteriole; thus, changes in the physical determinants of GFR critically influence Starling forces in the peritubular capillaries.
Of importance is that about 10% of glomeruli, mainly those at the corticomedullary junction, are connected in series to the vasa recta of the medulla. In the proximal tubule—whose peritubular capillaries receive 90% of blood flow from glomeruli—the relationship of hydraulic and oncotic driving forces to the transcapillary fluid flux is given by the Starling equation, as follows:
Rate abs = K r [ ( π c − π i ) − ( P c − P i ) ]
Also, because the peritubular capillary receives blood from the glomerulus, the plasma oncotic pressure is high at the outset as a result of prior filtration of protein-free fluid. It follows that the greater the GFR in relation to plasma flow rate, the higher the protein concentration in the efferent arteriolar plasma and the lower the hydraulic pressure in the proximal peritubule capillary. As a consequence, proximal fluid reabsorption is enhanced ( Figure 15.4 ). Therefore, in contradistinction to the glomerular and peripheral capillary, the peritubular capillary is characterized by high values of π c − π i that greatly exceed P c − P i , which results in net reabsorption of fluid.

The ratio of GFR to RBF defines the filtration fraction. The relationship of proximal reabsorption to filtration fraction may contribute to Na + -retaining and edema-forming states, such as heart failure (see Figure 15.4 ). A series of in vivo micropuncture and microperfusion studies, as well as studies using the isolated perfused tubule model, have yielded compelling experimental evidence for the relationship between proximal peritubular Starling forces and proximal fluid reabsorption. As a result of these studies, the role of peritubular forces in the setting of increased ECF volume can be summarized as follows:
- 1.
Acute saline expansion results in dilution of plasma proteins and reduction in efferent arteriolar oncotic pressure. The SNGFR and peritubular P c may be increased as well, but the decrease in peritubular π c by itself results in a decreased net peritubular capillary reabsorptive force and decreased Rate abs . GTB is disrupted because Rate abs falls, despite the tendency for SNGFR to rise, and this development allows the excess Na + to be excreted and plasma volume to be restored.
- 2.
Iso-oncotic plasma infusions tend to raise SNGFR and peritubular P c but lead to relative constancy of efferent arteriolar oncotic pressure. Rate abs may therefore decrease slightly, resulting in less disruption of GTB and natriuresis of lesser magnitude than that observed with saline expansion.
- 3.
Hyperoncotic expansion usually increases both SNGFR (because of volume expansion) and efferent arteriolar oncotic pressure. As a result, Rate abs is enhanced. GTB therefore tends to be better preserved than with iso-oncotic plasma or saline expansion.
- 4.
Changes in π i can directly alter proximal tubular reabsorption, independently of the peritubular capillary bed.
The alterations in proximal peritubular Starling forces that modulate fluid and electrolyte movements across the peritubular basement membrane into the surrounding capillary bed appear to be accompanied by corresponding changes in the structure of the peritubular interstitial compartment. Ultrastructural data from rats have suggested that the peritubular capillary wall is in tight apposition to the tubule basement membrane for about 60% of the tubule basolateral surface. However, irregularly shaped wide portions of peritubular interstitium also exist over about 40% of the tubule basolateral surface; thus, a major portion of reabsorbed fluid has to cross a true interstitial space before entering the peritubular capillaries. Alterations in the physical properties of the interstitial compartment could conceivably modulate passive or active components of net fluid transport in the proximal tubule. Starling forces in the peritubular capillary are thought to regulate the rate of volume entry from the peritubular interstitium into the capillary. Any change in this rate of flux could lead to changes in interstitial pressure that secondarily modify proximal tubule solute transport. This formulation could explain why experimental maneuvers known to raise P i (e.g., infusion of renal vasodilators, renal venous constriction, renal lymph ligation) were associated with a natriuretic response, whereas the opposite effect was seen with renal decapsulation, which lowers P i (see section, “ Medullary Hemodynamics and Interstitial Pressure in the Control of Sodium Excretion: Pressure Natriuresis ”).
Because of the relatively high permeability of the proximal tubule, changes in interstitial Starling forces are likely to be transduced mainly through alterations in passive bidirectional paracellular flux through the tight junctions. The claudin family of adhesion molecules has clearly proved that the tight junction is a dynamic, multifunctional complex that may be amenable to physiologic regulation by cellular second messengers or in pathologic states. Among the 24 known mammalian claudin family members, at least three—claudin-2, claudin-10, and claudin-11—are located in the proximal nephron of the mouse, and others are located at more distal nephron sites. Claudin-2 is selectively expressed in the proximal nephron. However, the exact role of the claudin family members in the influence of Starling forces on fluid reabsorption remains to be elucidated.
Luminal Composition.
In addition to peritubular capillary and interstitial Starling forces, luminal factors may also play a role in the regulation of proximal tubule transport. For example, Romano and colleagues have shown that GTB could be fully expressed, even when the native peritubular environment was kept constant while the rate of perfusion of proximal tubular segments with native tubular fluid was changed. Moreover, studies in isolated perfused rabbit proximal tubules have indicated that the presence of a transtubular anion gradient, normally present in the late portion of the proximal nephron, is necessary for the flow dependence to occur. A potential mechanism for the modulation of proximal Na + reabsorption in response to changes in filtered load depends on the close coupling of Na + transport with the cotransport of glucose, amino acids, and other organic solutes. The increased delivery of organic solutes that accompanies increases in GFR, together with the preferential reabsorption of Na + with bicarbonate in the early proximal tubule, would lead to increased delivery of both Cl − and organic solutes to the late proximal tubule. The resulting transtubular anion gradient would then facilitate the “passive” reabsorption of the organic solutes and NaCl in this segment, but overall net reabsorption would be reduced.
In summary, regardless of the exact mechanism, ECFV expansion impairs the integrity of GTB, thus allowing increased delivery of salt and fluid to more distal parts of the nephron. The major factors acting on the proximal nephron during a decrease in ECF and effective arterial circulating volume are outlined schematically in Figure 15.5 .

Physical Factors Beyond the Proximal Tubule.
Because the final urinary excretion of Na + , in response to volume expansion or depletion, can be dissociated from the amount delivered out of the superficial proximal nephron, more distal or deeper segments of the nephron contribute to the modulation of Na + and water excretion. Several sites along the nephron, such as the loop of Henle, distal nephron, and cortical and papillary collecting ducts, were found (by micropuncture and microcatheterization techniques) to increase or decrease the rate of Na + reabsorption in response to enhanced delivery from early segments of the nephron. However, direct evidence that these transport processes are mediated by changes in Starling forces per se is lacking. Jamison and coworkers have provided a detailed review of these experiments.
In summary, the intrarenal control of Na + excretion can be generalized as follows. If ECFV is held relatively constant, an increase in GFR leads to little or no increase in salt excretion because of a close coupling between the GFR and intrarenal physical forces acting at the peritubular capillary to control Rate abs . In addition, changes in the filtered load of small organic solutes, and perhaps other, as yet uncharacterized, glomerulus-borne substances in tubule fluid may influence Rate abs . To the extent that changes, if any, in the load of Na + delivered to more distal segments also occur, parallel changes in distal reabsorptive rates also occur to ensure a high degree of GTB for the kidneys as a whole. Conversely, ECFV expansion leads to large increases in Na + excretion, even in the presence of a reduced GFR. Changes in Na + reabsorption in the proximal tubule alone cannot account for this natriuresis of volume expansion, and a number of mechanisms for suppressing renal Na + reabsorption at more distal sites has been proposed.
Medullary Hemodynamics and Interstitial Pressure in the Control of Sodium Excretion: Pressure Natriuresis.
The idea that changes in renal medullary hemodynamics may be involved in the natriuresis evoked by volume expansion was initially proposed in the 1960s by Earley and Friedler and colleagues. According to their theory, ECFV expansion results in an increase in RPP that is transmitted as an increase in medullary plasma flow; this leads to a subsequent loss of medullary hypertonicity, elimination of the medullary osmotic gradient (“medullary washout”) and, thereby, decreased water reabsorption in the thin descending loop of Henle. The decrease in water reabsorption in the thin descending limb lowers the Na + concentration in the fluid entering the ascending loop of Henle, thus decreasing the transepithelial driving force for salt transport in this nephron segment. At the same time, a similar mechanism was proposed to explain the natriuresis after elevations in systemic blood pressure, a phenomenon termed pressure natriuresis.
The concept that alterations in the solute composition of the renal medulla and papilla play a key role in the regulation of Na + transport gained significant support in the 1970s and 1980s, when results of micropuncture studies suggested that volume expansion, renal vasodilation, and increased RPP produced a greater inhibition of salt reabsorption in the loops of Henle within juxtamedullary nephrons than in those within superficial nephrons. Measurement of medullary plasma flow with laser Doppler flowmetry and videomicroscopy in experimental animals has provided strong evidence for the redistribution of intrarenal blood flow toward the medulla after volume expansion and renal vasodilation. These studies were of particular interest with regard to the role of medullary hemodynamics in the control of Na + excretion, especially in the context of pressure natriuresis.
The importance of pressure natriuresis in the long-term control of arterial blood pressure and ECFV regulation was first recognized by Hall and associates. According to this view, the kidneys alter Na + excretion in response to changes in arterial blood pressure. For example, an increase in RPP results in a concomitant increase in Na + excretion, thereby decreasing circulating blood volume and restoring arterial pressure. The coupling between arterial pressure and Na + excretion was found to occur in the setting of preserved cortical autoregulation (i.e., in the absence of changes in total RBF, GFR, or filtered load of Na + ). This has led to the suggestion that the pressure natriuresis mechanism is triggered by changes in medullary circulation. Laser Doppler flowmetry and servo-null measurements of capillary pressure in volume-expanded rats have revealed that papillary blood flow is directly related to RPP over a wide range of pressures studied.
As mentioned earlier, an increase in medullary plasma flow might lead to medullary washout with a consequent reduction in the driving force for Na + reabsorption in the ascending loop of Henle, particularly in the deep nephrons. In addition, the increase in medullary perfusion may be associated with a rise in P i . In fact, increasing P i by ECFV expansion by infusion of renal vasodilatory agents, long-term mineralocorticoid escape, or hilar lymph ligation has resulted in a significant increase in Na + excretion. Moreover, prevention of the increase in P i by removal of the renal capsule significantly attenuates, but does not completely block, the natriuretic response to elevations in RPP. Thus, as depicted in Figure 15.6 , elevation in RPP is associated with an increase in medullary plasma flow and increased vasa recta capillary pressure, which results in an increase in medullary P i . This increase of P i is thought to be transmitted to the renal cortex in the encapsulated kidneys and to provide a signal that inhibits Na + reabsorption along the nephron. In that regard, the renal medulla may be viewed as a sensor that can detect changes in RPP and initiate the pressure natriuresis mechanism.

To explain how changes in systemic blood pressure are transmitted to the medulla in the presence of efficient RBF and GFR autoregulation, it has been suggested that shunt pathways connect preglomerular vessels of juxtamedullary nephrons directly to the postglomerular capillaries of the vasa recta. Alternatively, autoregulation of RBF might lead to increased shear stress in the preglomerular vasculature, triggering the release of nitric oxide and perhaps cytochrome P450 products of arachidonic acid metabolism (see later), thereby driving the cascade of events that inhibit Na + reabsorption. The mechanisms by which changes in P i and U Na excretion decrease tubular Na + reabsorption, as well as the nephron sites responding to the alterations in P i , have not been fully clarified. As noted earlier, it was postulated that elevations in P i may increase passive backleak or the paracellular pathway hydraulic conductivity, with a resultant increase in back flux of Na + through the paracellular pathways. However, the absolute changes in P i , in the range of 3 to 8 mm Hg in response to increments of about 50 to 90 mm Hg in RPP, are probably not sufficient to account for the decrease in tubular Na + reabsorption, even in the proximal tubule, the nephron segment with the highest transepithelial hydraulic conductivity. Nevertheless, considerable evidence from micropuncture studies has indicated that pressure natriuresis is associated with significant reduction in proximal fluid reabsorption, particularly in deep nephrons, with enhanced delivery to the loop of Henle, inhibition of sodium reabsorption in the thin descending limb and reduced blood flow in the vasa recta.
Pressure-induced changes in tubular reabsorption may also occur in more distal parts of the nephron, such as the ascending loop of Henle, distal nephron, and collecting duct. Therefore, elevations in RPP can affect tubular Na + reabsorption by proximal and distal mechanisms. The finding that small changes in P i are associated with significant alterations in tubular Na + reabsorption has led to the hypothesis that the changes in P i may be amplified by various hemodynamic, hormonal, and paracrine factors. Specifically, the phenomenon of pressure natriuresis is demonstrable, particularly in states of volume expansion and renal vasodilation, and is significantly attenuated in states of volume depletion. Among a variety of hormonal and paracrine systems that have been documented to play a role in modulating pressure natriuresis, changes in the activity of the RAAS and local production of prostaglandins within the kidneys have received considerable attention. Removal of the influence of Ang II by ACE inhibitors or Ang II type 1 receptor antagonists potentiates the pressure natriuretic response, and inhibitors of cyclooxygenase attenuate it. Moreover, blockade of the Ang II type 2 receptor allows the same amount of Na + to be excreted at lower arterial pressure. Of importance, however, is that pharmacologic blockade of these systems only attenuates but does not completely eliminate the pressure natriuresis response, which indicates that they act as modulators and not as mediators of the phenomenon.
The importance of endothelium-derived factors in the regulation of renal circulatory and excretory function has been recognized. Studies have suggested that endothelium-derived nitric oxide and P450 eicosanoids play a role in the mechanism of pressure natriuresis. Nitric oxide, generated in large amounts in the renal medulla, appears to play a critical role in the regulation of medullary blood flow and Na + excretion. Several studies have shown that inhibition of intrarenal nitric oxide production can reduce Na + excretion and markedly suppress the pressure natriuretic response, whereas administration of a nitric oxide precursor improves transmission of perfusion pressure into the renal interstitium and normalizes the defect in the pressure natriuresis response in Dahl salt-sensitive rats. Similarly, a positive correlation between urinary excretion of nitrites and nitrates (metabolites of nitric oxide) and changes in renal arterial pressure or U Na excretion were observed both in dogs and in rats. Hydrogen peroxide (H 2 O 2 ) has also been invoked in the mediation of RPP-induced changes in outer medullary blood flow and natriuresis. The response appears to be localized to the medullary thick ascending limb of Henle, in contrast to the nitric oxide effect, which occurs in the vasa recta. Other factors involved in the regulation of medullary blood flow include superoxide and heme oxygenase, both of which are released in the renal medulla in response to increased RPP.
The cytochrome P450 eicosanoids, particularly 20-HETE, are additional endothelium-derived factors that may participate in the mechanism of pressure natriuresis. These agents play an important role in the regulation of renal Na + transport and of renal and systemic hemodynamics. These observations support the hypothesis that alterations in the production of renal nitric oxide, reactive oxygen species, and eicosanoids may be involved in mediation of the pressure-induced natriuretic response.
It is tempting to speculate that acute elevations in RPP in the autoregulatory range result in increased blood flow velocity and shear stress, leading to increased endothelial release of nitric oxide and reactive oxygen species. Enhanced renal production of these molecules may increase U Na excretion by acting directly on tubular Na + reabsorption or through its vasodilatory effect on renal vasculature. ATP is another paracrine factor involved in pressure natriuresis. ATP is an important regulator of renal salt and water homeostasis. ATP release appears to be mediated by connexin 30 inasmuch as release in response to increased tubular flow or hypotonicity was abolished in connexin 30–deficient mice. Moreover, increased arterial pressure, induced by ligation of the distal aorta, led to diuresis and natriuresis in normal mice, but the response was attenuated in connexin 30 knockout mice. These data imply that mechanosensitive connexin 30 hemichannels play an integral role in pressure natriuresis by releasing ATP into the tubular fluid, thereby inhibiting salt and water reabsorption. Finally, Magyar and colleagues have reported that in response to an increase in RPP, the apical Na + /H + exchanger in the proximal tubules may be redistributed out of the brush border into intracellular compartments. Concomitantly, basolateral Na + -K + -ATPase activity decreased significantly. The mechanisms of these cellular events have not been fully elucidated, but they may be related directly to changes in P i or to changes in the intrarenal paracrine agents described previously.
A major assumption of the pressure natriuresis theory is that changes in systemic and RPP mediate the natriuretic response by the kidneys. As noted in comprehensive reviews, acute regulatory changes in renal salt excretion may occur without measurable elevation in arterial blood pressure. Of interest is that in many of these studies, the natriuresis was accompanied by a decrease in the activity of the RAAS, without changes in plasma ANP levels. Thus, whereas increases in arterial blood pressure can drive renal Na + excretion, other so-called pressure-independent control mechanisms must also operate to mediate the volume natriuresis.
Neural Mechanisms: Renal Nerves and Sympathetic Nervous System
Extensive autonomic innervation of the kidneys makes an important contribution to the physiologic regulation of all aspects of renal function. Sympathetic nerves, predominantly adrenergic, have been observed at all segments of the renal vasculature and tubule. Adrenergic nerve endings reach vascular smooth muscle cells and mesangial cells, cells of the juxtaglomerular apparatus, and all segments of the tubule—proximal, loop of Henle, and distal. Only the basolateral membrane separates the nerve endings from the tubular cells. Initial studies have determined that the greatest innervation is found in the renal vasculature, mostly at the level of the afferent arterioles, followed by the efferent arterioles and outer medullary descending vasa recta. However, high-density tubular innervation was found in the ascending limb of the loop of Henle, and the lowest density was observed in the collecting duct, inner medullary vascular elements, and papilla. The magnitude of the tubular response to renal nerve activation may thus be proportional to the differential density of innervation. In accordance with these anatomic observations, stimulation of the renal nerve results in vasoconstriction of afferent and efferent arterioles mediated by the activation of postjunctional α 1 -adrenoreceptors.
The presence of high-affinity adrenergic receptors in the nephron is also indicative of a significant role of the renal nerves in tubular function. The α 1 -adrenergic receptors and most of the α 2 -adrenergic receptors are localized in the basolateral membranes of the proximal tubule. In the rat, β-adrenoreceptors have been found in the corticalthick ascending limb of Henle and are subtyped as β 1 -adrenoceptors. The predominant neurotransmitters in renal sympathetic nerves are noradrenaline and, to a lesser extent, dopamine and acetylcholine. There is abundant evidence that changes in the activity of the renal sympathetic nerve play an important role in controlling body fluid homeostasis and blood pressure. Renal sympathetic nerve activity can influence renal function and Na + excretion through several mechanisms: (1) changes in renal and glomerular hemodynamics; (2) effect on renin release from juxtaglomerular cells, with increased formation of Ang II; and (3) direct effect on renal tubular fluid and electrolyte reabsorption. Graded direct electrical stimulation of renal nerves produces frequency-dependent changes in RBF and GFR, reabsorption of renal tubular Na + and water, and secretion of renin. The lowest frequency (0.5 to 1.0 Hz) stimulates renin secretion, and frequencies of 1.0 to 2.5 Hz increase renal tubule Na + and water reabsorption. Increasing the frequency of stimulation to 2.5 Hz and higher results in decreased RBF and GFR.
The decrease in SNGFR in response to enhanced renal nerve activity has been attributed to a combination of increases in afferent and efferent glomerular resistance, as well as decreases in glomerular capillary hydrostatic pressure (ΔP) and glomerular ultrafiltration coefficient (Kf). In Munich-Wistar rats, micropuncture experiments before and after renal nerve stimulation at different frequencies revealed that the effector loci for vasomotor control by renal nerves were in the afferent and efferent arteriole. In addition, although urine flow and Na + excretion declined with renal nerve stimulation, there was no change in absolute proximal fluid reabsorption rate, which suggests that reabsorption is increased in the more distal segments of the nephron.
Studies of the response of the kidneys to reflex activation of renal nerves have also indicated that the SNS has a role in regulating renal hemodynamic function and Na + excretion. In rats receiving diets with different Na + levels, DiBona and Kopp measured renal nerve activity in response to isotonic saline volume expansion and furosemide-induced volume contraction. A low-Na + diet resulted in a reduction in right atrial pressure and an increase in renal nerve activity. The magnitude of the increase in renal nerve activity was approximately 20% for each 1-mm Hg fall in atrial pressure. Conversely, the high-Na + diet resulted in increased right atrial pressure and a reduction in renal nerve activity. Other studies in conscious animals in which researchers used maneuvers such as HWI and left atrial balloon inflation have yielded evidence of the importance of reflex regulation of renal nerve activity.
Collectively, these studies demonstrated the reciprocal relationship between ECFV and renal nerve activity, which is consistent with the role of central cardiopulmonary mechanoreceptors governing renal nerve activity. Moreover, the contribution of efferent renal nerve activity is of greater significance during conditions of dietary Na + restriction, when the need for renal Na + conservation is maximal. When this linkage between the renal SNS and excretory renal function is defective, abnormalities in the regulation of ECF volume and blood pressure may develop.
Several studies in which the response of denervated kidneys to various physiologic maneuvers was examined also yielded evidence that renal nerves play a role in regulating renal hemodynamic function and Na + excretion. Early studies showed that acute denervation of the kidneys is associated with increased urine flow and Na + excretion. Micropuncture techniques showed that in euvolemic animals, elimination of renal innervation does not alter any of the determinants of SNGFR, indicating that renal nerves contribute little to the vasomotor tone of normal animals under baseline physiologic conditions. However, absolute proximal reabsorption was significantly reduced in the absence of changes in peritubular capillary oncotic pressure, hydraulic pressure, and renal interstitial pressure. The decrease in tubular electrolyte and water reabsorption after renal denervation was also observed in the loop of Henle and segments of the distal nephron.
In another micropuncture study in control rats and in rats with experimentally induced heart failure or acute volume depletion, denervation resulted in diuresis and natriuresis in normal rats but failed to alter any of the parameters of renal cortical microcirculation. In rats with heart failure, in contrast, denervation caused both an amelioration of renal vasoconstriction by decreasing afferent and efferent arteriolar resistance and, again, natriuresis. This study indicated that in situations in which efferent neural tone is heightened above baseline level, renal nerve activity may profoundly influence renal circulatory dynamics. However, although the basal level of renal nerve activity in normal rats or conscious animals is apparently insufficient to influence renal hemodynamics, it is sufficient to exert a tonic stimulation on renal tubular epithelial Na + reabsorption and renin release. Classic studies, in which guanethidine was given to achieve autonomic blockade or in patients with idiopathic autonomic insufficiency, have revealed that intact adrenergic innervation is required for the normal renal adaptive response to dietary Na + restriction.
More direct examination of efferent RSNA in humans has been made possible by the measurement of renal norepinephrine spillover to elucidate the kinetics of norepinephrine release. Friberg and associates have determined that in normal subjects, a low-Na + diet results in a fall in U Na excretion and an increase in norepinephrine spillover, with no change in cardiac norepinephrine uptake; these findings support the concept of a true increase of efferent renal nerve activity secondary to Na + restriction. Similarly, low-dose infusion of norepinephrine to normal salt-replete volunteers resulted in a physiologic plasma increment of this neurotransmitter in association with antinatriuresis. This reduction in Na + excretion occurred without any change in GFR but was associated with a significant decline in lithium (Li + ) clearance, an indication of enhanced proximal tubule reabsorption.
The cellular mechanisms mediating the tubular actions of norepinephrine appear to include stimulation of Na + – K + – ATPase activity and Na + /H + exchange in proximal tubular epithelial cells. It is assumed that α 1 -adrenoreceptor stimulation, mediated by phospholipase C, causes an increase in intracellular Ca 2+ that activates the Ca 2+ calmodulin-dependent calcineurin phosphatase. Calcineurin converts Na + – K + – ATPase from its inactive phosphorylated form to its active dephosphorylated form. The stimulatory effect of renal nerves on Na + /H + exchange is mediated through stimulation of the α 2 -adrenoreceptor.
In addition to the direct action of Na + on epithelial cell transport and renal hemodynamics, interactions of renal nerve input with other effector mechanisms may contribute to the regulation of renal handling of Na + . Efferent sympathetic nerve activity influences the rate of renin secretion in the kidneys by a variety of mechanisms, either directly or by interacting with the macula densa and vascular baroreceptor mechanisms for renin secretion. The increase in renin secretion is mediated primarily by direct stimulation of β 1 -adrenergic receptors located on juxtaglomerular granular cells. Sympathetic activation of renin release is augmented during RPP reduction. Results of studies in the isolated perfused rat kidney have suggested that intrarenal generation of Ang II has an important prejunctional action on renal sympathetic nerve terminals to facilitate norepinephrine release during renal nerve stimulation. However, the physiologic significance of this facilitatory interaction on tubular Na + reabsorption remains controversial. Thus, administration of an ACE inhibitor or an angiotensin receptor blocker (ARB) attenuates the antinatriuretic response to electrical renal nerve stimulation in anesthetized rats. In contrast, when nonhypotensive hemorrhage was used to produce a reflex increase in RSNA in conscious dogs, the associated antinatriuresis was unaffected by ACE inhibition or Ang II receptor blockade.
Sympathetic activity is also a stimulus for the production and release of renal prostaglandins, coupled in series to the adrenergic-mediated renal vasoconstriction. Evidence indicates that renal vasodilatory prostaglandins attenuate the renal hemodynamic vasoconstrictive response to activation of the renal adrenergic system in vivo and on isolated renal arterioles. In Munich-Wistar rats, results of micropuncture experiments have indicated that the primary factor responsible for the reduction in the glomerular Kf during renal nerve stimulation may be Ang II rather than norepinephrine, and that endogenously produced prostaglandins neutralize the vasoconstrictive effects of renal nerve stimulation at an intraglomerular locus rather than at the arteriolar level.
Another interaction examined is that between the renal SNS and AVP. Studies in conscious animals have shown that AVP exerts a dose-related effect on the arterial baroreflex. Low doses of AVP might have sensitized the central baroreflex neurons to afferent input, whereas higher doses caused direct excitations of these neurons, which resulted in a reduction in sympathetic outflow. In addition, AVP suppresses renal sympathetic outflow, and this response depends on the number of afferent inputs from baroreceptors. Conversely, renal nerve stimulation resulted in elevations of plasma AVP levels and arterial pressure in conscious, baroreceptor-intact Wistar rats. Many studies have demonstrated in normal and pathologic situations that increased RSNA can antagonize the natriuretic/diuretic response to ANP and that removal of the influence of sympathetic activity enhances the natriuretic action of the peptide. Conversely, renal denervation in Wistar rats increased ANP receptors and cGMP generation in glomeruli, which resulted in an increase in Kf after ANP infusion.
In summary, renal sympathetic nerves can regulate U Na and water excretion by changing renal vascular resistance, by influencing renin release from the juxtaglomerular granular cells, and through a direct effect on tubular epithelial cells ( Figure 15.7 ). These effects may be modulated through interactions with other hormonal systems, including ANP, prostaglandins, and AVP.

Humoral Mechanisms
Renin-Angiotensin-Aldosterone System.
The RAAS plays a central role in the regulation of ECF volume, Na + homeostasis, and cardiac function. The system is activated in situations that compromise hemodynamic stability, such as blood loss, reduced EABV, low Na + intake, hypotension, and increase in sympathetic nerve activity. The RAAS is comprised of a coordinated hormonal cascade whose synthesis is initiated by the release of renin from the juxtaglomerular apparatus in response to reduced renal perfusion or decrease in arterial pressure. Messenger RNA for renin exists in juxtaglomerular cells and in renal tubular cells. Renin acts on its circulating substrate, angiotensinogen, which is produced and secreted mainly by the liver, but also by the kidneys. ACE 1 (ACE1), which cleaves Ang I to Ang II, exists in large amounts in the microvasculature of the lungs but also on endothelial cells of other vascular beds and cell membranes of the brush border of the proximal nephron, heart, and brain. Ang II is the principal effector of the RAAS, although other smaller metabolic products of Ang II also have biologic activities. Nonrenin (cathepsin G, plasminogen-activating factor, tonin) and non-ACE pathways (chymase, cathepsin G) also exist in these tissues and may contribute to tissue Ang II synthesis.
In addition to its important function as a circulating hormone, Ang II produced locally acts as a paracrine agent in an organ-specific mode. In that regard, the properties of Ang II as a growth-promoting agent in the cardiovascular system and kidneys have been increasingly appreciated. For example, local generation of Ang II in the kidneys results in higher intrarenal levels of this peptide in proximal tubular fluid, interstitial fluid, and renal medulla than in the circulation. The epithelial cells of the proximal nephron are an important source for the in situ generation of Ang II because these cells show abundant expression of the messenger RNA for angiotensinogen. Furthermore, Ang II is secreted from tubular epithelial cells into the lumen of the proximal nephron. This may account for the fact that concentrations of Ang II are approximately 1000 times higher in the proximal tubular fluid than in the plasma. Moreover, the mechanisms regulating intrarenal levels of Ang II appear to be dissociated from those controlling the systemic concentrations of the peptide.
The biologic actions of Ang II are mediated through activation of at least two receptor subtypes, AT 1 and AT 2 , encoded by different genes residing on different chromosomes. Both receptors are G protein–coupled, seven-transmembrane polypeptides containing approximately 360 amino acids. In the adult organism, the AT 1 receptor mediates most of the biologic activities of Ang II, whereas the AT 2 receptor appears to have a vasodilatory and antiproliferative effect. AT 1 is expressed in the vascular poles of glomeruli, juxtaglomerular apparatus, and mesangial cells, whereas the quantitatively lower expression of AT 2 is confined to renal arteries and tubular structures. In addition to their functional distinction, the two receptor types use different downstream pathways. Stimulation of the AT 1 receptor activates phospholipases A 2 , C, and D, which results in increased cytosolic Ca 2+ and inositol triphosphate and inhibition of adenylate cyclase. In contrast, activation of the AT 2 receptor results in increases in nitric oxide and bradykinin levels, which lead to elevation in cGMP concentrations and to vasodilation.
In addition to being an important source of several components of the RAAS, the kidney acts as a major target organ for the principal hormonal mediators of this cascade, Ang II and aldosterone. The direct effect of Ang II is mediated via AT 1 receptors that exert multiple direct intrarenal influences, including renal vasoconstriction, stimulation of tubular epithelial Na + reabsorption, augmentation of TGF sensitivity, modulation of pressure natriuresis, and stimulation of mitogenic pathways. Moreover, exogenous infusion of Ang II, which results in relatively low circulating levels of Ang II (picomolar range), is highly effective in modulating renal hemodynamic and tubular function, in comparison with the 10- to 100-fold higher concentrations required for its extrarenal effects. Thus, the kidneys appear to be uniquely sensitive to the actions of Ang II.
Furthermore, the synergistic interactions that exist between the renal vascular and tubular actions of Ang II significantly amplify the influence of Ang II on Na + excretion. Of the direct renal actions of Ang II, its effect on renal hemodynamics appears to be of critical importance. Ang II elicits a dose-dependent decrease in RBF but slightly augments GFR as a result of its preferential vasoconstrictive effect on the efferent arteriole, and therefore increases the filtration fraction. In turn, the increased filtration fraction may further modulate peritubular Starling forces, possibly by decreasing hydraulic pressure and increasing colloid osmotic pressure in the interstitium. These peritubular changes eventually lead to enhanced reabsorption of proximal Na + and fluid. Of importance, however, is that changes in preglomerular resistance have also been described during Ang II infusion or blockade. These may be secondary to changes in systemic arterial pressure (myogenic reflex) or to increased sensitivity of TGF because Ang II does not alter preglomerular resistance when RPP is clamped or adjustments in TGF are prevented.
In addition, Ang II may affect GFR by reducing Kf, thereby altering the filtered load of Na + . This effect is believed to reflect the action of the hormone on mesangial cell contractility and increasing permeability to macromolecules. Finally, Ang II may also influence Na + excretion through its action on the medullary circulation. Because Ang II receptors are highly abundant in the renal medulla, this peptide may contribute significantly to the regulation of medullary blood flow. In fact, use of fiberoptic probes has revealed that Ang II usually reduces cortical blood flow and medullary blood flow and decreases Na + and water excretion. As noted earlier, changes in medullary blood flow may affect medullary tonicity, which determines the magnitude of passive salt reabsorption in the loop of Henle, and may also modulate pressure natriuresis through alterations in renal interstitial pressure.
The other well-characterized renal effect of Ang II is a direct action on proximal tubular epithelial transport. Infusions of Ang II to achieve systemic concentrations of 10 -12 to 10 -11 mol markedly stimulated Na + and water transport, independently of changes in renal or systemic hemodynamics. Ang II exerts a dose-dependent biphasic effect on proximal Na + reabsorption. Peritubular capillary infusion with solutions containing low concentrations of Ang II (10 -12 to 10 -10 mol) stimulated the proximal Na + reabsorption rate, whereas perfusion at higher concentrations of Ang II (>10 -7 mol) inhibited the proximal Na + reabsorption rate. Addition of the AT 1 receptor antagonist losartan or the ACE inhibitor enalaprilat directly into the luminal fluid of the proximal nephron resulted in a significant decrease in proximal fluid reabsorption, which is indicative of tonic regulation of proximal tubule transport by endogenous Ang II.
The specific mechanisms by which Ang II influences proximal tubule transport include increases in reabsorption of Na + and HCO 3 − by stimulation of the apical Na + -H + antiporter, Na + /H + -exchanger isoform 3 (NHE3), basolateral Na + -3HCO 3 − symporter, and Na + -K + -ATPase. Thus, Ang II can affect NaCl absorption by two mechanisms:
- 1.
Activation of NHE3 can directly increase NaCl absorption.
- 2.
Conditions that increase the rate of NaHCO 3 absorption can stimulate passive NaCl absorption by increasing the concentration gradient for passive Cl − diffusion.
In the early and late portions of the distal tubule, as well as the connecting tubule, Ang II regulates Na + and HCO 3 − reabsorption by stimulating NHE3 and the amiloride-sensitive Na + channel. Two additional mechanisms may amplify the antinatriuretic effects of Ang II that are mediated by the direct actions of the peptide on renal hemodynamics and tubular transport. The first concerns the increased sensitivity of the TGF mechanism in the presence of Ang II, and the second is related to the effect of Ang II on pressure natriuresis. The decrease in distal delivery produced by the action of Ang II on renal hemodynamics and proximal fluid reabsorption could elicit afferent arteriolar vasodilation by means of the TGF mechanism, which, in turn, could antagonize the Ang II–mediated increase in proximal reabsorption. This effect, however, is minimized because Ang II increases the responsiveness of the TGF mechanism, thus maintaining GFR at a lower delivery rate to the macula densa. The second mechanism by which the antinatriuretic effects of Ang II may be amplified is blunting of the pressure natriuresis mechanism so that higher pressures are needed to induce a given amount of Na + excretion. This shift to the right in the pressure natriuresis curve may be viewed as an important Na + -conserving mechanism in situations of elevated arterial pressure.
The use of ACE inhibitors and highly specific ARBs has provided additional insight into the mechanisms of action of Ang II in the kidneys; the findings have suggested that most of the known intrarenal actions of Ang II, particularly regulation of renal hemodynamics and proximal tubule reabsorption of Na + and HCO 3 − , are mediated by the AT 1 receptor. However, functional studies have shown that some of the actions of Ang II at the renal level are mediated by AT 2 receptors. The AT 2 receptor subtype plays a counterregulatory protective role against the AT 1 receptor–mediated antinatriuretic and pressor actions of Ang II. The accepted concept that Ang I was converted solely to Ang II was revised through the demonstration that Ang I is also a substrate for the formation of angiotensin-(1-7). Moreover, a recently discovered homolog of ACE, ACE type 2 (ACE2), is responsible for the formation of angiotensin-(1-7) from Ang II and for the conversion of Ang I to angiotensin-(1-9), which may be converted to angiotensin-(1-7) by ACE.
Angiotensin-(1-7), through its G protein–coupled receptor, Mas, may play a significant role as a regulator of cardiovascular and renal function by opposing the effects of Ang II; it does this through vasodilation, diuresis, and an antihypertrophic action. Thus, the RAAS can currently be envisioned as a dual-function system in which the vasoconstrictor/proliferative or vasodilator/antiproliferative actions are driven primarily by the ACE/ACE2 balance. According to this model, an increased ACE/ACE2 activity ratio leads to increased generation of Ang II and increased catabolism of angiotensin-(1-7), which is conducive to vasoconstriction; conversely, a decreased ACE/ACE2 ratio reduces Ang II and increases angiotensin-(1-7) levels, facilitating vasodilation. The additional effect of angiotensin-(1-7)/Mas to antagonize the actions of Ang II directly adds a further level of counterregulation.
The final component of the RAAS, aldosterone, is produced via Ang II stimulation of the adrenal cortex and also plays an important physiologic role in the maintenance of ECFV and Na + homeostasis. The primary sites of aldosterone action are the principal cells of the cortical collecting tubule and convoluted distal tubule, in which the hormone promotes the reabsorption of Na + and the secretion of K + and protons. Aldosterone may also enhance electrogenic Na + transport, but not K + secretion, in the IMCD and has recently been shown to act also in the proximal tubule. Aldosterone exerts its effects on ionic transport by increasing the number of open Na + and K + channels in the luminal membrane and the activity of Na + -K + -ATPase in the basolateral membrane. The effect of aldosterone on Na + permeability appears to be the primary event because blockade of the ENaC with amiloride prevents the initial increase in Na + permeability and Na + -K + -ATPase activity. This effect on Na + permeability is mediated by changes in intracellular Ca 2+ levels, intracellular pH, trafficking via protein kinase D1-phosphatidylinositol 4-kinaseIIIβ trans Golgi signaling, and methylation of channel proteins, thus increasing the mean open probability of ENaC. However, the long-term effect of aldosterone on Na + -K + -ATPase activity involves de novo protein synthesis, which is regulated at the transcriptional level by serum- and glucocorticoid-induced kinase-1.
It has become clear that aldosterone specifically regulates the α-subunit of ENaC and that changes in expression of a variety of genes are important intermediates in this process. Using microarray analysis in a mouse IMCD line, Gumz and associates examined the acute transcriptional effects of aldosterone. They found that the most prominent transcript was period homolog 1 (Per1), an important component of the circadian clock, and that disruption of the Per1 gene leads to attenuated expression of messenger RNA encoding for the α-subunit of ENaC and increased U Na excretion. They also noted that messenger RNA encoded by the α-subunit of ENaC was expressed in an apparent circadian pattern that was dramatically altered in mice lacking functional Per1 genes. These results imply that the circadian clock has a previously unknown role in the control of Na + balance. Perhaps of more importance is that it provides molecular insight into how the circadian cycle directly affects Na + homeostasis.
The Na + -retaining effect of aldosterone in the collecting tubule induces an increase in the transepithelial potential difference, which is conducive to K + excretion. In terms of overall body fluid homeostasis, the actions of aldosterone in the defense of ECF result from the net loss of an osmotically active particle confined primarily to the intracellular compartment (K + ) and its replacement with a corresponding particle confined primarily to the ECF (Na + ). The effect of a given circulating level of aldosterone on overall Na + excretion depends on the volume of filtrate reaching the collecting duct and the composition of luminal and intracellular fluids. As noted earlier, this delivery of filtrate is, in turn, determined by other effector mechanisms (Ang II, sympathetic nerve activity, and peritubular physical forces) acting at more proximal nephron sites.
It is not surprising that Na + balance can be regulated over a wide range of intake, even in subjects without adrenal glands and despite fixed low or high supplemental doses of mineralocorticoids. Under these circumstances, other effector mechanisms predominate in controlling urinary Na + excretion, although often in a setting of altered ECF volume or K + concentration. In this regard, how renal Na + reabsorption and K + excretion are coordinately regulated by aldosterone has long been a puzzle. In states of EABV depletion, aldosterone release stimulated by Ang II induces maximal Na + reabsorption without significantly affecting plasma K + levels. Conversely, hyperkalemia-induced aldosterone secretion stimulates maximum K + excretion without major effects on renal Na + handling.
Elegant studies on the intracellular signaling pathways involved in renal Na + and K + transport have shed light on this puzzle. The key elements in this transport regulation are the Ste20/SPS1-related proline/alanine-rich kinase (SPAK), oxidative stress-related kinase (OSR1) the with-no-lysine kinases (WNKs) and their effectors, the thiazide-sensitive NaCl cotransporter, and the K + secretory channel ROMK. According to the proposed model, when EABV is reduced or dietary salt intake is low, Ang II, mediated by the AT 1 receptor, leads to phosphorylation of WNK4, which stimulates phosphorylation of SPAK and OSR1. In turn, SPAK and OSR1 phosphorylate the NaCl cotransporter, inducing Na + transport and conservation. Simultaneous phosphorylation of the full-length isoform of WNK1, WNK1-L, causes endocytosis of the ROMK channel, thereby enabling K + conservation, despite high aldosterone levels. In contrast, in the presence of hyperkalemia or low dietary salt, Ang II levels are low so that WNK4 cannot be activated, SPAK, OSR1 and the NaCl cotransporter are not phosphorylated, and NaCl cotransporter trafficking to the apical membrane is inhibited. At the same time, K + -induced kidney-specific WNK1 leads to suppression of WNK1-L, which allows ROMK trafficking to the apical membrane and maximal K + secretion. For further details, the reader is referred to an excellent recent review.
In terms of blood pressure maintenance, systemic vasoconstriction—another major extrarenal action of Ang II—may be considered the appropriate response to perceived ECF volume contraction. As mentioned previously, higher concentrations of Ang II are needed to elicit this response than those that govern the renal antinatriuretic actions of Ang II, a situation analogous to the discrepancy between antidiuretic and pressor actions of vasopressin. Transition from an antinatriuretic to a natriuretic action of Ang II at high infusion rates can be attributed almost entirely to a concomitant rise in blood pressure. There is now clear evidence that in addition to the adrenal glomerulosa, aldosterone may also be produced by the heart and vasculature. It exerts powerful effects on blood vessels, independently of actions that can be attributed to the blood pressure rise through regulation of salt and water balance. As observed with Ang II, aldosterone also possesses significant mitogenic and fibrogenic properties. It directly increases the expression and production of transforming growth factor-β and thus is involved in the development of glomerulosclerosis, hypertension, and cardiac injury/hypertrophy.
In summary, Ang II, the principal effector of the RAAS, regulates extracellular volume and renal Na + excretion through intrarenal and extrarenal mechanisms. The intrarenal hemodynamic and tubular actions of the peptide and its main extrarenal actions (systemic vasoconstriction and aldosterone release) act in concert to adjust U Na excretion under a variety of circumstances associated with alterations in ECF volume. Many of these mechanisms are synergistic and tend to amplify the overall influence of the RAAS. However, additional counterregulatory mechanisms, induced directly or indirectly by Ang II, provide a buffer against the unopposed actions of the primary components of the RAAS.
Vasopressin.
AVP is a nonapeptide (nine–amino acid) hormone, synthesized in the brain, that is secreted from the posterior pituitary gland into the circulation in response to an increase in plasma osmolality (through osmoreceptor stimulation) or a decrease in EABV and blood pressure (through baroreceptor stimulation). Thus, AVP plays a major role in the regulation of water balance and the support of blood pressure and EABV. AVP exerts its biologic actions through at least three different G protein–coupled receptors. Two of these receptors, V 1A and V 2 , are abundantly expressed in the cardiovascular system and the kidneys; V 1B receptors are expressed on the surfaces of corticotrophic cells of the anterior pituitary gland, in the pancreas, and in the adrenal medulla. V 1A and V 2 receptors mediate the two main biologic actions of the hormone, vasoconstriction and increased water reabsorption by the kidneys, respectively. (V 2 receptor–mediated effects on hemostasis are discussed in Chapter 29 .) The V 1A and V 1B receptors operate through the phosphoinositide signaling pathway, causing release of intracellular Ca 2+ . The V 1A receptor, found in vascular smooth muscle cells, hepatocytes, and platelets, mediates vasoconstriction, glycogenolysis, and platelet aggregation, respectively. The V 2 receptor, found mainly in the renal collecting duct epithelial cells, is linked to the adenylate cyclase pathway, and cAMP is used as its second messenger.
Under physiologic conditions, AVP functions primarily to regulate water content in the body by adjusting water reabsorption in the collecting duct according to plasma tonicity. A change in plasma tonicity by as little as 1% causes a parallel change in AVP release. This change, in turn, alters the water permeability of the collecting duct. The antidiuretic action of AVP results from complex effects of this hormone on principal cells of the collecting duct. First, AVP provokes the insertion of aquaporin-2 (AQP2) water channels into the luminal membrane (short-term response) and increases the synthesis of AQP2 messenger RNA and protein ; both responses increase water permeability along the collecting duct. This is considered in detail in Chapter 11 . In brief, activation of V 2 receptors localized to the basolateral membrane of the principal cells increases cytosolic cAMP, which stimulates the activity of protein kinase A. The latter triggers a series of phosphorylation events that promotes the translocation of AQP2 from intracellular stores to the apical membrane, which allows the reabsorption of water from the lumen to the cells. The water then exits the cell to the hypertonic interstitium via aquaporin-3 and aquaporin-4, localized at the basolateral membrane.
The second complex effect of AVP on the collecting duct is to increase the permeability of the IMCD to urea through activation of the urea transporter UT-A1, which enables the accumulation of urea in the interstitium; there, along with Na + , it contributes to the hypertonicity of the medullary interstitium, which is a prerequisite for maximum urine concentration and water reabsorption. AVP exerts several effects on Na + handling at different segments of the nephron, in which it increases Na + reabsorption through activation of ENaC, mainly in the cortical and outer medullary collecting ducts.
In addition, AVP may influence renal hemodynamics and reduce RBF, especially to the inner medulla. The latter effect is mediated by the V 1A receptor and may be modulated by the local release of nitric oxide and prostaglandins. At higher concentrations, AVP may also decrease total RBF and GFR as part of the generalized vasoconstriction induced by the peptide.
The role of the V 1A receptor in the kidneys has been further elaborated. In V 1A receptor–deficient (V 1A R −/− ) mice, plasma volume and blood pressure were decreased. Also, urine volume of V 1A R −/− mice was greater than that of wild-type mice, particularly after a water load; however, GFR, U Na excretion, AVP-dependent cAMP generation, levels of V 2 receptor, and AQP2 expression in the kidneys were lower, which indicates that the diminishment of GFR and the V 2 receptor–AQP2 system led to impaired urinary concentration in V 1A R −/− mice. This result is interesting because classic models implicate the V 2 receptors in water handling by the nephron. Moreover, plasma renin and Ang II levels were decreased, as was renin expression in granule cells. In addition, the expression of renin stimulators such as nNOS and cyclo-oxygenase-2 (COX-2) in macula densa cells, where V 1A R is specifically expressed, was decreased in V 1A R −/− mice. Aoyagi and colleagues have concluded that AVP regulates body fluid homeostasis and GFR through the V 1A R in macula densa cells by activating the RAAS and subsequently the V 2 receptor–AQP2 system. Recent work from the same group (Yasuoka and associates ) has confirmed the importance of the V 1A R for the expression of AQP2 in the collecting ducts during control conditions and dehydration.
A third receptor for AVP, V 3 , is found predominantly in the anterior pituitary gland and is involved in the regulation of adrenocorticotropic hormone (ACTH) release. In addition to its renal effects, AVP also regulates extrarenal vascular tone through the V 1A receptor. Stimulation of this receptor by AVP results in a potent arteriolar vasoconstriction in various vascular beds, with a significant increase in systemic vascular resistance. However, physiologic increases in AVP do not usually cause a significant increase in blood pressure because AVP also potentiates the sinoaortic baroreflexes that subsequently reduce heart rate and cardiac output. Nevertheless, at supraphysiologic concentrations of AVP, such as those that occur when EABV is severely compromised (e.g., in shock or heart failure), AVP plays an important role in supporting arterial pressure and maintaining adequate perfusion to vital organs such as the brain and myocardium. AVP also has a direct, V 1 receptor–mediated, inotropic effect in the isolated heart. In vivo, however, AVP has been reported to decrease myocardial function ; this effect is attributed to cardioinhibitory reflexes or coronary vasoconstriction induced by the peptide. Of more importance is that AVP has been shown to stimulate cardiomyocyte hypertrophy and protein synthesis in neonatal rat cardiomyocytes and in intact myocardium through a V 1 -dependent mechanism. These effects are very similar to those obtained with exposure of cardiomyocytes to Ang II or catecholamines, although not necessarily through the same cellular mechanisms. By this growth-promoting property, AVP may contribute to the induction of cardiac hypertrophy and remodeling in heart failure.
Until recently, uncertainty existed regarding the effect of AVP on natriuresis; some authors have found a natriuretic response with infusions, and others have found Na + retention. These variations may have resulted from differences between species or from acute changes in volume status. It is now clear that an increase in Na + reabsorption along the distal nephron (connecting tubule and collecting duct), mediated by activation of ENaC by vasopressin, makes an important contribution to maintenance of the axial corticomedullary osmotic gradient necessary for maximal water reabsorption. Thus, the renal action of vasopressin includes not only a direct decrease in free water excretion to dilute plasma, but also Na + reabsorption and, consequently, decreased Na + excretion via ENaC activated along the distal nephron. In terms of overall volume homeostasis, regardless of the effects of AVP on Na + excretion, the predominant influence of the hormone is indirectly through water accumulation or vasoconstriction. In fact, the vasoconstrictive V 1 receptor effect of AVP overrides the osmotically driven effect in the presence of an ECF volume deficit of 20% or more (see Chapter 10 , Chapter 11 , Chapter 16 ). Nevertheless, in this regard, potential hypertensive effects of AVP are buffered by a concomitant increase in baroreflex-mediated sympathoinhibition or by an increase in PGE 2 , which results in a blunting of vasoconstriction, and by a direct vasodepressor action of V 2 receptor activation.
Prostaglandins.
Prostaglandins (see also Chapter 14 ), or cyclo-oxygenase–derived prostanoids, possess complex and diverse regulatory functions in the kidneys, including hemodynamics, renin secretion, growth response, tubular transport processes, and immune response in health and disease ( Table 15.3 ). Currently, two known principal isoforms of cyclo-oxygenase (COX-1 and COX-2) catalyze the synthesis of prostaglandin H 2 (PGH 2 ) from arachidonic acid, released from membrane phospholipids. PGH 2 is then metabolized to the five major prostanoids—PGE 2 , prostaglandins I 2 , D 2 , and F 2 α (PGI 2 , PGD 2 , and PGF 2 α), and thromboxane A 2 (TXA 2 )—through specific synthases (see also Chapter 14 ). An additional splice variant of the COX-1 gene, COX-3, has been identified, but its function in humans remains unclear.
Agent | Target Structure | Mode of Action | Direct Consequences |
---|---|---|---|
PGE 2 , PGI 2 | Intrarenal arterioles | Vasodilation | Increased renal perfusion (more pronounced in inner cortical and medullary regions) |
PGI 2 | Glomeruli | Vasodilation | Increased filtration rate |
PGE 2 , PGI 2 | Efferent arterioles | Vasodilation | Increased Na + excretion through increased postglomerular perfusion |
PGE 2 , PGI 2 , PGF 2α | Distal tubules | Decreased transport | Increased Na + excretion, decreased maximum medullary hypertonicity |
PGE 2 , PGI 2 , PGF 2α | Distal tubules | Inhibition of cAMP synthesis | Interference with AVP action |
PGE 2 , PGI 2 | Juxtaglomerular apparatus | cAMP stimulation (?) | Increased renin release |
TxA 2 | Intrarenal arterioles | Vasoconstriction | Decreased renal perfusion |
Prostanoids are rapidly degraded, so their effect is localized strictly to their site of synthesis, which accounts for the predominance of their autocrine and paracrine modes of action. Each prostanoid has a specific cell surface G protein–coupled receptor, distinct for a given location, that determines the specific function of the prostaglandin in the given cell type. COX-1 is constitutively expressed and serves in a housekeeping role in many cell types; it is expressed abundantly and is highly immunoreactive in the kidneys, especially in the collecting duct but also in medullary interstitial, mesangial, and arteriolar endothelial cells of most species. In contrast, the expression of COX-2 is inducible and cell type–specific, and its renal expression is prominent in medullary interstitial cells, cortical cells of the thick ascending limb of Henle, and cells of the macula densa, in which expression is regulated in response to varying amounts of salt intake. Furthermore, the profile of sensitivity to pharmacologic inhibitors differs between the two isoforms. The principal prostanoid in the kidneys is PGE 2 ; others present are PGI 2 , PGF 2 , and TXA 2 . PGI 2 and PGE 2 are the main products in the cortex of normal kidneys, and PGE 2 predominates in the medulla. The metabolism of arachidonic acid by other pathways (e.g., lipoxygenase, epoxygenase) leads to products that are involved in crosstalk with cyclo-oxygenase. The major sites for prostaglandin production (and hence for local actions) are the renal arteries and arterioles and glomeruli in the cortex and interstitial cells in the medulla, with additional contributions from epithelial cells of the cortical and medullary collecting tubules.
The two major roles for prostaglandins in volume homeostasis are (1) their effect on RBF and GFR and (2) their effect on tubular handling of salt and water. Table 15.3 lists target structures, mode of action, and major biologic effects of the active renal prostanoids. PGI 2 and PGE 2 have predominantly vasodilating and natriuretic activities; they also modulate the action of AVP and tend to stimulate renin secretion. TXA 2 has been shown to cause vasoconstriction, although the importance of the physiologic effects of TXA 2 on the kidneys is still controversial. The end results of the stimulation of renal prostaglandin secretion in the kidneys are vasodilation, increased renal perfusion, natriuresis, and facilitation of water excretion.
The role of prostaglandins as vasodilators in the glomerular microcirculation is now well established. The cellular targets for vasoactive hormones in the glomerular microcirculation are vascular smooth muscle cells of the afferent and efferent arterioles and mesangial cells within the glomeruli. Action at these sites governs renal vascular resistance, glomerular function, and downstream microcirculatory function in peritubular capillaries and vasa recta. In vivo studies have shown that intrarenal infusions of PGE 2 and PGI 2 cause vasodilation and increased RBF. In agreement with these findings, in vitro experiments with isolated renal microvessels have shown that PGE 2 and prostaglandin E 1 (PGE 1 ) attenuate Ang II–induced afferent arteriolar vasoconstriction, and PGI 2 antagonizes Ang II–induced efferent arteriolar vasoconstriction. Similarly, PGE 2 has been shown to counteract Ang II–induced contraction of isolated glomeruli and glomerular mesangial cells in culture and, conversely, cyclo-oxygenase inhibition augments these contractile responses. An inhibitory counterregulatory role of prostaglandins with regard to renal nerve stimulation has also been demonstrated from micropuncture studies. Furthermore, in volume-contracted states, COX-2 expression and PGE 2 release in the macula densa and cortical thick ascending limb of Henle dramatically increase in response to decreased luminal Cl − delivery. In addition to its direct vasodilator effect on afferent arterioles, PGE 2 leads to increased renin release from the macula densa. The resulting rise in Ang II and consequent efferent arteriolar constriction also ensure maintenance of the GFR.
In the clinical situation, in volume-replete states, the renal vasoconstrictive influences of Ang II and norepinephrine are mitigated by their simultaneous stimulation of vasodilatory renal prostaglandins, so that RBF and GFR are maintained. However, in the setting of heightened vasoconstrictor input from the RAAS, SNS, and AVP, as occurs during states of EABV depletion, the vasorelaxant action of PGE 2 and PGI 2 is overwhelmed, with the concomitant risk for the development of acute kidney injury. Similarly, when this prostaglandin-mediated counterregulatory mechanism is suppressed by nonselective or COX-2–selective inhibitors, the unopposed actions of Ang II and norepinephrine can also lead to a rapid deterioration in renal function. Moreover, COX-2–derived prostanoids also promote natriuresis and stimulate renin secretion. Therefore, during states of volume depletion, low Na + intake, or the use of loop diuretics, COX-2 inhibitors (e.g., celecoxib), and the nonselective COX inhibitors diclofenac and naproxen, can cause Na + and K + retention, edema formation, heart failure, and hypertension.
In addition to the role of prostaglandins in modulating glomerular vasoreactivity in states of varying salt balance, prostaglandins also have direct effects on salt excretion. Clearly, the aforementioned vascular effects of prostaglandins can be expected to have secondary effects on tubular function through the various physical factors described earlier in this chapter. One particular consequence of prostaglandin-induced renal vasodilation may be medullary interstitial solute washout. Such a change in medullary interstitial composition could potentially account for the observed increase in U Na excretion with intrarenal infusion of PGE 2 . The natriuretic response to PGE 2 may also be attenuated by preventing an increase in renal interstitial hydraulic pressure, even in the presence of a persistent increase in RBF. In addition, in rats, the natriuresis usually accompanying direct expansion of renal interstitial volume can be significantly attenuated by inhibition of prostaglandin synthesis. These findings are consistent with the proposal that changes in prostaglandins have a significant effect on renal Na + excretion.
Results of micropuncture and microcatheterization studies in vivo have suggested that prostaglandins affect U Na excretion independently of hemodynamic changes. Subsequently, direct effects of PGE 2 on epithelial transport processes were demonstrated and found to vary considerably in different nephron segments. In the medullary thick ascending limb of Henle and collecting tubule, PGE 2 caused a decrease in the reabsorption of water, Na + , and Cl − that was correlated with reduced Na + -K + -ATPase activity. In contrast, in the distal convoluted tubule, PGE 2 caused increased Na + -K + -ATPase activity. The net effect of locally produced prostaglandins on tubular Na + handling is probably inhibitory because complete blockade of prostaglandin synthesis by indomethacin in rats receiving a normal or salt-loaded diet increased fractional Na + reabsorption and enhanced the activity of the renal medullary Na + -K + -ATPase. In addition, PGE 2 inhibited AVP-stimulated NaCl reabsorption in the medullary thick ascending limb of Henle and AVP-stimulated water reabsorption in the collecting duct. Both these effects tend to antagonize the overall hydroosmotic response to AVP. However, because no such effect is seen in the cortical thick ascending limb of Henle, which is capable of augmenting NaCl reabsorption in response to an increased delivered load, and because the effects of prostaglandins on solute transport in the collecting tubule remain unresolved, no conclusions can be reached from these studies about the contribution of direct epithelial effects of prostaglandins to overall Na + excretion.
In whole animal and clinical balance studies, researchers have examined the effects of prostaglandin infusion or prostaglandin synthesis inhibition on urinary Na + excretion, or have attempted to correlate changes in urinary prostaglandin excretion with changes in salt balance; these studies have also yielded conflicting and inconclusive results. Nevertheless, as elaborated earlier, prostaglandins have an important role in states of Na + imbalance (real or perceived Na + depletion) in which they are involved to preserve GFR by countervailing renal vasoconstrictive influences.
The influence of changes in Na + intake on renal COX-1 and COX-2 expression has been studied extensively. The expression of COX-2 in the macula densa and thick ascending limb of Henle is increased by a low-salt diet, inhibition of RAAS, and renal hypoperfusion. In contrast, a high-salt diet has been reported to decrease COX-2 expression in the renal cortex. None of these changes in Na + intake affect the expression of COX-1 in the cortex. In the medulla, whereas a low-salt diet downregulated both COX-1 and COX-2, a high-salt diet enhanced the expression of these cyclo-oxygenase isoforms. In vitro studies have shown that high osmolarity of the medium of cultured IMCD cells induces the expression of COX-2. Infusion of nimesulide (a selective COX-2 inhibitor) into anesthetized dogs on a normal Na + diet reduced U Na excretion and urine flow rate, despite the lack of effect on renal hemodynamics or systemic blood pressure.
Collectively, the differential regulation of COX-2 in the renal cortex and medulla can be integrated into a physiologically relevant model in which upregulation of COX-2 in the cortical thick ascending limb of Henle and macula densa is induced in a volume-contracted or vasoconstrictor state. In the cortical thick ascending limb of Henle, the effect is by direct inhibition of Na + excretion, whereas in the macula densa, COX-2 stimulates renin release, which leads to Ang II–mediated Na + retention. In contrast, medullary COX-2 is induced by a high-salt diet, which leads to net Na + excretion.
Finally, in addition to the hemodynamically mediated and potential direct epithelial effects of prostaglandins, these agents may mediate the physiologic responses to other hormonal agents. The intermediacy of prostaglandins in renin release responses has already been cited. As another example, some, but not all, of the known physiologic effects of bradykinin and other products of the kallikrein-kinin system are mediated through bradykinin-stimulated prostaglandin production (e.g., inhibition of AVP-stimulated osmotic water permeability in the cortical collecting tubule). In addition, the renal and systemic actions of Ang II appear to be differentially regulated by prostaglandin production that is catalyzed by COX-1 and COX-2. For example, COX-2 deficiency in mice, induced by COX-2 inhibitors or gene knockout, dramatically augmented the systemic pressor effect of Ang II, whereas COX-1 deficiency abolished this pressor effect. Similarly, Ang II infusion reduced medullary blood flow in COX-2–deficient animals, but not in COX-1–deficient animals, which suggests that COX-2–dependent vasodilators are synthesized in the renal medulla. Moreover, the diuretic and natriuretic effects of Ang II were absent in COX-2–deficient animals but remained in COX-1–deficient animals. Thus, COX-1 and COX-2 exert opposite effects on systemic blood pressure and renal function.
Natriuretic Peptides.
The physiologic and pathophysiologic roles of the NP family in the regulation of Na + and water balance have been extensively investigated since the discovery of ANP by de Bold and colleagues. ANP is an endogenous, 28–amino acid peptide secreted mainly by the right atrium. In addition to ANP, three other NPs have renal effects—BNP, CNP, and DNP. Although encoded by different genes, these peptides are highly similar in chemical structure, gene regulation, and degradation pathways, constituting a hormonal system that exerts various biologic actions on the renal, cardiac, and blood vessel tissues. ANP plays an important role in blood pressure and volume homeostasis through its ability to induce natriuretic/diuretic, and vasodilatory responses. BNP has an amino acid sequence similar to that of ANP, with an extended NH 2 -terminus. In humans, BNP is produced from pro–brain NP (proBNP), which contains 108 amino acids and, in accordance with a proteolytic process, releases a mature, 32–amino acid molecule and N-terminal fragment into the circulation. Although BNP was originally cloned from the brain, it is now considered a circulating hormone produced mainly in the cardiac ventricles. CNP, which is produced mostly by endothelial cells, shares the ring structure common to all NP members; however, it lacks the C-terminal tail. DNP is released by the kidney and is a more effective activator of renal functions than ANP.
The biologic effects of the natriuretic peptides (NPs) are mediated by binding the peptide to specific membrane receptors localized to numerous tissues, including the vasculature, renal arteries, glomerular mesangial and epithelial cells, collecting ducts, adrenal zona glomerulosa, and the CNS. At least three different subtypes of NP receptors have been identified—NP-A, NP-B, and NP-C. NP-A and NP-B, single-transmembrane proteins with molecular weights of approximately 120 to 140 kDa, mediate most of the biologic effects of NPs. Both are coupled to guanylate cyclase in their intracellular portions. After binding to their receptors, all three NP isoforms markedly increase cGMP in target tissues and in plasma. Therefore, analogues of cGMP or inhibitors of degradation of this second messenger mimic the vasorelaxant and renal effects of NPs. The third class of NP-binding receptors, NP-C (molecular weight, 60 to 70 kDa), is believed to serve as a clearance receptor because it is not coupled to any known second-messenger system. ANP-C is the most abundant type of NP receptor in many key target organs of NPs.
Two additional routes for the removal of NPs are worth noting. The first well-established pathway is the enzymatic degradation by neutral endopeptidase (NEP) 24.11, a metalloproteinase located mainly in the lungs and the kidneys. This pathway has undergone extensive research in attempts to find specific inhibitors that would lead to enhanced NP activity in situations of Na + retention (see section, “ Specific Treatments Based on the Pathophysiology of Congestive Heart Failure ”). The second route is entirely novel and involves the negative regulation of ANP by microRNA-425 (miRNA-425). In this context, carriers of the rs5068 minor G allele of the gene encoding ANP, NPPA, have a 15% lower risk of hypertension and ANP levels 50% higher than those with two copies of the major A allele. miRNA-425, expressed in human atria and ventricles, is predicted to bind the sequence spanning rs5068 in the 3′ untranslated region of the A, but not G, allele. Only the A allele was silenced by miRNA-425, whereas possession of the G allele conferred resistance to miR-425. The results raise the possibility that miR-425 antagonists could be used to treat disorders of salt overload, such as hypertension and heart failure.
Atrial Natriuretic Peptide.
Both in vivo and in vitro studies, in humans and experimental animals, have established the role of ANP in the regulation of ECFV and blood pressure by acting on all organs and tissues involved in the homeostasis of Na + and blood pressure ( Table 15.4 ). Therefore, it is not surprising that ANP and NH 2 -terminal ANP levels are increased in certain situations: (1) conditions associated with enhanced atrial pressure; (2) systolic or diastolic cardiac dysfunction; (3) cardiac hypertrophy/remodeling; and (4) severe myocardial infarction. In the kidneys, ANP exerts hemodynamic/glomerular effects that increase Na + and water delivery to the tubule in combination with inhibitory effects on tubular Na + and water reabsorption, which lead to remarkable diuresis and natriuresis.
Target Organ | Biologic Effects |
---|---|
Kidney |
|
| |
| |
Cardiac |
|
Hemodynamic |
|
Endocrine |
|
Mitogenesis |
|
In addition to its powerful diuretic and natriuretic activities, ANP also relaxes vascular smooth muscle and leads to vasodilation by antagonizing the concomitant vasoconstrictive influences of Ang II, endothelin, AVP, and α 1 -adrenergic input. This vasodilation reduces preload, which results in a fall in cardiac output. In addition, ANP reduces cardiac output by shifting fluid from the intravascular to extravascular compartment, an effect mediated by increased capillary hydraulic conductivity for water. Studies in endothelial-restricted, GC-A knockout mice have found that ANP, through GC-A, enhances albumin permeability in the microcirculation of the skin and skeletal muscle. This effect is mediated by caveolae and is critically involved in the endocrine hypovolemic and hypotensive actions of the cardiac hormone in vivo.
ANP has also been shown to exert antiproliferative, growth regulatory properties in cultured glomerular mesangial cells, vascular smooth muscle cells, and endothelial cells. Within the kidneys, ANP causes afferent vasodilation, efferent vasoconstriction, and mesangial relaxation, which lead to increases in glomerular capillary pressure, GFR, and filtration fraction. In combination with increased medullary blood flow, these hemodynamic effects enhance diuresis and natriuresis. However, the overall natriuretic effect of ANP infusion does not require these changes in glomerular function (except in response to larger doses of the peptide). At the tubular level, ANP inhibits the stimulatory effect of Ang II on the luminal Na + /H + exchanger of the proximal tubule. Similarly, ANP, acting through cGMP, inhibits the thiazide-sensitive NaCl cotransporter in the distal tubule and ENaC in the collecting duct, along with inhibition of AVP-induced AQP2 incorporation into the apical membrane of these segments of the nephron (see Table 15.4 ).
Brain Natriuretic Peptide.
BNP is produced by activated satellite cells in ischemic skeletal muscle or by cardiomyocytes in response to pressure load, thereby regulating the regeneration of neighboring endothelia through GC-A. BNP-mediated paracrine communication may be critically involved in coordinating muscle regeneration or hypertrophy and angiogenesis. However, the administration of BNP to human subjects induces natriuretic, endocrine, and hemodynamic responses similar to those induced by ANP.
BNP is produced and secreted mainly by the ventricles but also, in small amounts, by the atrium. Increased volume or pressure overload states such as HF and hypertension enhance the secretion of BNP from the ventricles. Despite the comparable elevation in plasma levels of ANP and BNP in patients with HF and other chronic volume-expanded conditions, acute intravenous saline loading or infusion of pressor doses of Ang II yields different patterns of ANP and BNP secretion. Whereas plasma levels of ANP increase rapidly, the changes in plasma BNP of atrial origin are negligible, as expected in view of the minimal atrial content of BNP, in contrast to the abundance of ANP. Moreover, plasma levels of BNP rise with age, from 26 ± 2 pg/mL in subjects aged 55 to 64 years to 31 ± 2 pg/mL in those aged 65 to 74 years and to 64 ± 6 pg/mL in those 75 years of age or older.
Studies in animals and humans have demonstrated the natriuretic effects of pharmacologic doses of BNP. When administered to normal volunteers and hypertensive subjects at low doses, BNP induces a significant increase in U Na excretion and, to a lesser extent, in urinary flow. Significant natriuresis and diuresis were observed after the infusion of ANP or BNP to normal subjects. The combination of ANP and BNP did not produce a synergistic renal effect, which suggests that these peptides share similar mechanisms of action. Moreover, like ANP, BNP exerts a hypotensive effect in animals and humans. For example, transgenic mice that overexpress the BNP gene exhibit significant and lifelong hypotension to the same extent as transgenic mice that overexpress the ANP gene. Therefore, it is clear that BNP induces its biologic actions through mechanisms similar to those of ANP.
This notion is supported by several findings: (1) both ANP and BNP act through the same receptors, and both induce similar renal, cardiovascular, and endocrine actions in association with an increase in cGMP production (see Table 15.4 ); and (2) BNP suppresses ACTH-induced aldosterone generation both in cell culture and when BNP is infused in vivo. The latter action may be attributed to BNP inhibition of renin secretion, at least in dogs, although apparently not in humans. Similar to the hemodynamic effects of ANP, those of BNP vary according to dose and species. When injected as a bolus at high doses, BNP caused a profound fall in systolic blood pressure in humans; however, when infused at low doses, this peptide fails to change blood pressure or heart rate. The effects of BNP have been used in the clinical setting in the diagnosis and treatment of the volume overload state of HF (see section, “ Specific Treatments Based on the Pathophysiology of Congestive Heart Failure ”).
C-Type Natriuretic Peptide.
Although CNP is considered a neurotransmitter in the CNS, considerable amounts of this NP are produced by endothelial cells, where it plays a role in the local regulation of vascular tone. Smaller amounts of CNP are produced in the kidneys, heart ventricles, and intestines. In addition, CNP, which could be of endothelial or cardiac origin, has been found in human plasma. The physiologic stimuli for CNP production have not been identified, although enhanced expression of CNP messenger RNA has been reported after volume overload. Intravenous infusion of CNP decreases blood pressure, cardiac output, urinary volume, and Na + excretion. Furthermore, the hypotensive effects of CNP are less pronounced compared to those of ANP and BNP, but CNP strongly stimulates cGMP production and inhibits vascular smooth muscle cell proliferation.
All three NPs inhibit the RAAS, although CNP does not induce significant changes in cardiac output, blood pressure, and plasma volume in sheep. This finding supports the widely accepted concept that ANP and BNP are the major circulating NPs, whereas CNP is a local regulator of vascular structure and tone.
D-Type Natriuretic Peptide.
DNP infused intravenously into rabbits increased urine volume and urinary excretion of electrolytes. These renal actions, induced by DNP, were more pronounced than those of ANP, possibly because of the degradation resistance of DNP against the endogenous peptidases in plasma or tissues. DNP, specifically via the NP-A receptor, induced the greatest cGMP production in glomeruli compared to other renal structures, including cortical tubules, outer medullary tubules, and inner medullary tubules. Thus, DNP appears to play a pivotal role, at least in sheep, as a renal regulating peptide via specific NP receptors with a guanylate cyclase domain.
Although all forms of NPs exist in the brain, the role and significance of their CNS expression in the regulation of salt and water balance are not understood. Together, the various biologic actions of NPs lead to reduction of EABV, an expected response to perceived overfilling of the central intrathoracic circulation. Furthermore, all NPs counteract the adverse effects of the RAAS, which suggests that the two systems are acting in opposite directions in the regulation of body fluid and cardiovascular homeostasis.
Endothelium-Derived Factors.
The endothelium is a major source of active substances that regulate vascular tone in healthy states and disease. The best known representatives are endothelin, nitric oxide, and PGI 2 . These vasoconstricting and vasodilating factors regulate the perfusion pressure of multiple organ systems that are strongly involved in water and Na + balance, such as the kidneys, heart, and vasculature. This section summarizes some concepts regarding actions of endothelin and nitric oxide that are relevant to volume homeostasis.
Endothelin.
The endothelin system consists of three vasoactive peptides—endothelin 1 (ET-1), endothelin 2 (ET-2), and endothelin 3 (ET-3). These peptides are synthesized and released mainly by endothelial cells and act in a paracrine and autocrine manner. ET-1, the major representative of the endothelin family, is still the most potent vasoconstrictor known (and see section, “ Urotensin ”). All endothelins are synthesized by proteolytic cleavage from specific prepro-endothelins that are further cleaved to form 37– to 39–amino acid precursors, called big endothelin. Big endothelin is then converted into the biologically active, 21 – amino acid peptide by a highly specific endothelin-converting enzyme (ECE), a phosphoramidon-sensitive, membrane-bound metalloprotease. To date, two isoforms of ECE have been identified, ECE-1 and ECE-2. ECE-1 exists as four different isoforms. ECE-2 is localized mainly to vascular smooth muscle cells and is probably an intracellular enzyme. In ECE-1 knockout mice, tissue levels of ET-1 are reduced by about one third, which suggests that ECE-independent pathways are involved in the synthesis of this peptide. In this regard, both chymase and carboxypeptidase A have been shown to be involved in mature endothelin production.
The endothelins bind to two distinct receptors, designated endothelin types A and B (ET-A and ET-B). The ET-A receptor shows a higher affinity for ET-1 than for ET-2 or ET-3. The ET-B receptor shows equal affinity for each of the three endothelins. ET-A receptors are found mainly on vascular smooth muscle cells, on which their activation leads to vasoconstriction through an increase in cytosolic Ca 2+ . ET-B receptors are also found on vascular smooth muscle cells, on which they can mediate vasoconstriction, but are found predominantly on vascular endothelium, in which their activation results in vasodilation through prostacyclin and nitric oxide. Endothelin is detectable in the plasma of human subjects and many experimental animals, and therefore may also act as a circulating vasoactive hormone.
Selective ET-A receptor antagonism is associated with vasodilation and a reduction in blood pressure, whereas selective ET-B antagonism is accompanied by vasoconstriction and a rise in blood pressure. These data suggest complementary roles for the endothelin receptor subtypes in the maintenance of vascular tone. In addition to its vasoconstrictive action, endothelin has a variety of effects on the kidneys. The kidney (mainly the inner medulla) is both a source and an important target organ of endothelin. ET-1 is synthesized by the endothelial cells of the renal vessels, whereas ET-1 and ET-3 are produced by various cell types of the nephron. ET-2 and ET-3 are produced at a rate one to two orders of magnitude lower than ET-1, which appears to be the principal subtype involved in renal functional regulation.
In relation to volume homeostasis, three major aspects of renal function are affected by ET-1 in a paracrine or autocrine manner: (1) renal and intrarenal blood flow; (2) glomerular hemodynamics; and (3) renal tubular transport of salt and water. Both ET-A and ET-B receptors are present in the glomerulus, renal vessels, and tubular epithelial cells, but most ET-B receptors are found in the medulla. The renal vasculature, in comparison with other vascular beds, appears to be most sensitive to the vasoconstrictor action of ET-1. Infusion of ET-1 into the renal artery of anesthetized rabbits was found to decrease RBF, GFR, natriuresis, and urine volume. Micropuncture studies have demonstrated that ET-1 increases afferent and efferent arteriolar resistance (afferent more than efferent), which results in a reduction in the glomerular plasma flow rate. In addition, Kf is reduced because of mesangial cell contraction, resulting in a diminished SNGFR.
The profound reduction of RBF and concomitant lesser reduction in GFR should result in a rise in filtration fraction, but the effect of ET-1 on the filtration fraction appears to be variable. Some groups, using low doses in a canine model, have reported an increase, and others have reported no significant effect. Infusion of ET-1 for 8 days into conscious dogs increased plasma levels of endothelin by twofold to threefold and resulted in increased renal vascular resistance and decreased GFR and RBF. Interestingly, the effect of endothelin on regional intrarenal blood flow is not homogeneous. Using laser Doppler flowmetry, administration of ET-1 in control rats produced a sustained cortical vasoconstriction and a transient medullary vasodilatory response. These results are in line with the medullary predominance of ET-B receptors and the high density of ET-A–binding sites in the cortex.
The effect of endothelin on Na + and water excretion varies and depends on the dose and source of endothelin. Systemic infusion of endothelin in high doses results in profound antinatriuresis and antidiuresis, apparently secondary to the decrease in GFR and RBF. However, in low doses, or when produced locally in tubular epithelial cells, endothelin decreases the reabsorption of salt and water, consistent with ET-1 target sites on renal tubules. Also, administration of the endothelin precursor, big endothelin, has been shown to cause natriuresis, which supports the notion of a direct inhibitory autocrine action of endothelin on tubular salt reabsorption.
The natriuretic and diuretic actions of big ET-1 can be significantly reduced by ET-B–specific blockade given acutely and chronically by osmotic minipump. Furthermore, ET-B knockout rats have salt-sensitive hypertension that is reversed by luminal ENaC blockade with amiloride, which suggests that ET-B in the collecting duct in vivo tonically inhibits ENaC activity, the final regulator of Na + balance. Similarly, mice with collecting duct–specific knockout of the ET-1 gene have impaired Na + excretion in response to Na + load and develop hypertension with a high salt intake. These mice also have heightened sensitivity to AVP and reduced ability to excrete an acute water load. These findings are in line with in vitro observations that ET-B mediates the inhibitory effects of ET-1 on Na + and water transport in the collecting duct and thick ascending limb of Henle. Thus, if vascular and mesangial endothelin exerts a greater physiologic effect than tubule-derived endothelin, then RBF is diminished and net fluid retention occurs, whereas if the tubule-derived endothelin effect predominates, salt and water excretion are increased.
The ability of ET-1 to inhibit AVP-stimulated water permeability reversibly was first shown in the isolated perfused IMCD. ET-1 also reduces AVP-stimulated cAMP accumulation and water permeability in the IMCD, and mitigates the hydroosmotic effect of AVP in the cortical and outer medullary collecting ducts. Furthermore, studies of the rabbit cortical collecting duct have demonstrated that ET-1 may inhibit the luminal amiloride-sensitive ENaC by a Ca 2+ -dependent effect. Moreover, collecting duct–specific ET-1 knockout mice were shown to have an impaired ability to excrete Na + and water loads in comparison with their wild-type counterparts. Taking into account the fact that the medulla contains ET-B receptors and the highest endothelin concentrations in the body, and that endothelins also inhibit Na + -K + -ATPase in IMCD, these effects may contribute to the diuretic and natriuretic actions of locally produced ET-1. This may also explain the natriuretic effect of ET-1 reported by some investigators, despite the reduction in RBF and GFR.
Endothelin production in the kidneys is regulated differently than in the vasculature. Whereas vascular (and mesangial) endothelin generation is controlled by thrombin, Ang II, and transforming growth factor–β, tubular endothelin production seems to depend on entirely different mechanisms, of which medullary tonicity may be particularly important. Volume expansion in humans increases urinary endothelin excretion, suggestive of an inhibitory action of renal endothelin on water reabsorption, particularly in the collecting duct. Also, a high-salt diet, by raising medullary tonicity, stimulates ET-1 release, which in turn leads to increased eNOS (NOS3) expression and natriuresis. (The NOS-dependent ET-1 effects are discussed further in the “ Nitric Oxide ” section, below). Therefore, salt and water balance appear to regulate renal endothelin production and collecting duct fluid reabsorption by altering medullary tonicity. The signaling mechanisms for these phenomena, as well other renal actions of ET-1, continue to be a subject of intensive research, and the interested reader is referred to a recent review that summarizes the current state of knowledge.
Nitric Oxide.
Nitric oxide (NO) is a diffusible gaseous molecule produced from its precursor l -arginine by the enzyme NOS, which exists in three distinct isoforms—nNOS (NOS1), inducible NOS (iNOS, or NOS2), and eNOS (NOS3). NOS is expressed in endothelial cells of the renal vasculature (mainly eNOS), tubular epithelial and mesangial cells, and macula densa (mainly nNOS). There is controversy regarding the renal expression of iNOS in normal kidneys, but upregulation of this isoform is clearly seen in pathologic conditions such as ischemia-reperfusion injury.
The availability of selective NOS inhibitors and NOS knockout mice has facilitated the investigation of the individual role of the NOS isoforms in the regulation of renal function. However, the role of specific nitric oxide isoforms in a given cell type is still a work in progress. Therefore, this discussion refers to the renal effects of nitric oxide regardless of its isoform, unless otherwise specified.
The action of nitric oxide is mediated by activation of a soluble guanylate cyclase (sGC), thereby increasing intracellular levels of its second messenger, cGMP. In the kidneys, the physiologic roles of nitric oxide include the regulation of glomerular hemodynamics, attenuation of TGF, mediation of pressure natriuresis, maintenance of medullary perfusion, inhibition of tubular Na + reabsorption, and modulation of RSNA. Renal NOS activity is regulated by several humoral factors, such as Ang II (see earlier section, “ Tubuloglomerular Feedback ”) and salt intake.
The role of nitric oxide in the regulation of renal hemodynamics and excretory function is best illustrated by the fact that inhibition of intrarenal nitric oxide production results in increased blood pressure and impaired renal function. Infusion of the NOS inhibitor, N g -monomethyl- l -arginine ( l -NMMA), into one kidney in anesthetized dogs resulted in a dose-dependent decrease in urinary cGMP levels, decreases in RBF and GFR, Na + and water retention, and a decline in fractional Na + excretion in the ipsilateral kidney in comparison with the contralateral kidney. In addition, acute nitric oxide blockade amplified the renal vasoconstrictive action of Ang II in isolated microperfused rabbit afferent arterioles and in conscious rats, which suggests that nitric oxide and Ang II interact in the control of renal vasculature. This concept is supported by the finding that l -NMMA–induced vasoconstriction leads to decreased RBF and Kf and is prevented by RAAS blockade; thus, some of the major effects of nitric oxide are to counterbalance the vasoconstrictive action of Ang II.
Nitric oxide has also been shown to exert a vasodilatory action on afferent arterioles and to mediate the renal vasorelaxant actions of acetylcholine, but not bradykinin. The counterbalancing effect of nitric oxide on Ang II–induced efferent arteriolar vasoconstriction and its role in regulating TGF and in modulating renin secretion by the juxtaglomerular apparatus have been discussed earlier (see section, “ Tubuloglomerular Feedback ”).
The involvement of nitric oxide in the regulation of Na + balance is well characterized. In conscious dogs on a normal Na + diet, nitric oxide inhibition induces a significant decrease in natriuresis and diuresis without a change in arterial pressure. In dogs receiving a high-Na + diet and treatment with the nitric oxide inhibitor, N G -nitro- l -arginine methyl ester ( l -NAME), both arterial pressure and cumulative Na + balance were higher than in dogs receiving a comparable diet but no treatment with nitric oxide inhibitors. Exposure of rats to a high salt intake (1% NaCl drinking water) for 2 weeks induced increased serum concentration and urinary excretion of the nitric oxide metabolites, NO 2 and NO 3 . Urinary NO 2 + NO 3 and Na + excretion were significantly correlated. The increase in urinary nitric oxide metabolites is attributed to the enhanced expression of all three NOS isoforms in the renal medulla by high salt intake. These findings suggest that nitric oxide has a role in promoting diuresis and natriuresis in normal and increased salt intake/volume-expanded states.
The action of l -NAME infused directly into the renal medullary interstitium of anesthetized rats to reduce papillary blood flow, in association with decreased Na + and water excretion, indicates that nitric oxide exerts a vasodilatory effect on the renal medullary circulation and promotes Na + excretion. Consistent with these data are the findings of high levels of eNOS in the renal medulla and the inhibitory effect of nitric oxide on Na + -K + -ATPase in the collecting duct. Additional evidence of the involvement of the nitric oxide system in Na + homeostasis has been derived from studies in which researchers examined the mechanism of salt-sensitive hypertension. According to these studies, activity of NOS, mainly nNOS, is significantly lower in salt-sensitive rats than in salt-resistant rats maintained on a high-salt diet. In another study, intravenous l -arginine increased nitric oxide production and prevented the development of salt-induced hypertension in Dahl salt-sensitive rats. These findings suggest that nNOS plays an important role in Na + handling and that decreases in nNOS activity may in part be involved in the mechanism of salt-sensitive hypertension.
The involvement of nitric oxide in the abnormal Na + handling in hypertension could result from an inadequate direct effect on tubular Na + reabsorption in proximal and distal segments. However, attenuated inhibitory actions of nitric oxide on renin secretion and TGF may also contribute to salt retention and subsequent hypertension. In this context, investigators concluded that nitric oxide originating from the macula densa blunted the TGF-mediated vasoconstriction during high salt intake in salt-resistant rats, whereas in salt-sensitive rats, this response was lost. As noted, there is strong evidence that the medullary and other effects of nitric oxide occur in response to local endothelin production. For example, the inhibition of NOS by l -NAME or the highly selective ET-B antagonist A-192621 abolished the diuretic and natriuretic effects of big ET-1 in the kidneys of anesthetized rats. In addition, ET-1 acutely activated eNOS in the isolated medullary thick ascending limb of Henle and nNOS in isolated IMCD cells, via ET-B activation. Studies in ET-B receptor–deficient rats have shown that this activation of nNOS and eNOS is accompanied by an increase in nNOS protein but no change in messenger RNA expression. These data suggest that nNOS and eNOS activation occur by posttranscriptional pathways. NO also reduces Cl − absorption in the cortical collecting duct (CCD) through a mechanism that is ENaC dependent.
Activation of eNOS in the IMCD, where the highest renal NOS activity is found, is also associated with inhibition of Na + reabsorption in the medullary thick ascending limb of Henle through phosphatidylinositol-3-kinase (PI3K)–stimulated Akt activity, leading to eNOS phosphorylation at Ser1177. Thus, ET-1 has a paracrine effect on eNOS expression in the IMCD. However, the functional corollary of nNOS activation in the IMCD remains to be determined. A further action of nitric oxide is the inhibition of AVP-enhanced Na + reabsorption and hydroosmotic water permeability of the cortical collecting duct. A new mouse model of specific collecting duct NOS1 gene deletion should be a valuable tool to study the signaling mechanisms involved in the nitric oxide effects on AVP-enhanced Na + reabsorption, as well as salt-dependent blood pressure mechanisms in general. The role of nitric oxide in pressure natriuresis and RSNA is discussed in the relevant sections.
Kinins.
The kallikrein-kinin system is a complex cascade responsible for the generation and release of vasoactive kinins. The active peptides bradykinin and kallidin are formed from precursors (kininogens) that are cleaved by tissue and circulatory kinin-forming enzymes. Kinins are produced by many cell types and can be detected in urine, saliva, sweat, interstitial fluid and, in rare cases, venous blood. The levels of bradykinin in the circulation are almost undetectable because of rapid metabolism by kininases, particularly kininase II/ACE1. The renal kallikrein-kinin system can produce local concentrations of bradykinin much higher than those present in blood. In the kidneys, bradykinin is metabolized by NEP.
Kinins play an important role in hemodynamic and excretory processes through their G protein–coupled receptors, BK-B 1 and BK-B 2 . The BK-B 2 receptors mediate most of the actions of kinins and are located mainly in the kidneys, although they are also detectable in the heart, lungs, brain, uterus, and testes. Activation of BK-B 2 receptors results in vasodilation, probably through a nitric oxide– or arachidonic acid metabolite–dependent mechanism. For example, bradykinin selectively increases perfusion of the medulla, especially of its inner layer, via activation of the NO system and of Ca 2+ -activated K + channels. Bradykinin is known for its multiple effects on the cardiovascular system, particularly vasodilation and plasma extravasation.
In addition to the vasculature, the kidney is an important target organ of kinins, in which they induce diuresis and natriuresis through activation of BK-B 2 receptors. These effects are attributed to an increase in RBF and to inhibition of Na + and water reabsorption via ENaC in the distal nephron. The latter effect is secondary to the observed action of kinins in reducing vascular resistance. Unlike many vasodilators, bradykinin increases RBF without significantly affecting GFR or Na + reabsorption at the proximal tubule level, but the accompanying marked decrease in water and salt reabsorption in the distal nephron contributes significantly to increased urine volume and Na + excretion.
Studies with transgenic animals have enriched the understanding of the physiologic role of the kinins and the interaction between the kallikrein-kinin system and the RAAS. For example, in the kidneys, Ang II acting through the AT 2 receptor stimulates a vasodilator cascade of bradykinin, nitric oxide, and cGMP during conditions of increased Ang II, such as Na + depletion. In the absence of the AT 2 receptor, pressor and antinatriuretic hypersensitivity to Ang II is associated with bradykinin and nitric oxide deficiency. Furthermore, involvement of the renal kinins in pressure natriuresis has been documented. Bradykinin also mediates the biologic actions of angiotensin-(1-7), as shown in rats transgenic for the kallikrein gene, which display significantly augmented angiotensin-(1-7)–mediated diuresis and natriuresis. Because ACE is involved in the degradation of kinins, ACE inhibitors not only attenuate the formation of Ang II but may also lead to the accumulation of kinins. The latter are believed to be responsible in part for the beneficial effects of ACE inhibitors in patients with HF, but also for their troublesome side effect of cough. On the basis of the results of these studies, as well as those in which BK-B 2 -specific antagonists were used, the kallikrein-kinin system is believed to play a pivotal role in the regulation of fluid and electrolyte balance, mainly by acting as a counterregulatory modulator of vasoconstrictor and Na + -retaining mechanisms.
Adrenomedullin.
Human adrenomedullin (AM) is a 52–amino acid peptide that was discovered in 1993 in extracts of human pheochromocytoma cells. Adrenomedullin is approximately 30% homologous in structure with calcitonin gene–related peptide and amylin. Adrenomedullin is produced from a 185–amino acid preprohormone that also contains a unique 20–amino acid sequence in the NH 2 -terminus, termed proadrenomedullin NH 2 -terminal 20 peptide. This sequence exists in vivo and has biologic activity similar to that of AM.
Adrenomedullin messenger RNA is expressed in several tissues, including those of the atria, ventricles, vascular tissue, lungs, kidneys, pancreas, smooth muscle cells, small intestine, and brain. The synthesis and secretion of AM are stimulated by chemical factors and physical stress. Among these stimulants are cytokines, corticosteroids, thyroid hormones, Ang II, norepinephrine, endothelin, bradykinin, and shear stress. AM immunoreactivity has been localized in high concentrations in pheochromocytoma cells, adrenal medulla, atria, pituitary gland and, at lower levels, in cardiac ventricles, vascular smooth muscle cells, endothelial cells, glomeruli, distal and medullary collecting tubules, and digestive, respiratory, reproductive, and endocrine systems.
Adrenomedullin acts through a 395–amino acid membrane receptor that structurally resembles a G protein–coupled receptor and contains seven transmembrane domains. AM receptors constitute the calcitonin receptor–like receptor and a family of receptor activity–modifying proteins. Activation of these receptors increases intracellular cAMP, which probably serves as a second messenger for the peptide. The most impressive biologic effect of AM is long-lasting and dose-dependent vasodilation of the vascular system, including the coronary arteries. Injection of AM into anesthetized rats, cats, or conscious sheep induces a potent and long-lasting hypotensive response associated with reduction in vascular resistance in the kidneys, brain, lungs, hind limbs, and mesentery. The hypotensive action of AM is accompanied by increases in heart rate and cardiac output caused by positive inotropic effects. The vasodilating effect of AM can be blocked by inhibiting NOS, which suggests that nitric oxide partly mediates the decrease in systemic vascular resistance.
In addition to its hypotensive action, AM increases RBF through preglomerular and postglomerular arteriolar vasodilation. The AM-induced hyperperfusion is associated with dose-dependent diuresis and natriuresis. These effects result from a decrease in tubular Na + reabsorption, despite the AM-induced hyperfiltration and may be mediated partially by locally released nitric oxide and prostaglandins. In addition, NEP inhibition potentiates exogenous AM-induced natriuresis without affecting GFR. Like NPs, AM suppresses aldosterone secretion in response to Ang II and high potassium levels. Furthermore, in cultured vascular smooth muscle cells, AM inhibits endothelin production induced by various stimuli. AM acts in the CNS to inhibit water and salt intake. In the hypothalamus, AM inhibits the secretion of AVP, an effect that may also contribute to its diuretic and natriuretic actions.
Together, these findings show that AM is a vasoactive peptide that may be involved in the physiologic control of renal, adrenal, vascular, and cardiac function. Furthermore, the existence of AM-like immunoreactivity in the glomerulus and collecting tubule, in association with detectable amounts of AM messenger RNA in the kidneys, suggests that AM plays a renal paracrine role.
Two other members of the AM family, AM-2 (intermedin) and AM-5, have been identified. AM-2 is about 30% homologous with AM and has renal and cardiovascular effects similar to those of AM-1. AM-5 has cardiovascular effects similar to those of AM-1, but no apparent renal effects, in normal animals.
Urotensin.
Urotensin II is a highly conserved peptide that binds to the human orphan G protein–coupled receptor GPR14, now termed the urotensin II receptor. The parent peptide, prepro–urotensin II, is widely expressed in human tissues, including those of the CNS and peripheral nervous system, GI tract, vascular system, and kidneys. In the kidneys, immunoreactive staining for urotensin II was detected in the epithelial cells of the tubules, mostly in the distal tubule, with moderate staining in endothelial cells of the renal capillaries. The C-terminus of the prohormone is cleaved to produce urotensin II, an 11–amino acid residue peptide. The human form of urotensin II includes a cyclic hexapeptide sequence that is fundamental for the action of this compound. The metabolic pathway leading to the production of urotensin II still remains incompletely characterized. Substantial urotensin II arteriovenous gradients (36% to 44%) have been demonstrated in the heart, liver, and kidneys, indicative of local urotensin II production.
In vivo in humans, systemic infusion of urotensin II range leads to local vasoconstriction in the forearm, no effect, or cutaneous vasodilation. These dissimilarities are probably attributable to many factors, including species variation, site and modality of injection, dose, vascular bed, and functional conditions of the experimental model. Because urotensin II has been described as the most potent vasoconstrictor (see earlier section, “ Endothelin ”), it is reasonable to postulate that the vasoconstrictive action is direct, whereas the vasodilatory response may be mediated by other factors, such as cyclo-oxygenase products and nitric oxide.
The involvement of the urotensin II system in the regulation of renal function in mammals is still unclear, and the data reported to date are as contradictory as those for vascular tone. In normal rats, intravenous boluses in the nanomolar range caused minor reductions in GFR and no effect on Na + excretion. However, in another study in which the same model was used, continuous infusion of urotensin II at doses in the picomolar range elicited clear increases in GFR and nitric oxide–dependent diuresis and natriuresis. In contrast, bolus injections in the picomolar range produced a dose-dependent decrease in GFR associated with reduced urine flow and Na + excretion. Studies in rats with an aortocaval fistula (a model of chronic volume overload) have shown that urotensin II boluses in the nanomolar range exert favorable, nitric oxide–dependent, renal hemodynamic effects. Thus, the effect of urotensin II on renal function seems dependent on the modality of administration (bolus vs. continuous infusion) and on the experimental condition being investigated (normal rats vs. those with heart failure).
The variability in renal and vascular responses to urotensin II administration may also depend on the fact that the action of this peptide is regulated at the receptor level. The binding density of urotensin II is correlated with vasoconstrictor response in rats, and small changes in receptor density may result in pathophysiologic effects. Under normal conditions, most urotensin II receptors are already occupied by urotensin II. Changes in unoccupied receptor reserve—perhaps in response to alterations in urotensin II levels generated in experimental models or observed in disease states—might explain, at least in part, the observed variability in studies of renal and vascular function.
Selective urotensin II receptor antagonists have been developed, the most potent of which currently is urantide. In normal rats, continuous administration of this compound increases GFR, as well as urine flow and Na + excretion. On the basis of experimental results indicating that urotensin II increases epithelial Na + transport in fish, it appears likely that urotensin II exerts a direct tubular effect, inasmuch as its receptor is expressed in the distal tubule. In light of the contradictory effects of urotensin II on renal function, the focus on potential clinical applications of urotensin 2 inhibition has moved away from the kidney to the treatment of chronic lung and cardiovascular diseases.
Digitalis-Like Factors.
In the early 1960s, Clarkson, de Wardener, and coworkers hypothesized the existence of endogenous digitalis-like factors, and an endogenous, ouabain-like compound in human and other mammalian plasma was initially reported in the late 1970s. Since 2000, interest in such factors—also known as endogenous cardiotonic steroids—has expanded considerably. In particular, two specific cardiotonic steroids in humans have been characterized extensively, endogenous cardenolide (or ouabain) and bufadienolide (marinobufagenin). An alternative mechanism whereby cardiotonic steroids can signal through the Na + -K + -ATPase has also been described. The main site of synthesis of these compounds is the adrenal cortex, and the main consequences of Na + pump inhibition are attenuation of renal Na + transport and increased cytosolic Ca 2+ in vascular smooth muscle cells, which lead to increased vascular resistance. The latter mechanism has been implicated in the pathogenesis of hypertension. These hormones also play a role in the regulation of cell growth, differentiation, apoptosis, and fibrosis, in the modulation of immunity and carbohydrate metabolism, and in the control of various central nervous functions, including behavior.
Neuropeptide Y.
Neuropeptide Y, a 36-residue peptide, is a sympathetic cotransmitter stored and released together with noradrenaline by adrenergic nerve terminals of the SNS. Structurally, neuropeptide Y is highly homologous to two other members of the pancreatic polypeptide family, peptide YY and pancreatic polypeptide. These two closely related peptides are produced and released by the intestinal endocrine and pancreatic islet cells, respectively, and act as hormones. Although neuropeptide Y was originally isolated from the brain and is highly expressed in the CNS, the peptide exhibits a wide spectrum of biologic activities in the cardiovascular system, GI tract, and kidneys through multiple G i/ o protein–coupled receptors—Y1, Y2, Y4, and Y5.
In numerous studies, in vivo and in vitro techniques have demonstrated the capacity of neuropeptide Y to reduce RBF and increase renal vascular resistance in various species, including humans. Despite the potent vasoconstrictor effect of the peptide on renal vasculature, this effect does not appear to be associated with any significant change in GFR. Similarly, neuropeptide Y may exert a natriuretic or antinatriuretic action, depending on the experimental conditions and the species studied. These data suggest that neuropeptide Y is unlikely to have a significant role in the physiologic regulation of renal hemodynamics and electrolyte excretion.
Apelin.
Apelin is the endogenous ligand of the angiotensin-like receptor 1, a G protein–coupled receptor found to be involved in various physiologic events, such as water homeostasis, regulation of cardiovascular tone, and cardiac contractility. Apelin and its receptor are widely expressed in the CNS and in peripheral tissues, especially in endothelial cells. Apelin is also expressed in endothelial and vascular smooth muscle cells of glomerular arterioles and, to a lesser extent, in other parts of the nephron.
Angiotensin-like receptor 1 activation leads to inhibition of cAMP production and activation of the Na + /H + exchanger type 1. Through the former pathway, apelin enhances vascular dilation after the induction of eNOS, whereas the burst of Na + /H + exchanger type 1 activity in cardiomyocytes leads to a dose-dependent increase in myocardial contractility. With regard to the renal effects of apelin, direct injection into the hypothalamus of lactating rats inhibited AVP release and reduced circulating AVP. Conversely, water deprivation led to increased systemic AVP and decreased apelin levels. These findings suggest that AVP and apelin have a reciprocal relationship in controlling water diuresis.
Apelin appears to also counterregulate several effects of Ang II. For example, intravenous injection of apelin caused a nitric oxide–dependent fall in arterial pressure. Moreover, apelin receptor knockout mice displayed an enhanced vasopressor response to systemic Ang II. In addition, apelin modulated the abnormal aortic vascular tone in response to Ang II through eNOS phosphorylation in diabetic mice; this finding provided further evidence of a role for apelin in vascular function. Intravenous injection of apelin also induced a significant diuresis and caused vasorelaxation of Ang II–preconstricted efferent and afferent arterioles. Activation of endothelial apelin receptors caused release of nitric oxide, which inhibited the Ang II–induced rise in intracellular Ca 2+ levels. Furthermore, apelin had a direct receptor-mediated vasoconstrictive effect on vascular smooth muscle. These results indicate that apelin has complex effects on the preglomerular and postglomerular microvasculature regulating renal hemodynamics. A direct role in tubular function remains to be determined but is suggested by collecting duct expression in close proximity to the vasopressin V 2 receptor.
Glucagon-Like Peptide-1
The incretin hormone glucagon-like peptide-1 (GLP-1) is released from the gut in response to fat or carbohydrate and contributes to negative feedback control of blood glucose by stimulating insulin secretion, inhibiting glucagon, and slowing gastric emptying. GLP-1 receptors (GLP-1Rs) are also expressed in the proximal tubule and possibly elsewhere in the kidney. The GLP-1 agonist, exenatide, has natriuretic effects by reducing proximal tubular reabsorption of sodium, an effect that may be mediated by Ang II inhibition. Exenatide also increased SNGFR by 33% to 50%, doubled early distal flow rate, and increased urine flow rate sixfold without altering the efficiency of glomerulotubular balance, TGF responsiveness, or the tonic influence of TGF. This implies that exenatide is a proximal diuretic and a renal vasodilator. Because the natural agonist for the GLP-1 receptor is regulated by intake of fat and carbohydrate, but not by salt or fluid, the control of salt excretion by the GLP-1R system departs from the usual negative-feedback paradigm for regulating salt balance.
Novel Factors
A novel intrarenal paracrine mechanism for sodium regulation in mice has been described. Earlier studies showed that changes in dietary acid-base load can reverse the direction of apical transport of the tricarboxylic acid intermediate, α-ketoglutarate (α-KG), in the proximal tubule and loop of Henle from reabsorption following an acid load to secretion following a base load. Recent work on isolated microperfused cortical collecting ducts from Oxgr1 −/ − mice has indicated that the concentration of α-KG is sensed by the α-KG receptor, OXGR1, expressed in the type B and non-A, non-B intercalated cells of the connecting tubule and cortical collecting duct. Addition of 1 mM α-KG to the tubular lumen strongly stimulated Cl-dependent HCO 3 − secretion and electroneutral NaCl reabsorption in tubules of wild-type but not Oxgr1 −/ − mice. Oxgr1 −/ − mice also displayed significantly increased functional activity of ENaC, without changes in plasma aldosterone. In contrast, α-KG has been shown to inhibit amiloride-sensitive sodium reabsorption in principal cells independently of OXGR1 activation. This effect is possibly related to increased ATP production inducing autocrine activation of P2Y2 receptors and, thereby, inhibition of ENaC. It appears that receptor-dependent and receptor-independent effects of α-KG converge to compensate for an alkalosis-induced decrease in proximal tubular reabsorption of NaCl by favoring NaCl reabsorption over Na + /K + exchange along the connecting tubule and cortical collecting duct. Taken together, the data indicate that α-KG acts as a paracrine mediator involved in the functional coordination of proximal and distal parts of the tubule in the adaptive regulation of HCO 3 − secretion and NaCl reabsorption in the presence of acid-base disturbances.
Members of the epidermal growth factor (EGF) family have been shown to be important for maintaining transepithelial Na + transport. For example, a high salt diet was shown to decrease cortical EGF levels promoting ENaC-mediated Na + reabsorption in the collecting duct and the development of hypertension. Conversely, EGF infused intravenously decreased ENaC activity, prevented the development of hypertension, and attenuated glomerular and renal tubular damage in the Dahl salt-sensitive rat. The implications of these observations are eagerly awaited.
The role of obesity in the pathogenesis of hypertension and renal dysfunction has led to the exploration of appetite-related hormones in salt and water retention. In this context, the orexigenic hormone, ghrelin, secreted by the stomach, has recently been shown to stimulate Na + absorption through cAMP-dependent trafficking of ENaC in the cortical collecting duct. The effect appears to be mediated via ghrelin receptors in this nephron segment. Although ghrelin seems to be a physiologic regulator of ENaC, future studies will be needed to clarify its physiologic and pathologic roles in sodium homeostasis.
Another novel development is the growing interest in the circadian rhythmicity of many basic physiologic functions. These functional rhythms are driven, in part, by the circadian clock, a ubiquitous molecular mechanism allowing cells and tissues to anticipate regular environmental events and to prepare for them. This mechanism has been shown to play a role in the regulation and maintenance of RPF, GFR, tubular reabsorption, and secretion of Na + , Cl − and K + . Studies in clock-deficient mice have identified the 20-HETE synthesis pathway as one of the clock’s principal renal targets to mediate Na + excretion. The researchers suggested that the circadian clock affects blood pressure, at least in part, by exerting dynamic control over renal sodium handling.
Rhythmicity of salt regulation seems to occur not only at the circadian level but also on a longer periodic basis (so-called infradian rhythms). In a fascinating study on men involved in space flight simulations, Rakova and colleagues have shown that even on fixed salt diets (6, 9, or 12 g/day), daily Na + excretion exhibited aldosterone-dependent, weekly (circaseptan) rhythms, resulting in periodic Na + storage. Changes in total-body Na + (±200 to 400 mmol) exhibited monthly or longer period lengths, without parallel changes in body weight and extracellular water. These changes were directly related to urinary aldosterone excretion and inversely to urinary cortisol, suggesting rhythmic hormonal control. These findings suggest the existence of rhythmic Na + excretory and retention patterns independent of blood pressure or body water and irrespective of salt intake.
Sodium Balance Disorders
Hypovolemia
Definition
Hypovolemia is the condition in which the volume of the ECF compartment is reduced in relation to its capacitance. As noted, the reduction may be absolute or relative. In states of absolute hypovolemia, the Na + balance is truly negative, reflecting past or ongoing losses. Hypovolemia is described as relative when there is no Na + deficit but the capacitance of the ECF compartment is increased. In this situation, of reduced EABV, the ECF intravascular and extravascular (interstitial) compartments may vary in the same or opposite directions. ICF volume, reflected by measurements of plasma Na + or osmolality, may or may not be concomitantly disturbed; thus, hypovolemia may be classified as normonatremic, hyponatremic, or hypernatremic (see Chapter 16 ).
Etiology
The causes of hypovolemia are summarized in Table 15.5 . Absolute and relative hypovolemia, in turn, can have extrarenal or renal causes. Absolute hypovolemia results from massive blood loss or from fluid loss from the skin, GI or respiratory system, or kidneys. Relative hypovolemia results from states of vasodilation, generalized edema, or third-space loss. In absolute and relative hypovolemia, the perceived reduction in intravascular volume prompts the compensatory hemodynamic changes and renal responses described earlier (see section, “ Physiology ”).
Absolute |
Extrarenal |
|
Renal |
|
Relative |
Extrarenal |
|
Renal |
Severe nephrotic syndrome |
Pathophysiology
Absolute Hypovolemia
Extrarenal.
The most common causes of absolute hypovolemia include persistent diarrhea, vomiting, and massive bleeding, either gastrointestinal or as a result of trauma. The reduction in ECF volume is isotonic inasmuch as there is a proportionate loss of water and plasma. The consequent fall in systemic blood pressure leads to compensatory tachycardia and vasoconstriction, and the ensuing altered transcapillary Starling hydraulic forces enable a shift of fluid from the interstitial to intravascular compartment. In addition, the neural and hormonal responses to hypovolemia (see section, “ Physiology ”) result in renal Na + and water retention, with the aim of restoring intravascular volume and hemodynamic stability.
Similar compensatory mechanisms become activated after fluid losses from the skin, GI system, and respiratory system. Because of the large surface area of the skin, large amounts of fluid can be lost from this tissue, which can be caused by burns or excessive perspiration. Severe burns allow the loss of large volumes of plasma and interstitial fluid and can lead rapidly to profound hypovolemia. Without medical intervention, hemoconcentration and hypoalbuminemia supervene. As occurs after massive bleeding, the fluid loss is isotonic, so plasma Na + concentration and osmolality remain normal. In contrast, excessive sweating, induced by exertion in a hot environment, leads to hypotonic fluid loss as a result of the relatively low Na + concentration in this fluid (20 to 50 mmol/L). The resulting hypovolemia may therefore be accompanied by hypernatremia and hyperosmolality, and the type of fluid replacement must be tailored accordingly (see Chapter 16 ).
In addition to oral intake, the GI tract is characterized by the entry of approximately 7 L of isotonic fluid, the overwhelming majority of which is reabsorbed in the large intestine. Hence, in normal conditions, fecal fluid loss is minimal. However, in the presence of pathologic conditions, such as vomiting, diarrhea, colostomy, and ileostomy secretions, especially those caused by infection, considerable or even massive fluid loss may occur. The ionic composition, osmolality, and pH of secretions vary according to the part of the GI tract involved; therefore, the resulting hypovolemia is associated with a large spectrum of electrolyte and acid-base abnormalities (see Chapters 17 and 18 for further discussion).
In contrast to the massive losses that can occur from the skin and GI system, fluid loss from the respiratory tract—as occurs in febrile states and in patients who receive mechanical ventilation with inadequate humidification—is usually modest, and hypovolemia ensues only in the presence of accompanying causes. Finally, a special situation in which hypovolemia can occur is after excessive ultrafiltration in dialysis patients (see Chapter 65 ).
Renal.
As described earlier, when the GFR and plasma Na + concentration are normal, approximately 24,000 mmol of Na + is filtered/day. Even when GFR is markedly impaired, the amount of filtered Na + far exceeds the dietary intake. To maintain Na + balance, all but 1% of the filtered load is reabsorbed. However, if the integrity of one or more of the tubular reabsorptive mechanisms is impaired, serious Na + deficit and absolute volume depletion can occur. The causes of absolute renal Na + losses include pharmacologic agents and renal structural, endocrine, and systemic disorders (see Table 15.5 ). All the diuretics widely used to treat hypervolemic states may induce hypovolemia if administered in excess or inappropriately. In particular, the powerful loop diuretics furosemide, bumetanide, torsemide, and ethacrynic acid are often given in combination with diuretics acting on other tubular segments (e.g., thiazides, aldosterone antagonists, distal ENaC blockers, and carbonic anhydrase inhibitors). Patients receiving these combinations need to be carefully monitored and fluid balance scrupulously adjusted to prevent hypovolemia. Patients commonly at risk are those with HF or underlying hypertension who develop intercurrent infections.
In patients with hypertension, the frequent use of diuretics for treatment appreciably increases the risk of volume depletion. Osmotic diuretics, endogenous or exogenous, may also reduce tubular Na + reabsorption. Endogenous agents include urea, the principle molecule involved in the polyuric recovery phase of acute kidney injury and postobstructive diuresis, and glucose in hyperglycemia. In patients with increased intracranial pressure, exogenous agents, such as mannitol or glycerol, may be used to induce translocation of fluid from the ICF to the ECF compartment and decrease brain swelling. The resulting polyuria may be associated with electrolyte and acid-base disturbances, the nature of which depends on the complex interplay between fluid intake and intercompartmental fluid shifts.
Na + reabsorption may also be disrupted in inherited and acquired tubular disorders. Inherited disorders of the proximal tubules (e.g., Fanconi syndrome) and distal tubules (e.g., Bartter and Gitelman syndromes) may lead to salt-wasting states in association with other electrolyte or acid-base disturbances. Acquired disorders of Na + reabsorption may be acute, as in nonoliguric acute kidney injury, the period immediately after renal transplantation, the polyuric recovery phase of acute kidney injury, and postobstructive diuresis (see relevant chapters for further details), or they may be chronic as a result of tubulointerstitial diseases with a propensity for salt wasting. Chronic kidney disease of any cause is associated with heightened vulnerability to Na + losses because the ability to match tubular reabsorption with the sum of filtered load minus dietary intake is impaired.
In addition to intrinsic tubular disorders, endocrine and other systemic disturbances may lead to impaired Na + reabsorption. The principal endocrine causes are mineralocorticoid deficiency and resistance states. A controversial cause is the systemic disturbance known as cerebral salt wasting. In this condition, salt wasting is thought to occur in response to an as yet unidentified factor released in the setting of acute head injury or intracranial hemorrhage. The condition is usually diagnosed because of concomitant hyponatremia, and some experts doubt its independent existence, regarding cerebral salt wasting as essentially indistinguishable from the syndrome of inappropriate antidiuretic hormone.
An underappreciated, but not uncommon, clinical setting for renal Na + loss is after the administration of large volumes of intravenous saline to patients over several days after surgery or after trauma. In this situation, tubular reabsorption of Na + is downregulated. If intravenous fluids are stopped before full reabsorptive capacity is restored, volume depletion may ensue. The phenomenon can be minimized by graded reduction in the infusion rate, which allows Na + reabsorptive pathways to be restored gradually.
In the context of volume depletion, diabetes insipidus should be mentioned. However, because this results from a deficiency of or tubular resistance to AVP, water loss is the main consequence, and the impact on ECF volume is only minor. AVP-related disorders are considered in Chapter 10 , Chapter 11 , Chapter 16 .
Relative Hypovolemia
Extrarenal.
As outlined previously, the principal causes of relative hypovolemia are edematous states, vasodilation, and third-space loss (see Table 15.5 ). Vasodilation may be physiologic, as in normal pregnancy, or induced by drugs (hypotensive agents, such as hydralazine or minoxidil, that cause arteriolar vasodilation), or it may occur in sepsis during the phase of peripheral vasodilation and consequent low systemic vascular resistance.
Edematous states in which the EABV and, hence, tissue perfusion are reduced include HF, decompensated cirrhosis with ascites, and nephrotic syndrome. In severe HF, low cardiac output and resulting low systemic blood pressure lead to a fall in RPP. As in absolute hypovolemia, the kidneys respond by retaining Na + . Because the increased venous return cannot raise the cardiac output, a vicious cycle is created in which edema is further exacerbated and the persistently reduced cardiac output leads to further Na + retention. In decompensated cirrhosis, splanchnic venous pooling leads to decreased venous return, a consequent fall in cardiac output, and compensatory renal Na + retention. The pathophysiologic features of edematous states are discussed later in more detail (see section, “ Hypervolemia ”). Third-space loss occurs when fluid is sequestered into compartments not normally perfused with fluids, as in states of GI obstruction, after trauma, with burns, or in pancreatitis, peritonitis, or malignant ascites. The end result is that even though total body Na + is markedly increased, the EABV is severely reduced.
Renal.
Approximately 10% of patients with the nephrotic syndrome—especially children with minimal change disease, but also any patient with a serum albumin level lower than 2 g/dL—manifest the clinical signs of hypovolemia. The low plasma oncotic pressure is conducive to movement of fluid from the ECF compartment to the interstitial space, thereby leading to reduced EABV.
Clinical Manifestations
The clinical manifestations of hypovolemia depend on the magnitude and rate of volume loss, solute composition of the net fluid loss (i.e., the difference between input and output), and vascular and renal responses. The clinical features can be considered as being related to the underlying pathophysiologic process, hemodynamic consequences, and electrolyte and acid-base disturbances that accompany the renal response to hypovolemia. A detailed history often reveals the cause of volume depletion (bleeding, vomiting, diarrhea, polyuria, diaphoresis, medications).
The symptoms and physical signs of hypovolemia appear only when intravascular volume is decreased by 5% to 15% and are often related to tissue hypoperfusion. Symptoms include generalized weakness, muscle cramps, and postural lightheadedness. Thirst is prominent if concomitant hypertonicity is present (hypertonic hypovolemia). Physical signs are related to the hemodynamic consequences of hypovolemia and include tachycardia, hypotension, which may be postural, absolute, or relative to the usual blood pressure, and low central or jugular venous pressure. Elevation of jugular venous pressure, however, does not rule out hypovolemia, because of the possible confounding effects of underlying heart failure or lung disease. When volume depletion exceeds 10% to 20%, circulatory collapse is liable to occur, with severe supine hypotension, peripheral cyanosis, cold extremities, and impaired consciousness, extending even to coma. This is especially possible if fluid loss is rapid or occurs against a background of comorbid conditions. When the source of volume loss is extrarenal, oliguria also occurs. The traditional signs—reduced skin turgor, sunken eyes, and dry mucous membranes—are inconstant findings, and their absence is not considered useful for ruling out hypovolemia.
Diagnosis
The diagnosis of hypovolemia is based essentially on the clinical findings. Nevertheless, when the clinical findings are equivocal, various laboratory parameters may be helpful for confirming the diagnosis or for elucidating other changes that may be associated with volume depletion.
Laboratory Findings
Hemoglobin.
This may decrease if significant bleeding has occurred or is ongoing, but this change, which is caused by hemodilution that results from translocation of fluid from the interstitial to intravascular compartment, may take up to 24 hours. Therefore, stable hemoglobin does not rule out significant bleeding. Moreover, the adaptive response of hemodilution may moderate the severity of hemodynamic compromise and resulting physical signs. In hypovolemic situations that do not arise from bleeding, hemoconcentration is often seen, although this too is not universal, inasmuch as underlying chronic diseases that cause anemia may mask the differential loss of plasma.
Hemoconcentration may also be manifested as a rise in plasma albumin concentration if albumin-free fluid is lost from the skin, GI tract, or kidneys. On the other hand, when albumin is lost, either in parallel with other extracellular fluids (as in proteinuria, hepatic disease, protein-losing enteropathy, or catabolic states) or in protein-rich fluid (third-space sequestration, burns), significant hypoalbuminemia is observed.
Plasma Na + Concentration.
This may be low, normal, or high, depending on the solute composition of the fluid lost and the replacement solution administered by the patient or the treating physician. For example, the hypovolemic stimulus for AVP release may lead to preferential water retention and hyponatremia, especially if hypotonic replacement fluid is used. In contrast, the fluid content of diarrhea may be hypotonic or hypertonic, resulting in hypernatremia or hyponatremia, respectively. The plasma Na + concentration reflects the tonicity of plasma and provides no direct information about volume status, which is a clinical diagnosis.
Plasma K + and Acid-Base Parameters.
These can also change in hypovolemic conditions. After vomiting, and after some forms of diarrhea, loss of K + and Cl − may lead to alkalosis. More often, the principal anion lost in diarrhea is bicarbonate, which leads to hyperchloremic (non–anion gap) acidosis. When diuretics or Bartter and Gitelman syndromes (the inherited tubulopathies; see Chapter 45 ) are the cause of hypovolemia, hypokalemic alkalosis is again typically seen. On the other hand, U Na loss that occurs in adrenal insufficiency or is caused by aldosterone hyporesponsiveness is accompanied by a tendency for hyperkalemia and metabolic acidosis. Finally, when hypovolemia is sufficiently severe to impair tissue perfusion, high anion gap acidosis caused by lactic acid accumulation may be observed.
Blood Urea and Creatinine Levels.
These frequently rise in hypovolemic states, and this elevation reflects impaired renal perfusion. If tubular integrity is preserved, then the rise in urea level is typically disproportionate to that of creatinine (see Chapter 31 ). This results mainly because AVP enhances reabsorption of urea in the medullary collecting duct and as an effect of an increased filtration fraction on proximal tubule handling of urea. In the presence of severe hypovolemia, acute kidney injury may ensue, leading to loss of the differential rise in urea level. Proportional rises in urea and creatinine are also observed when hypovolemia occurs against a background of underlying renal functional impairment, as in chronic kidney disease, stages 3 to 5 (see Chapter 52 ).
Urine Biochemical Parameters.
These can be extremely useful in establishing the diagnosis of hypovolemia caused by extrarenal fluid losses if there is no intrinsic renal injury and the patient is oliguric. The expected renal response of Na + and water conservation, by enhanced renal tubular reabsorption, results in oliguria, urine specific gravity exceeding 1.020, Na + concentration less than 10 mmol/L, and osmolality higher than 400 mOsm/kg. When Na + concentration is 20 to 40 mmol/L, the finding of a fractional excretion of Na + ofless than 1% in the presence of a reduced GFR may be helpful. In a patient who previously had undergone diuretic therapy, especially with loop diuretics, these indices may merely reflect the U Na losses. In that case, fractional excretion of urea from less than 30% to 35% may help in the diagnosis of hypovolemia, although the specificity of this test is rather low.
When hypovolemia occurs in the presence of arterial vasodilation, as observed in sepsis, some, but not all, of the clinical manifestations of hypovolemia are observed. Thus, tachycardia and hypotension are usually present, but the extremities are warm, which suggests that perfusion is maintained. This finding is misleading because vital organs, particularly the brain and kidneys, are underperfused as a result of the hypotension. The presence of lactic acidosis helps establish the correct diagnosis. Reduction in the EABV, as manifested by relative hypotension, may be observed in generalized edematous states, even though there is an overall excess of Na + and water; however, this excess is maldistributed between the extracellular and interstitial spaces.
Treatment
Absolute Hypovolemia
General Principles.
The goals of treatment of hypovolemia are to restore normal hemodynamic status and tissue perfusion. These goals are achieved by reversal of the clinical symptoms and signs, described previously. Treatment can be divided into three stages: (1) initial replacement of the immediate fluid deficit; (2) maintenance of the restored ECF volume in the presence of ongoing losses; and (3) treatment of the underlying cause, whenever possible. The main strategies to be addressed by the clinician are the route, volume, rate of administration, and composition of the replacement and maintenance fluids. These are liable to change according to the patient’s response.
In general, when hypovolemia is associated with a significant hemodynamic disturbance, intravenous rehydration is required. (The use of oral electrolyte solutions in the management of infants and children is discussed in Chapter 75 .) The volume of fluid and rate of administration should be determined on the basis of the urgency of the threat to circulatory integrity, adequacy of the clinical response, and underlying cardiac function. Older patients are especially vulnerable to aggressive fluid challenge, and careful monitoring is required, particularly to prevent acute left ventricular failure and pulmonary edema that result from overzealous correction.
Sometimes the clinical signs do not point unequivocally to the diagnosis of hypovolemia, even though the history is strongly suggestive. Because invasive monitoring of central venous and pulmonary venous pressures has not been shown to improve outcomes in this situation, a so-called diagnostic fluid challenge can be performed. If the patient improves clinically, blood pressure and urine output increase, and no overt signs of heart failure appear over the succeeding 6 to 12 hours, then the diagnosis is substantiated and fluid therapy can be cautiously continued. Conversely, if overt signs of fluid overload appear, the fluid challenge can be stopped and diuretic therapy reinstituted.
The initial calculations for replacing the fluid deficit are based on hemodynamic status. It is notoriously difficult to calculate volume deficits; therefore, good clinical judgment is necessary for successful management. Patients with life-threatening circulatory collapse and hypovolemic shock require rapid intravenous replacement through the cannula with the widest bore possible. This replacement should continue until blood pressure is corrected and tissue perfusion is restored. In the second stage of fluid replacement, the rate of administration should be reduced to maintain blood pressure and tissue perfusion. In older patients and those with underlying cardiac dysfunction, the risk of over rapid correction and precipitating pulmonary edema is heightened; therefore, slower treatment is preferable, to allow gradual filling of the ECF volume rather than causing pulmonary edema and the threat of mechanical ventilation associated with adverse outcomes.
Composition of Replacement Fluids.
The composition of replacement fluid had been thought to be less critical than the rate of infusion. However, recent work has cast serious doubt on this and is discussed here. The two main categories of replacement solution are crystalloid and colloid solutions. Crystalloid solutions are based largely on NaCl of varying tonicity or dextrose. Isotonic (0.9%) saline, containing 154 mmol of Na + /L, is the mainstay of volume replacement therapy inasmuch as it is confined to the ECF compartment in the absence of deviations in Na + concentration. One L of isotonic saline increases plasma volume by approximately 300 mL; the rest is distributed to the interstitial compartment. In contrast, 1 L of 5% dextrose in water (D 5 W), which is also isosmotic (277 mOsm/L), is eventually distributed throughout all the body fluid compartments so that only 10% to 15% (100 to 150 mL) remains in the ECF. Therefore, D 5 W should not be used for volume replacement.
Administration of 1 L of 0.45% saline (77 mmol of Na + /L) in D 5 W is equivalent to giving 500 mL of isotonic saline and the same volume of solute-free water. The distribution of the solute-free compartment throughout all the fluid compartments would result in plasma dilution and reduction in the plasma Na + . Therefore, this solution should be reserved for the management of hypernatremic hypovolemia. Even in that situation, it must be remembered that volume replacement is less efficient than with isotonic saline and, early on during the treatment course, may cause plasma tonicity to fall too rapidly.
When hypovolemia is accompanied by severe metabolic acidosis (pH < 7.10; plasma HCO 3 − < 10 mmol/L), bicarbonate supplementation may be indicated. (For a discussion of bicarbonate balance, see Chapter 27 .) Because this anion is manufactured as 8.4% sodium bicarbonate (1000 mmol/L) for use in cardiac resuscitation, appropriate dilution is required for the treatment of acidosis associated with hypovolemia. Nephrologists are frequently called on for consultation in these situations, and they should be ready to provide detailed protocols for the preparation of isotonic NaHCO 3 . Two convenient methods are suggested. Either 75 mL (75 mmol) of 8.4% NaHCO 3 can be added to 1 L of 0.45% saline, or 150 mL of concentrated bicarbonate can be added to 1 L of D 5 W. Although the latter is hypertonic in the short term, it is unlikely to be harmful.
In the presence of accompanying hypokalemia, especially if metabolic alkalosis is also present, volume replacement solutions must be supplemented with K + . Commercially available 1-L solutions of isotonic saline supplemented with 10 or 20 mmol of KCl make this option safe and convenient. (For details, see Chapters 18 and 75 .) On the other hand, other commercially available crystalloid solutions containing lactate (converted by the liver to bicarbonate) and low concentrations of KCl were traditionally regarded as offering no advantage and less flexibility than isotonic saline. This “dogma” was recently challenged by the findings of a large prospective observational study performed in the intensive care unit setting. In this study, two periods were compared; in the control period, all patients received isotonic saline as fluid replacement, whereas during the intervention period, Hartmann solution (lactate-containing), Plasma-Lyte 148 (a balanced salt solution), or chloride-poor 20% albumin solution was administered. The chloride-poor solutions were associated with a significantly lower risk of all stages of subsequent acute kidney injury, as defined by the RIFLE ( R isk, I njury, F ailure, L oss, E nd-stage renal disease) criteria, even after adjustment for covariates. Clearly, these provocative results indicate the need for randomized control trials comparing chloride-rich with more balanced salt solutions for fluid resuscitation.
Colloid solutions include plasma itself, albumin, and high-molecular-weight carbohydrate molecules, such as hydroxyethyl starch and dextrans, at concentrations that exert colloid osmotic pressures equal to or greater than that of plasma. Because the transcapillary barrier is impermeable to these large molecules, in theory they expand the intravascular compartment more rapidly and efficiently than crystalloid solutions. Colloid solutions may be useful in the management of burns and severe trauma when plasma protein losses are substantial, and rapid plasma expansion with relatively small volumes is efficacious. However, when capillary permeability is increased, as in states of multiorgan failure or the systemic inflammatory response syndrome, colloid administration is ineffective. Moreover, randomized controlled studies in which crystalloid solutions were compared with colloid solutions have shown no survival benefit and even harm with some colloid solutions, particularly hydroxyethyl starch. Therefore, the much cheaper and more readily available crystalloid solutions should remain the mainstay of therapy pending further studies.
Relative Hypovolemia
Treatment of relative hypovolemia is more difficult than that of absolute hypovolemia because there is no real fluid deficit. If the relative hypovolemia is caused by peripheral vasodilation, as in a septic patient, it may be necessary to administer a crystalloid solution cautiously, such as isotonic saline, to maintain ECF volume until the systemic vascular resistance and venous capacitance return to normal. The excess volume administered can then be excreted by the kidneys. When vasodilation is more severe, vasoconstrictor agents may be needed to maintain systemic blood pressure. In the edematous states of severe HF, advanced cirrhosis with portal hypertension, and severe nephrotic syndrome, when EABV is low but there is an overall excess of Na + and water, treatment may be extremely problematic. Administration of crystalloid solution will, in all likelihood, lead to worsening interstitial edema without significantly affecting the EABV. In these situations, prognosis is determined by whether the underlying condition can be reversed.
Hypervolemia
Definition
Hypervolemia is the condition in which the volume of the ECF compartment is expanded in relation to its capacitance. In most people, increments in Na + intake are matched by corresponding changes in Na + excretion as a result of the actions of the compensatory mechanisms detailed earlier (see section, “ Physiology ”). In these cases, no clinically detectable changes are observed. However, in the approximately 20% of the population who are salt-sensitive, the upward shift in ECF volume induced by high salt intake leads to a persistent rise in systemic arterial pressure, albeit without other overt signs of fluid retention (see Chapter 47 for a detailed discussion). In the following sections, the discussion is confined to the strict definition of hypervolemia, in which Na + retention is ongoing and inappropriate for the prevailing ECF volume, with the appearance of clinical signs of volume overload.
Etiology
The causes of hypervolemia can be conveniently divided into two major categories, primary renal Na + retention and secondary retention resulting from compensatory mechanisms activated as a result of disease in other major organs ( Table 15.6 ).
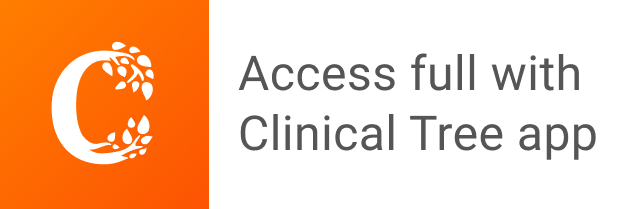