Chapter Outline
VASOPRESSIN—THE ANTIDIURETIC HORMONE, 282
THE TYPE 2 VASOPRESSIN RECEPTOR—A G PROTEIN–COUPLED RECEPTOR, 282
Interaction of Type 2 Vasopressin Receptor with Heterotrimeric G Proteins and β-Arrestin, 282
Fate of the Type 2 Vasopressin Receptor after Internalization—Delivery to Lysosomes, 284
Diabetes Insipidus (Central and Nephrogenic), 285
THE AQUAPORINS—A FAMILY OF WATER CHANNEL PROTEINS, 286
Other Permeability Properties of Aquaporins, 286
Aquaporin-2: The Vasopressin-Sensitive Collecting Duct Water Channel, 286
An Overview of Vasopressin-Regulated Aquaporin-2 Trafficking in Collecting Duct Principal Cells, 287
Use of In Vitro Systems to Examine Aquaporin-2 Trafficking and Function, 288
Expression of Multiple Basolateral Aquaporins (Aquaporin-2, Aquaporin-3, and/or Aquaporin-4) in Principal Cells, 289
A Role of Basolateral Aquaporin-2 in Cell Migration and Tubule Morphogenesis, 290
INTRACELLULAR PATHWAYS OF AQUAPORIN-2 TRAFFICKING, 291
Role of Clathrin-Coated Pits in Aquaporin-2 Recycling, 291
Aquaporin-2 Localization in Intracellular Compartments during Recycling, 291
Aquaporin-2 Is a Constitutively Recycling Membrane Protein, 291
REGULATION OF AQUAPORIN-2 TRAFFICKING, 291
Role of Kinases and A-Kinase Anchoring Proteins in Aquaporin-2 Trafficking, 292
Importance of the S256 Residue for Aquaporin-2 Membrane Accumulation, 293
Other Phosphorylation Sites (S261, S264, S269) Are Modified by Vasopressin, 293
Role of Phosphorylation in Exocytosis and Endocytosis of Aquaporin-2, 293
Phosphorylation of S256 Modulates Aquaporin-2 Interaction with Endocytotic Proteins, 293
Role of the Actin Cytoskeleton in Aquaporin-2 Trafficking, 294
Identification of Actin-Associated Proteins Potentially Involved in Aquaporin-2 Trafficking, 294
Microtubules and Aquaporin-2 Trafficking, 295
SNARE Proteins and Aquaporin-2 Trafficking, 295
LONG-TERM REGULATION OF WATER BALANCE, 296
Acquired Water Balance Disorders, 297
Lithium Treatment, 297
Electrolyte Abnormalities: Hypokalemia and Hypercalcemia , 299
Obstruction of the Urinary Tract, 299
Acute and Chronic Renal Injury, 299
Liver Cirrhosis and Congestive Heart Failure, 299
Novel Approaches for X-Linked Nephrogenic Diabetes Insipidus Therapy, 300
Vasopressin Receptor–Independent Membrane Insertion of Aquaporin-2—Potential Strategies for Treating Nephrogenic Diabetes Insipidus, 300
The small peptide hormone vasopressin (AVP) and its type 2 receptor (V 2 R) play a central role in the urinary concentrating mechanism. V 2 R activation results in the accumulation of a water channel, aquaporin-2 (AQP2), on the plasma membrane of collecting duct (CD) principal cells, thus increasing their permeability to water. This event permits the collecting duct luminal fluid to equilibrate osmotically with the surrounding interstitium in the kidney, resulting in water reabsorption and urine concentration. In humans the glomerulus filters approximately 180 L/day of fluid from plasma, of which 90% is returned to the circulation by reabsorption in the proximal tubule and descending limb of Henle’s loop. Most of the remaining 18 L is delivered to the CD system and is reabsorbed under the regulation of AVP. Dysfunction of this reabsorptive mechanism in the CD results in the production of large amounts of dilute urine, up to 18 L per day—a disease known as diabetes insipidus (DI). The antidiuretic hormone, AVP, plays a multifaceted role in the urinary concentrating process in mammals. In addition to activating the V 2 R and increasing plasma membrane AQP2 levels, AVP stimulates NaCl reabsorption by thick ascending limbs of Henle. AVP also stimulates urea transport in terminal portions of the CD, which allows high levels of urea to be excreted without reducing urinary concentrating ability. The urinary concentration mechanism therefore requires the tight coordination of cellular and molecular events within the context of renal architecture and fluid dynamics in the vasculature. This chapter will focus on the AVP-activated renal concentrating mechanism and will address how the V 2 R and the AQP2 water channel interact via intracellular signaling pathways to regulate CD water reabsorption and urine concentration.
Vasopressin—the Antidiuretic Hormone
The antidiuretic hormone of most mammals is a nine–amino acid peptide, AVP. Secretion of AVP from the posterior pituitary is stimulated by an increase in plasma osmolality, but also by a reduction in plasma volume (see Chapter 16 ). A change in osmolality as small as 1% can cause a significant rise in plasma AVP levels. AVP then activates regulatory systems necessary to retain water and restore osmolality to normal. In contrast, a 5% to 10% decrease in volume is required to stimulate AVP secretion, but AVP nevertheless has important clinical applications in the control of vasodilatory shock. The effects of AVP occur through the stimulation of receptors that are located on different cell types. The V 1 receptor activates a Ca ++ pathway and is involved in the pressor effect of AVP, whereas the V 2 R activates a cyclic adenosine monophosphate (cAMP) pathway that regulates transepithelial water transport in the kidney. In situations when it becomes critical to distinguish V 1 from V 2 receptor effects, a modified form of AVP, known as 1-desamino-8- d -arginine vasopressin(DDAVP) is used, which is specific for the V 2 R and has little or no V 1 -related pressor effect. The remainder of this discussion will focus on the V 2 R in renal epithelial cells.
The Type 2 Vasopressin Receptor—a G Protein–Coupled Receptor
The V 2 R is a member of the family of seven membrane-spanning domain receptors that couple to heterotrimeric G proteins (GPCRs). In the kidney it is expressed in segments from the thick ascending limb of the loop of Henle through to the CD principal cells. Most studies on V 2 R recycling, downregulation, and desensitization have been performed in cell culture using epitope-tagged V 2 R constructs. When AVP binds to the V 2 R, adenylyl cyclase (AC) activity is stimulated and cytosolic cAMP levels increase. This activates protein kinases, leading to the phosphorylation of several proteins, including AQP2. This water channel then accumulates in the apical plasma membrane of CD principal cells, thus increasing transepithelial water permeability and facilitating osmotically driven water reabsorption ( Figure 11.1 ). AQP2 contains several C-terminal residues whose phosphorylation status changes upon AVP treatment of V 2 R-expressing cells. Intracellular calcium is also increased by AVP via a mechanism involving calmodulin ; this is also involved in the regulated trafficking of AQP2.

Interaction of Type 2 Vasopressin Receptor with Heterotrimeric G Proteins and β-Arrestin
The V 2 R has been cloned from several mammalian species, and the sequences are more than 90% identical. Upon ligand binding, the V 2 R assumes an active configuration and the bound heterotrimeric G protein, Gs, dissociates into Gsα and Gsβγ subunits. This G protein is localized on the basolateral plasma membrane of the thick ascending limb of Henle, distal convoluted tubule, and CD principal cells. AC is stimulated by activated Gsα, and cAMP levels are increased. The predominant AC isoform in the adult rat kidney is AC-6, but several other AC isoforms are expressed at lower levels, including AC-4, AC-5, and AC-9 and calmodulin-sensitive AC-3. Knockout mice lacking the AC-6 isoform have significant nephrogenic DI (NDI), confirming its key role in the V 2 R-mediated signal transduction pathway. Liganded V 2 R interacts with Gs via its cytosolic domain, and a peptide corresponding to the third intracellular loop of the V 2 R inhibits signaling through Gs.
The AVP/V 2 R association increases cellular cAMP, which initiates a cascade of events that increases CD water permeability by changing the phosphorylation pattern of AQP2 and causing its accumulation at the cell surface. Termination of this response depends on the internalization of the V 2 R after AVP binding and its delivery to and degradation in lysosomes ( Figure 11.2 A and D ). Many accessory proteins are involved in V 2 R downregulation, including inhibitory G i proteins, proteins involved in clathrin-mediated endocytosis, and proteins of the so-called retromer complex. In addition to less receptor at the cell surface, the level of V 2 R messenger RNA (mRNA) also decreases rapidly after elevation of plasma AVP. Additional mechanisms that downregulate the AVP response include destruction of cAMP by cytosolic phosphodiesterases and inhibition by prostaglandins, dopamine, adenosine receptor stimulation, adrenergic agonists, endothelin-1, bradykinin, and epidermal growth factor. However, data have shown that cAMP levels in AVP target cells remain elevated for a considerable time after stimulation, and that the V 2 R continues to signal from endosomes after internalization.

Changes in receptor conformation after AVP binding are followed by receptor phosphorylation, desensitization, internalization, and sequestration. One critical step in this process is the binding of β-arrestin to the V 2 R, which is triggered by phosphorylation of the V 2 R by kinases, including G protein–coupled receptor kinases (GRKs). Interestingly, different cellular responses to receptor phosphorylation can be dissected depending on the kinase that is involved in the phosphorylation process. Thus phosphorylation of V 2 R with GRK2 and GRK3 results in AVP-dependent desensitization and recruitment of β-arrestins. In contrast, GRK5 and GRK6 phosphorylation is involved in extracellular signal–regulated kinase activation.
Arrestin-receptor complexes recruit the clathrin adaptor protein AP-2, an important component of the endocytotic mechanism, and the complex is then internalized via clathrin-mediated endocytosis. Arrestins also uncouple GPCRs from heterotrimeric G proteins, producing a desensitized receptor. After downregulation, restoration of prestimulation levels of V 2 R at the cell surface requires several hours. In contrast, prestimulation levels of the β 2 -adrenergic receptor (β 2 AR) are restored on the cell surface within an hour of internalization. This difference has been correlated with the association characteristics of the receptor/β-arrestin complex. The V 2 R forms a more stable interaction with β-arrestin than the β 2 AR. This interaction is responsible for the intracellular retention of the V 2 R and its continued signaling from intracellular vesicles, but it does not dictate the final cellular destination of the receptor.
Fate of the Type 2 Vasopressin Receptor after Internalization—Delivery to Lysosomes
After AVP stimulation, there is a rapid β-arrestin–dependent ubiquitination of the V 2 R, followed by internalization and increased degradation. Much of the V 2 R that is internalized with the AVP ligand enters a lysosomal degradation compartment. Real-time microscopy of transfected LLC-PK 1 cells shows that after ligand binding, V 2 R–green fluorescent protein (GFP) moves along microtubules ( Figure 11.2 E ) to perinuclear late endosomes and lysosomes for degradation. Restoration of prestimulation levels of the V 2 R at the cell surface is greatly inhibited by cycloheximide, indicating that new protein synthesis is involved in the resensitization process. However, the V 2 R can continue to signal even after internalization, because of the presence of the requisite array of signal transduction proteins on endosomes. This can extend the duration of cAMP elevation in cells, in contrast to oxytocin, which stimulates a cAMP pulse of much shorter duration. This prolonged cAMP signaling via the V 2 R may be an adaptation to the unusually harsh (high osmolality, low pH) conditions in the renal medulla, which, combined with low circulating levels of AVP that allow only low receptor occupancy, might otherwise lead to only a small cAMP response.
In summary, the V 2 R—classified as a “slow-recycling” GPCR—continues to signal after internalization and is then largely degraded in lysosomes. This pathway may allow the V 2 R to function in the harsh environment of the renal medulla, which can be acidic and of high osmolality. Receptor-ligand pairs often separate in the acidic environment of endosomes, but AVP must actually associate with the V 2 R in the acidic renal medulla, indicating that it is at least partially resistant to pH-induced dissociation. Delivery of both the ligand and receptor to lysosomes may be required to terminate the physiologic response to AVP.
Diabetes Insipidus (Central and Nephrogenic)
DI is characterized by excessive urinary water loss (up to 18 L of dilute urine/day) via the kidneys. Central DI (CDI), results from loss of AVP, whereas in NDI the kidneys no longer respond to circulating AVP. Both types can be either acquired or inherited. Failure of water reabsorption in the CD is associated with most cases of DI. If not detected and corrected early in life, DI can result in severe dehydration and damage to the central nervous system, hypernatremia, and bladder enlargement. The most common cause of NDI is in bipolar patients treated with lithium, which results in NDI in approximately 20% of treated patients. Other acquired causes of NDI include hypokalemia, hypercalcemia, and ureteral obstruction. Both acquired and congenital forms of NDI have been linked to defects in AVP signaling and AQP2 trafficking. Although the inherited forms of DI are much rarer, their molecular basis has been largely elucidated following identification and characterization of the key proteins involved: the V 2 R, the AVP-sensitive CD water channel, AQP2, and the gene coding for the AVP/neurophysin/glycopeptide precursor protein from which active AVP is generated. Chapters 16 and 45 provide more extensive details on the pathophysiology of this disease, and several review articles have covered this topic in depth.
Central (Neurohypophyseal) Diabetes Insipidus
CDI results from a defect in the production and release of functional AVP. Acquired CDI is often idiopathic but can also result from damage to the AVP-producing and AVP-secreting cells in the hypothalamus/pituitary, for example from trauma or infection. Hereditary forms of familial diabetes insipidus have been linked to over 66 different mutations of the gene encoding the AVP-neurophysin II precursor. Absence of functional AVP can be generally treated by administration of AVP or DDAVP, usually via nasal aerosol. However, AVP is also involved in stimulating AQP2 transcription; AQP2 levels are significantly lower than normal in Brattleboro rats—a valuable, but now difficult to obtain, animal model of CDI that does not produce functional AVP because of a single amino acid mutation in the neurophysin domain of the AVP gene. It is likely, therefore, that the beneficial effect of AVP in CDI results from increased sodium reabsorption in the thick ascending limb of Henle, increased AQP2 trafficking (see later), and increased AQP2 protein levels following transcriptional activation of the AQP2 gene.
Nephrogenic Diabetes Insipidus
The loss of an appropriate renal response to AVP in NDI usually reflects a functional defect in either the V 2 R or AQP2 protein. Administration of AVP is not, therefore, usually sufficient to rectify the concentrating defect. However, the mutated V 2 R may have a reduced affinity for AVP in some patients, and they may respond if circulating AVP levels are increased sufficiently.
NDI can be of a rare congenital/hereditary nature or, more commonly, an acquired disease. A variety of gene mutations that result in defective targeting and/or function of the V 2 R or the AQP2 water channel result in several distinct forms of NDI. The predominant form (type 1) affecting the V 2 R is an X-linked recessive trait resulting from mutations in the AVP receptor type 2 gene (AVPR2). Mutations in AVPR2 represent approximately 90% of hereditary NDI cases. Over 225 mutations that result in NDI have been identified in the V 2 R protein, and these are discussed in more detail in Chapter 45 . These mutations can be divided into five classes. Class I mutations lead to improperly processed/unstable mRNA, frameshifts, or nonsense mutations resulting in truncation of the receptor. Class II mutations result in misfolding of the receptor and retention in the endoplasmic reticulum (ER). Class III mutations cause misfolding of the V 2 R, reduced interaction of the V 2 R with G proteins, and impaired cAMP production. Class IV mutations also lead to misfolding, with the V 2 R able to reach the plasma membrane yet unable to interact properly with AVP. Class V mutations are missorted to an incorrect cellular compartment. Novel approaches for X-linked NDI therapy are discussed later in this chapter.
Type II NDI is a much less common autosomal recessive disease caused by mutations in the AQP2 gene, representing approximately 10% of NDI cases. Currently 49 mutations in the AQP2 gene, resulting in two different molecular outcomes, have been described. First, mutations in AQP2 can affect the routing of functional AQP2 to the membrane. Second, some AQP2 mutations result in a defect in the formation of the pore-forming structure of AQP2, resulting in a lack of water channel function. In addition to these recessive forms, 10% of autosomal NDI cases are inherited in a dominant manner. The defect in these cases is due to mutations in the C-terminal tail of AQP2, which is essential for correct intracellular routing of the channel. In this case, heterotetramers of AQP2 monomers may be formed between the wild-type and the mutated form, causing misrouting of AQP2, retention in the Golgi apparatus, or sorting of AQP2 to late endosomes, lysosomes, or the basolateral plasma membrane. Thus the mutations act by a dominant negative mechanism. Because the condition is only partial, some wild-type AQP2 forms functional homotetramers, and fluid restriction or desmopressin (DDAVP) administration increases urine osmolality except in severe cases.
Although specific treatments for non–X-linked NDI are not available, various novel approaches have been proposed (see later). In addition, therapeutic guidelines include the use of a low-salt diet, combined with hydrochlorothiazide and amiloride treatment. In addition, inhibitors of cyclo-oxygenases, such as indomethacin, are often prescribed, despite their involvement in gastrointestinal disturbances.
The Aquaporins—a Family of Water Channel Proteins
A total of 12 mammalian aquaporin homologs are known, and dozens more have been identified in virtually all organisms, including invertebrates, plants, and microbes. The first water channel (AQP1) was identified in 1991 by Peter Agre and his associates and the CD AVP-sensitive water channel, AQP2, was cloned in 1993. AQP1 is expressed in many cells and tissues with high constitutive water permeability, including proximal tubules and thin descending limbs of Henle’s loop in the kidney. AQP2 is the principal cell water channel that is involved in AVP-regulated urinary concentration in the kidney. The membrane topography and some key features (e.g., phosphorylation sites) of AQP2 are illustrated in Figure 11.3 .

Other Permeability Properties of Aquaporins
The single channel water permeability of different aquaporins varies greatly, and some aquaporins, including AQP3, AQP8, and AQP9—referred to as aquaglyceroporins—even allow the passage of molecules, including urea, glycerol, ammonia, and other small solutes. However, all aquaporins are impermeable to protons, a property that was first demonstrated using isolated apical endosomes from rat kidney papilla. Crystallographic evidence has shown that the central region of the aqueous channel with charged residues lining a constricted region renders the passage of protons energetically unfavorable. Physiologically the impermeability of aquaporins, including AQP2, to protons is important because the CD luminal fluid is acidic due to proton secretion and can reach a pH close to 4.5. It would be problematic if the cytosol of the principal cells equilibrated with the low pH of the tubule lumen simultaneously with an increase in apical membrane water permeability.
Aquaporin-2: the Vasopressin-Sensitive Collecting Duct Water Channel
AQP2 is the AVP-regulated water channel in kidney CD principal cells. AVP stimulation of the kidney CD results in the accumulation of AQP2 on the plasma membrane of principal cells ( Figure 11.4 ). This involves the recycling of AQP2 between intracellular vesicles and the cell surface. However, both AQP3 and AQP4, which are found in the basolateral membrane of principal cells, are also regulated at the expression and possibly functional level by AVP and/or dehydration.

The AVP-induced change from a low-to-high permeability state of CD principal cells, and vice versa, involves the reversible redistribution of AQP2 from cytoplasmic vesicles to the apical plasma membrane. Because the basolateral membrane of these cells always has high water permeability due to the presence of AQP3 and/or AQP4, the luminal fluid then equilibrates osmotically with the surrounding interstitium. The osmolality in the renal inner medulla reaches approximately 1200 mOsm/kg in humans, and the urine can reach the same concentration in the presence of AVP. However, this mechanism also functions in the cortical CDs, in which a lumen (approximately 100 mOsm/kg) to interstitial (approximately 300 mOsm/kg) osmotic gradient results from NaCl extraction (luminal dilution) in the thick ascending limbs of Henle. Approximately 60% of the distally delivered water load is reabsorbed in the cortical CD system, or approximately 12 L/day.
An Overview of Vasopressin-Regulated Aquaporin-2 Trafficking in Collecting Duct Principal Cells
Early freeze-fracture electron microscopy studies using amphibian urinary bladder and skin suggested that clusters of water channels are located on intracellular vesicles that fuse with the apical plasma membrane upon AVP stimulation. The water channels are internalized back into the cell by endocytosis after AVP washout. Following the identification of aquaporins, specific antibodies allowed direct testing of the mechanism by which membrane water permeability is increased in AVP target cells, both in the kidney and in cell culture models. AQP2 is located in the apical plasma membrane of CD principal cells, as well as in intracellular vesicles. In vitro and in vivo studies correlated the AVP-stimulated increase in CD water permeability and urinary concentration with relocalization of AQP2 from intracellular vesicles to the plasma membrane of principal cells (see Figure 11.4 ). This relocation was reversible upon AVP washout and in animals either infused with a V 2 R antagonist or subjected to water loading to reduce circulating AVP levels. These and other data indicated that AVP acutely regulates the osmotic water permeability of CD principal cells by inducing a reversible shift in the steady state distribution of AQP2. One unexpected observation from initial studies was that significant amounts of AQP2 were present on principal cell basolateral membranes in some kidney regions, and that this staining tended to increase after AVP treatment. This issue will be addressed in more detail later in this chapter.
The internalized AQP2 that accumulates in endosomes after AVP withdrawal follows a complex intracellular pathway before reinsertion into the plasma membrane. Recycling of the existing cohort of AQP2 can occur even when protein synthesis is inhibited, indicating that de novo protein synthesis is not required for sequential responses to AVP stimulation. However, not all AQP2 is recycled. A significant amount of AQP2 also accumulates in multivesicular bodies (MVBs) after treatment of rats with an AVP antagonist. This pool of AQP2 can then be directed to lysosomes for degradation, be transferred to a recycling compartment, or be directly transported to the cell surface via transport vesicles that derive from the MVBs. The fate of internalized AQP2 seems to be at least in part regulated by ubiquitinylation, and this pathway will be discussed later.
At least some of the MVBs can fuse with the apical membrane of principal cells and release small vesicles known as exosomes into the tubule lumen. These exosomes contain AQP2 on their limiting membranes, as well as AQP2 mRNA and many other mRNAs and microRNAs within their lumen. AQP2 protein can be detected in urine, and the amount increases in conditions of antidiuresis, when more AQP2 is present in the apical membrane of principal cells. The physiologic relevance, if any, of this urinary excretion of AQP2 and other membrane proteins in exosomes remains unknown, but the amount of exosomal AQP2 can be increased by AVP and urinary alkalinization. It has also been shown that exosomes from DDAVP treated rats can induce increased AQP2 expression and water permeability in mCCD11 cells in culture, implying a role in cell-cell communication. Interestingly, urinary AQP2 correlates with the severity of nocturnal enuresis in children, and lowering urinary calcium levels (by a low-calcium diet) has a beneficial effect in reducing the severity of the enuresis and reducing AQP2 secretion in hypercalcemic children treated with DDAVP.
Use of in Vitro Systems to Examine Aquaporin-2 Trafficking and Function
Expression of Aquaporins in Xenopus Oocytes
Xenopus oocytes were the first model protein expression system that allowed the identification of AQP1 as a functional water channel using an oocyte swelling assay. This system has also been valuable in assessing the function and regulation of mutated aquaporins and addressing the role of oligomerization and phosphorylation of both wild-type and mutated AQP2 on its function. In this way, many of the mutant AQP2 proteins resulting in NDI were shown to have folding defects that cause retention and ultimate degradation in the rough endoplasmic reticulum and/or retention in the Golgi.
Expression of Aquaporins in Nonepithelial Cells
Chinese hamster ovary (CHO) and other nonpolarized cells, although not being appropriate models for studying the polarized expression of aquaporins in epithelia, can be used to measure functional and physical properties of aquaporins. For example, freeze-fracture studies on CHO cells revealed that AQP1 assembles in the lipid bilayer as a tetramer, in agreement with biochemical cross-linking data and cryomicroscopy and atomic force microscopy. Important information was gathered concerning the abnormal intracellular location and the defective functional activity of AQP2 mutations. CHO cells were also used to demonstrate that chemical chaperones could increase the delivery of misfolded AQP2 protein to the cell surface.
Transfected Polarized Cells Expressing Exogenous Aquaporin-2
Several laboratories developed stably transfected cells to dissect AQP2 trafficking and V 2 R signaling. These include LLC-PK 1 cells ( Figure 11.5 ), rabbit CD epithelial cells, Madin-Darby canine kidney (MDCK) cells, and primary cultures of inner medullary CD (IMCD) cells. Transfected LLC-PK 1 and MDCK cells showed constitutive plasma membrane expression of AQP1, similar to its pattern of expression in vivo, whereas AQP2 accumulation at the cell surface was increased by AVP or forskolin. Similar data were obtained using transformed rabbit CD epithelial cells. Since their initial development, AQP2-expressing cultured cell lines have proven to be reliable cell models that in most cases predict the in vivo behavior of the AVP-stimulated AQP2 trafficking pathway.

Cells Expressing Endogenous Aquaporin-2
Some cell lines, including mpkCCD(cl4) cells, express endogenous AQP2 and can be used to examine factors regulating AQP2 transcription. These include studies on the tonicity-responsive enhancer–binding protein (TonEBP) and the nuclear factor-kappaB pathway regulating AQP2 expression in response to hypertonicity and lipopolysaccharide, showing that the effect of lithium on AQP2 downregulation was unrelated to AC activity, demonstrating the role of exchange protein activated by cAMP (Epac) in long-term regulation of AQP2 transcription, and showing that angiotensin II increases AQP2 protein expression. Other studies using mpkCCD cells to address issues of AQP2 expression and trafficking have also used this cell line.
Use of Kidney Tissue Slices and Isolated Collecting Duct to Examine Aquaporin-2 Trafficking
Kidney slices and isolated tubules have been extremely valuable tools to study AQP trafficking. Although there may be potential issues of oxygen, nutrient, and drug diffusion into 150- to 200-µm–thick slices, dozens of samples can be prepared from the same kidney and treated simultaneously in paired studies. Isolated perfused tubules provide invaluable data, but the procedure is technically demanding and is accessible to only a few laboratories. Finally, isolated, microdissected CDs in suspension have been exploited as a system to study AQP2 recycling, as well as to provide a pure IMCD cell population for detailed proteomic analysis of IMCD cells before and after exposure to AVP.
Expression of Multiple Basolateral Aquaporins (Aquaporin-2, Aquaporin-3, And/or Aquaporin-4) in Principal Cells
The presence of AQP3 and/or AQP4 renders the basolateral plasma membranes of CD principal cells constitutively permeable to water. AQP3 expression is predominant in the cortex and decreases toward the inner medulla, with the reverse pattern for AQP4, which is most abundant in the inner medulla. Interestingly, AQP2 is also localized in the basolateral plasma membrane of these cells in some regions of the CD. The bipolar expression of AQP2 is most evident in the cortical connecting segment ( Figure 11.6 A ) and the inner medulla ( Figure 11.6 B ) but is also detectable in other regions, including the outer medullary CD. An example of AQP2 and AQP4 staining in the same principal cells from an outer medullary CD is shown in Figure 11.7 . In the inner medulla, basolateral expression of AQP2 is greatly increased by AVP (see Figure 11.6 B ) and oxytocin, with hypertonicity in the medulla playing a modulating role. Basolateral AQP2 expression is increased by long-term (6 days) aldosterone or AVP treatment of rats in the cortical CD. Importantly, basolateral membrane water permeability in this region is increased in a mercurial-sensitive manner by AVP treatment, ruling out the contribution of the mercurial-insensitive AQP4 to this process.


A frameshift mutation in AQP2 that results in NDI in humans also results in basolateral targeting when the mutated protein is expressed in polarized MDCK cells. This shows that an increased basolateral expression of AQP2 is not sufficient to increase transepithelial water permeability in the CD. Based on new data (see later), it now appears that the basolateral AQP2 represents a transient step in an indirect apical targeting pathway for the AQP2 protein.
A Role of Basolateral Aquaporin-2 in Cell Migration and Tubule Morphogenesis
Because other water channels are basolaterally localized, the additional role of basolateral AQP2 in principal cell water transport remains unclear. However, studies have suggested that basolateral AQP2 may have a role in cell migration and tubulogenesis. The extracellular domain of AQP2 contains an arginine-glycine-aspartic acid (RGD) domain that is involved in interaction with some integrins. Many transgenic or knockout mice that lack AQP2 expression have a defect in kidney structure, most evident in the medulla, which can lead to premature death ( Figure 11.8 ). These phenotypes are similar to those seen in a β1 integrin knockout mouse. The structural alterations in AQP2 knockout mice were previously attributed to increased urine flow due to an AQP2-related concentrating defect. However, based on analysis of the phenotypes of various mice with NDI, the kidney structural defects may instead be due to the absence of normal AQP2/integrin/extracellular matrix interactions during renal development and perhaps adulthood. This morphogenic effect seems to be independent of the water-transporting activity of AQP2. In contrast, the effects of AQP1 expression on cell migration were attributed to its role in increasing membrane water permeability.

Intracellular Pathways of Aquaporin-2 Trafficking
AQP2 is continually (constitutively) recycling between intracellular vesicles and the cell surface even in the absence of AVP stimulation. Membrane accumulation of AQP2 can then be achieved by a combination of stimulation of exocytosis and inhibition of endocytosis. This pattern of trafficking has been described for some other membrane transporters, including the insulin-regulated glucose transporter 4 (GLUT4) and cystic fibrosis transmembrane conductance regulator (CFTR).
Role of Clathrin-Coated Pits in Aquaporin-2 Recycling
Clathrin-coated pits concentrate and internalize many plasma membrane proteins, including receptors, transporters, and channels. Clathrin-coated pits are critical for the internalization of both AQP2 and the V 2 R. When clathrin-mediated endocytosis is inhibited by the expression of a mutated dominant negative form of the protein dynamin in LLC-PK 1 cells, AQP2 accumulates on the plasma membrane and is depleted from cytoplasmic vesicles. Dynamin is a GTPase that is involved in the formation and pinching off of clathrin-coated pits to form clathrin-coated vesicles. The cholesterol-depleting drug methyl-β-cyclodextrin (MBCD) also blocks clathrin-mediated endocytosis. Exposure of both AQP2-expressing cell cultures and intact kidneys (using an isolated perfused kidney preparation) to MBCD results in AQP2 accumulation on the plasma membrane within 15 minutes ( Figure 11.9 ). Taken together, these data demonstrate the central role of clathrin-coated pits in AQP2 endocytosis. A later study proposed a role for caveolae as an alternative endocytotic pathway for AQP2 in cultured cells, but under normal conditions, caveolae and caveolin are not present on the apical pole of principal cells in vivo. Therefore, if caveolae are indeed involved in apical endocytosis in vitro, this is probably an artifact of cell culture. It has already been shown that several endocytotic events attributed to caveolae in proximal tubular cell cultures are not relevant to the in vivo situation. Proximal tubules do not express caveolin under normal conditions, but expression is induced in proximal tubular cell cultures.

Aquaporin-2 Localization in Intracellular Compartments during Recycling
Recycling of AQP2 was directly demonstrated in cycloheximide-treated, AQP2-transfected LLC-PK 1 cells, in which several rounds of exocytosis and endocytosis of AQP2 occurred despite the inhibition of de novo AQP2 synthesis. After internalization via clathrin-coated pits, AQP2 enters an early endosomal antigen 1 (EEA1)–positive compartment. This subapical recycling compartment is distinct from organelles such as the Golgi, the trans-Golgi network (TGN) and lysosomes, does not contain transferrin receptor, and is distinct from vesicles that contain GLUT4 (another recycling protein) in adipocytes that coexpress AQP2 and GLUT4. Whether vesicles containing recycling AQP2 represent a novel “organelle” or whether AQP2 usurps an already existing pathway in cultured cells and modifies it based on intrinsic signals within the AQP2 sequence remains unclear. It is probable that as newly synthesized AQP2 is loaded into transporting vesicles as it exits the TGN, the fate of the vesicles is indeed determined by signals on the AQP2 protein itself.
However, when recycling of AQP2 is interrupted by lowering the incubation temperature of the cells to 20° C, or by incubating cells with bafilomycin (an inhibitor of the vacuolar hydrogen ion adenosine triphosphatase [H + -ATPase]), AQP2 can be concentrated in a clathrin-positive, Golgi-associated compartment. This accumulation occurs even in the presence of cycloheximide, indicating that recycling AQP2 is also accumulating in this juxtanuclear compartment. The 20° C block prevents exit of proteins from the TGN, and clathrin-coated vesicles are enriched in this cellular compartment. However, some portions of the so-called recycling endosome, which is located in a similar juxtanuclear region of some cells, also have clathrin-coated domains. Therefore, the AQP2 could be recycling either via the TGN, through a specialized clathrin-coated recycling endosome, or both. Indeed recycling AQP2 is partially co-localized with rab11, a marker of the recycling endosomal compartment, in subapical vesicles.
Aquaporin-2 is a Constitutively Recycling Membrane Protein
As discussed earlier, AQP2 recycles continually between intracellular vesicles and the cell surface, both in cultured cells and in principal cells in situ . This provides the opportunity to modulate the plasma membrane content of AQP2 by increasing the rate of exocytosis, decreasing endocytosis, or both. Such a dual action of AVP was predicted by Knepper and Nielsen by comparing mathematical models of AVP-induced permeability changes to experimental data from perfused CDs. Constitutive recycling was subsequently shown experimentally by blocking the AQP2 recycling pathway either in an intracellular perinuclear compartment identified as the TGN as discussed earlier, or at the cell surface. When clathrin-mediated endocytosis is arrested using dominant negative dynamin or MBCD, AQP2 accumulates at the plasma membrane in an AVP-independent manner (see Figure 11.9 ). Importantly, MBCD also causes a rapid and significant accumulation of AQP2 in the apical membrane of CD principal cells in situ (see Figure 11.9 E and F ). This observation raises the possibility that inhibition of endocytosis is a potential pathway by which AQP2 can be accumulated at the cell surface of CD principal cells in patients with X-linked NDI.
Regulation of Aquaporin-2 Trafficking
Our understanding of AQP2 recycling continues to evolve in parallel with new discoveries related to the targeting and trafficking of membrane proteins in general. These include the discovery of alternative signaling pathways for AQP2 trafficking in addition to the “conventional” cAMP pathway, the role of phosphorylation by various kinases, the involvement of the actin cytoskeleton, and the gradual discovery of accessory interacting proteins, including phosphorylation-dependent AQP2-binding proteins.
Role of Kinases and a-Kinase Anchoring Proteins in Aquaporin-2 Trafficking
Phosphorylation of the C-terminal tail of AQP2 plays a complex regulatory role in trafficking and compartmentalization of the protein. AQP2 contains several putative phosphorylation sites for kinases (see Figure 11.3 ), including protein kinase A (PKA), protein kinase G (PKG), protein kinase C (PKC), Golgi casein kinase, and casein kinase II. The majority of work has focused on the role of PKA-induced phosphorylation of S256 in the AVP-induced signaling cascade, because this site is critical for the AVP-induced membrane accumulation of AQP2. However, S256 is part of a polyphosphorylated region at the C terminus of AQP2, which contains three further AVP-regulated phosphorylation sites: S261, S264, and S269 (threonine in humans), as well as a threonine at position T244. The rise in intracellular cAMP following activation of AC by AVP/ V 2 R results in recruitment of PKA to AQP2-containing vesicles by A-kinase-anchoring proteins (AKAPs). The co-localization of vesicular AQP2 with AKAP 18δ makes this the most likely isoform to mediate this event. Inhibition of the cAMP-specific phosphodiesterase 4D (PDE 4D ) with rolipram increases AKAP-tethered PKA activity in AQP2-bearing vesicles and enhances AQP2 trafficking, indicating that a novel, compartmentalized cAMP-dependent signal transduction pathway consisting of anchored PDE 4D , AKAP18δ, and PKA plays an essential role in AQP2 translocation. Furthermore, AKAP Ht31 directly interacts with the actin-modifying GTPase RhoA, which plays a crucial role in modulating AQP2 trafficking (see later).
Importance of the S256 Residue for Aquaporin-2 Membrane Accumulation
Phosphorylation is certainly involved in the regulated accumulation of AQP2 in the plasma membrane; phosphorylation of S256 and S269 prevent or reduce AQP2 endocytosis (see later). Whether the actual water permeability of AQP2 is also modulated by phosphorylation—by analogy with some of the plant aquaporins and AQP4 —is controversial. In addition, PKA-dependent phosphorylation of AQP2 in purified endosomes had no effect on single channel water permeability. In contrast, regulation of membrane permeability by AQP2 trafficking has been established in a variety of experimental systems. Following PKA activation, phosphorylation of AQP2 on S256 is critical for AVP-induced cell-surface accumulation of AQP2 (see Figure 11.5 ). In oocytes, S256 phosphorylation is required for AQP2 trafficking to the plasma membrane. A mouse strain with an amino acid substitution at S256 (S256L), which prevents phosphorylation of this residue and inhibits AQP2 accumulation on the plasma membrane, has polyuria and congenital progressive hydronephrosis. The importance of S256 phosphorylation was shown in humans by the identification of a mutation in AQP2 (S254L), which destroys the PKA phosphorylation site at S256 and results in NDI.
Other Phosphorylation Sites (S261, S264, S269) are Modified by Vasopressin
The role of three other phosphorylation sites in the AQP2 C terminus, S261, S264, and S269, remains uncertain, although the pS269 form of AQP2 is exclusively detected in the apical plasma membrane, suggesting a regulatory role of this phosphorylation site directly in the plasma membrane, perhaps by inhibiting endocytosis. S256 seems to be the “master” phosphorylation site, because downstream phosphorylation of S264 and S269 requires prior PKA-mediated phosphorylation of S256, whereas S261 phosphorylation is independent of any of the other sites. Acute AVP exposure normally decreases the abundance of pS261 but increases that of both pS264 and pS269. All three phosphorylated forms are localized to some degree in the plasma membrane in vivo, but cell-culture studies have demonstrated that S261 phosphorylation is not required for either AVP-stimulated AQP2 trafficking or constitutive recycling. Preventing dephosphorylation of AQP2 with the phosphatase inhibitor okadaic acid increases cell surface accumulation of AQP2 in cultured cells, although this effect was also unexpectedly found in the presence of the kinase inhibitor H89, suggesting an effect that is not dependent on AQP2 phosphorylation by PKA. However, there is a complex interaction between S261 phosphorylation and AQP2 ubiquitinylation that plays a role in modulating AQP2 trafficking. As for other proteins with multiple kinase target sites, dissecting their individual contributions to AQP2 trafficking is likely to be a complex and difficult process, made even more complicated by the superimposition of AQP2 ubiquitinylation events that contribute to the regulation of AQP2 trafficking.
Role of Phosphorylation in Exocytosis and Endocytosis of Aquaporin-2
Although phosphorylation has clearly been shown to inhibit endocytosis of AQP2, its role in exocytosis is much less clear. An S256A mutant, from which the PKA phosphorylation site is absent, readily accumulates on the plasma membrane upon inhibition of endocytosis with either K44A dynamin or MBCD (see Figure 11.9 ), suggesting that the exocytotic pathway is intact under these conditions. Using a fluorescence-based exocytosis assay, Nunes and associates showed that AVP increases exocytosis of vesicles in AQP2-expressing cells whether or not AQP2 is phosphorylated at S256. Thus, although AVP-induced accumulation of AQP2 at the cell surface requires S256 phosphorylation, exocytotic insertion of AQP2 into the plasma membrane is probably independent of this phosphorylation event.
Although phosphorylation of AQP2 is usually required for AVP-induced cell surface expression of AQP2, the internalization of AQP2 may not be dependent on its phosphorylation state at S256. Prostaglandin E 2 (PGE 2 ) stimulates removal of AQP2 from the surface of principal cells when added after AVP treatment but does not appear to alter the S256 phosphorylated state of AQP2. This conclusion was based on whole-cell examination, however, and it remains possible that the cohort of AQP2 that is internalized does in fact undergo dephosphorylation. Resolving this issue will require immunolabeling of individual endocytotic events/vesicles, either by electron microscopy or superresolution fluorescence imaging. However, the S256D-AQP2 mutant—which mimics a phosphorylated S256 residue—is constitutively expressed predominantly at the cell surface, and internalization can be induced by either PGE 2 or dopamine, but only after preexposing cells to forskolin. This suggests that PGE 2 and dopamine induce internalization of AQP2 independently of AQP2 S256 dephosphorylation, but that preceding activation of cAMP production is necessary for PGE 2 and dopamine to cause AQP2 internalization. These data imply that phosphorylation of another intracellular target(s) (presumably by forskolin-stimulated elevation of cAMP) is necessary for AQP2 endocytosis to occur (see later). However, other data suggest that the effect of PGE 2 on AQP2 recycling depends on which PGE 2 receptor (EP receptor) it acts upon, because EP2 and EP4 receptor stimulation is able to increase intracellular cAMP levels and presumably AQP2 membrane insertion. In a similar manner, the fact that AVP-induced exocytosis of AQP2 also seems to be independent of S256 phosphorylation also suggests that AVP acts on other targets within the cell to stimulate vesicle exocytosis. One such target is the actin cytoskeleton (see later).
Phosphorylation of S256 Modulates Aquaporin-2 Interaction with Endocytotic Proteins
The mechanism by which phosphorylation of AQP2 affects the steady-state redistribution of AQP2 is slowly being unraveled. One report has suggested that a Golgi casein kinase–mediated phosphorylation of S256 is necessary for the passage of AQP2 through the Golgi apparatus in its biosynthetic pathway. However, this conclusion is not supported by studies showing that S256A AQP2 can recycle and accumulate at the cell surface when endocytosis is inhibited. Nonetheless, accumulating evidence suggests that phosphorylation of AQP2 mediates its interaction with other proteins. For example, phosphorylation modifies AQP2 interaction with key proteins of the endocytotic machinery, including heat shock cognate/heat shock protein 70 (hsc/hsp70). AQP2 is present in “endocytosis-resistant” membrane domains after AVP treatment, probably because the interaction of AQP2 with hsc/hsp70 and other key proteins, such as dynamin and clathrin itself, that are required for clathrin-mediated endocytosis is greatly reduced by phosphorylation of AQP2 at S256. AQP2 membrane accumulation was increased in cells expressing a dominant negative mutation of hsc70, which blocks clathrin-mediated endocytosis. A comparative proteomic approach determined that several proteins, including hsp70 isoforms 1, 2, and 5 (hsp70-1, hsp70-2, hsp70-5), and annexin 2, differentially interact with AQP2 depending on its phosphorylation status. The myelin and lymphocyte protein (MAL) is an AQP2-interacting protein that enhances AQP2 cell surface accumulation by reducing AQP2 internalization. MAL associates less with AQP256A than with wild-type AQP2.
Role of the Actin Cytoskeleton in Aquaporin-2 Trafficking
It has been appreciated for many years that the actin cytoskeleton is important for AQP2 trafficking. G-actin associates directly with non–S256-phosphorylated AQP2, and both β- and γ-actin are associated with AQP2-containing vesicles. Early studies conducted in rat inner medulla and toad urinary bladder demonstrated that AVP induces a reduction in F-actin in apical regions of cells in parallel with the accumulation of water channels on apical membranes. Later studies in mpkCCD14 cells have confirmed these findings and demonstrated that in response to AVP, F-actin disappears from near the center of the apical plasma membrane while consolidating laterally near the tight junction in a calcium-dependent manner. Furthermore, AVP-induced apical AQP2 trafficking and forskolin-induced water permeability increases were blocked by F-actin disruption.
The potential role of actin in AQP2 trafficking was directly examined in transfected cells in culture. Exposure of cells to Clostridium toxin B, which inhibits Rho GTPases that are involved in regulating the actin cytoskeleton, resulted in actin depolymerization and accumulation of AQP2 in the plasma membrane. AQP2 translocation was also seen in cells treated with the downstream Rho kinase inhibitor, Y-27632. Conversely, expression of constitutively active RhoA in these cells induced stress fiber formation, indicating actin polymerization, and inhibited the AQP2 translocation in response to forskolin. The actin cytoskeleton has varied and complex effects on several aspects of vesicle trafficking, including an involvement in endocytosis in some systems. This mechanism is probably responsible for the effects of actin depolymerization on AQP2 membrane accumulation.
Noda and colleagues showed that AQP2 phosphorylation results in release of AQP2 from G-actin and an increased affinity of AQP2 for tropomyosin 5b (TM5b). This causes a reduction of TM5b bound to F-actin, inducing F-actin destabilization and subsequent AQP2 trafficking to the plasma membrane. These findings also suggest a novel mechanism of protein trafficking in which the channel protein itself critically regulates local actin reorganization to initiate its movement. This idea is supported by data showing that AVP induces a burst of exocytosis only in cells expressing AQP2, and not in cells that are AQP2 null. In addition, AVP induces significant actin depolymerization in LLC-PK 1 cells and MDCK only when they express AQP2. Thus the presence of AQP2 influences the cellular effect of AVP on target cells, despite the fact that cAMP levels are similarly increased under all conditions. Further evidence for the involvement of actin in AQP2 trafficking comes from work using statins. After acute application to cells, statins cause the inhibition of RhoA, actin depolymerization, and AQP2 membrane accumulation due to inhibition of endocytosis.
In contrast to the data showing membrane accumulation of AQP2 after actin depolymerization, a study using transfected MDCK cells reported that AQP2 was concentrated in an EEA1-positive early endosomal compartment upon actin filament disruption by either cytochalasin D or latrunculin. These contrasting effects may reflect the use of different model systems. The physiologic role played by actin on AQP2 trafficking in renal principal cells in situ is still not clear, but apical fluid shear stress has been reported also to depolymerize the apical actin cytoskeleton and cause AQP2 membrane accumulation in IMCD cells, suggesting that luminal flow might also be implicated in this response in vivo.
Identification of Actin-Associated Proteins Potentially Involved in Aquaporin-2 Trafficking
Many studies have implicated actin and associated proteins such as the myosins, as well as microtubules (see later), in sequential transport steps of vesicle trafficking. Myosin I, an actin-associated motor protein, was localized on AQP2-containing vesicles by electron microscopy, and various other myosin isoforms, including nonmuscle myosins IIA and IIB, myosin VI, and myosin IXB, were identified by mass spectrometry using these vesicles. Coupling of motor proteins to vesicles requires multiple Rab proteins, and myosin VB has been suggested to play a role in the AQP2 shuttle by Rab11-family interacting protein 2–dependent recycling through a perinuclear Rab11 compartment. Quantitative proteomics has revealed a network of 14 actin regulatory proteins whose abundance is changed in cortical CD cells after AVP stimulation. Myosin light-chain kinase, the myosin regulatory light chain, and nonmuscle myosin IIA and IIB isoforms have also been detected in rat IMCD cells and implicated in a calcium/calmodulin-regulated pathway leading to AQP2 membrane accumulation. These results support previous data that Ca 2+ release from ryanodine-sensitive stores plays an essential role in AVP-mediated AQP2 trafficking via a calmodulin-dependent mechanism. A role of Epac, which is expressed in the CD, in AVP-induced calcium mobilization, and AQP2 exocytosis in perfused CDs, has also been shown. Epac has also been implicated in extending the AQP2 transcriptional response to AVP when cAMP levels are no longer elevated. However, the role of calcium in the AVP response was questioned by another group who provided capacitance data in support of a cAMP-dependent but calcium-independent exocytotic process after AVP stimulation.
Immunoaffinity isolation of AQP2 followed by protein mass spectrometry has been used to identify a “multiprotein complex” containing ionized calcium–binding adapter molecule 2, myosin regulatory light-chain smooth muscle isoforms 2A and 2B, α-tropomyosin 5b, annexin A2 and A6, scinderin, gelsolin, α-actinin-4, αII-spectrin, and myosin heavy-chain nonmuscle type A. Interestingly, the gelsolin-like protein adseverin is much more highly expressed in CD principal cells than gelsolin (which is abundant in intercalated cells), indicating that it might be a physiologically important player in calcium-activated actin remodeling. In addition to myosins, members of the ERM (ezrin-radixin-moesin) family of scaffolding proteins, have also been implicated in the apical trafficking process. Ezrin expression is significantly upregulated in the CDs of lithium-treated rats, indicating that actin remodeling might occur. The GTPase Rap1 and the signal-induced proliferation–associated gene 1 (SPA-1) may also have a role in regulating AQP2 trafficking. Activation of Rap1 was found to inhibit AQP2 plasma membrane targeting, possibly by increasing actin polymerization mediated by SPA-1.
Based on these studies, it is clear that actin and its complex array of regulatory proteins play critical roles in the membrane accumulation and recycling of AQP2. However, with few exceptions, the precise steps in the pathway, and how these processes are regulated by AVP (e.g., via protein phosphorylation) have not been established in any detail.
Microtubules and Aquaporin-2 Trafficking
Intracellular vesicles can move along microtubules, driven by microtubule “mechanoenzymes” or motors. It is therefore not surprising that microtubule-depolymerizing agents such as colchicine and nocodazole partially inhibit the AVP-induced water permeability increase in target epithelia. Colchicine and combretastatin treatment disrupt the apical localization of AQP2 in rat kidney principal cells, resulting in AQP2 scattering in vesicles throughout the cytoplasm. Furthermore, cold treatment, which depolymerizes microtubules, also inhibits the AVP response, indicating that caution must be exercised in the interpretation of data from cell or tissue preparations that involve a cold incubation step as part of the experimental procedure.
Two large protein families are critically involved in microtubule-based vesicle movement. These are ATPases known as motor proteins, the dyneins and the kinesins. Minus end–directed motors (dyneins) transport vesicles toward the microtubule-organizing center, whereas plus end–directed motors (kinesins) transport vesicles in the opposite direction. Dynein and dynactin, a protein complex thought to link dynein to microtubules and vesicles, are associated with AQP2-bearing vesicles. The effect of AVP on transepithelial water flow in toad bladder and CDs was only partially inhibited by microtubule disruption (approximately 65% in CDs). These data support the idea that microtubules are involved in the long-range trafficking of vesicles toward the plasma membrane, but that the final steps of approach and fusion are microtubule independent. Upon AVP exposure the microtubule network is reversibly reorganized with increased formation of microtubules in the cell periphery. In the same study, depolymerization of microtubules prevented the perinuclear positioning of AQP2 in resting cells, but, after internalization of AQP2 following AVP washout, forskolin stimulation still caused a redistribution of AQP2 to the plasma membrane, in agreement with previous data. These results suggest that the microtubule-dependent translocation of AQP2 is predominantly responsible for trafficking and localization of AQP2 inside the cell after internalization, but not for its exocytic translocation. Microtubules are also critical for the trafficking of the V 2 R to lysosomes after ligand-induced internalization, as described earlier (see Figure 11.2 ).
Later studies demonstrated a role of microtubules in the basolateral to apical transcytosis of AQP2. Data from MDCK cells suggest that AQP2 trafficking to the apical recycling endosome and ultimately the apical plasma membrane can occur via an indirect pathway through the basolateral membrane of epithelial cells ( Figure 11.10 ). It is possible that this pathway is in some way disrupted or absent in LLC-PK 1 cells, in which AQP2 is inserted into the basolateral membrane both constitutively and after stimulation by AVP or forskolin.

SNARE Proteins and Aquaporin-2 Trafficking
The membrane docking and fusion steps of AQP2 vesicle exocytosis are mediated by vesicle-targeting proteins similar to those described in many other cell types. Vesicle tethering, docking, and fusion involve a complex series of protein-protein interactions that are combined under the name “the SNARE hypothesis.” This requires a complex interaction between integral membrane proteins, the SNAREs (soluble N -ethylmaleimide–sensitive factor attachment protein receptors), present in the vesicle (v-SNAREs) and the target membrane (t-SNAREs). In the CD principal cell, several proteins of the SNARE complex are associated with AQP2-containing vesicles and/or the apical plasma membrane of principal cells. The SNARE protein VAMP-2 (vesicle-associated membrane protein 2, also known as synaptobrevin-2) is associated with AQP2 vesicles, and disruption of VAMP-2 with tetanus toxin diminishes cAMP-dependent AQP2 trafficking. Further SNARES that co-localize with AQP2 include VAMP-3 (cellubrevin), SNAP23 (synaptosomal-associated protein 23), and the ATPase Hrs-2, which may regulate exocytosis via interaction with SNAP25. The t-SNARE syntaxin 4 is present in the apical plasma membrane of CD principal cells, and SNAP23 is associated with syntaxin 4 and VAMP-2. The interaction of AQP2 and the t-SNARE complex may be mediated by the protein snapin and/or by the angiotensin-converting enzyme 2 homolog collectrin, which has been implicated in salt-sensitive hypertension. Furthermore, other proteins that are involved in exocytotic processes in other cell types, such as members of the Rab GTPase family Rab3 and Rab5a, have been identified in AQP2-containing vesicles and may also play a role in vesicle docking and fusion. Several additional SNARE proteins, including syntaxin 7, syntaxin 12, and syntaxin 13, were identified in a proteomic screen using vesicles immunoisolated using anti-AQP2 antibodies. However, such isolated vesicles represent a mixed population that contain AQP2 but are not all in the exocytotic pathway. A study using small interfering RNA has shown that VAMP-2, VAMP-3, syntaxin 3, and SNAP23 are a complementary set of SNAREs responsible for AQP2-vesicle fusion into the apical plasma membrane, and Munc18b is a negative regulator of the SNARE complex. Finally, VAMP-8–null mice have hydronephrosis, and AQP2 exocytosis is impaired in VAMP-8–null CD cells. This study also showed that VAMP-8 interacts with both syntaxin 3 and syntaxin 4.
Long-Term Regulation of Water Balance
The actions of AVP on the kidney CD are mediated by two different but convergent mechanisms. As described earlier, AVP-mediated short-term regulation of CD water permeability is highly dependent on AQP2 trafficking events. Of equal importance are the effects of long-term AVP exposure. Prolonged dehydration increases urinary concentrating ability to a similar extent as acute AVP treatment, whereas chronic water loading reduces urinary concentrating capacity. The consequences of long-term dehydration are complex and probably result from numerous adaptational changes. For example, in addition to changes in circulating AVP levels, dehydration alters circulating levels of glucocorticoids and prostaglandins, in addition to causing adaptational changes in the kidney, such as reducing glomerular filtration rate. However, one aspect of long-term regulation can be ascribed to changes in CD water permeability. Lankford and colleagues demonstrated that isolated perfused CDs isolated from water-restricted rats displayed a much higher AVP-stimulated osmotic water permeability than tubules from water-loaded rats, suggesting an adaptation in CD principal cells. One such adaptation is that AQP2 expression markedly increases in response to water restriction with a greater abundance of AQP2 in the apical plasma membrane, and long-term AVP exposure results in increased abundance of several forms of phosphorylated AQP2. It was shown that the cAMP responsive element pathway is involved in the initial rise in AQP2 levels after DDAVP stimulation, but not in the long-term effect of DDAVP. Instead, long-term regulation of AQP2 may involve the activation of Epac. In addition, both AVP-dependent and AVP-independent pathways probably play a role in these changes, with evidence suggesting that both secretin and oxytocin are important for AVP-independent AQP2 translocation and expression. Also, the long-term effects of AVP on medullary hyperosmolality should not be underestimated, because extracellular tonicity, through activation of the tonicity-responsive enhancer–binding protein, increases AVP-induced AQP2 transcription and subsequent whole-cell AQP2 abundance.
Acquired Water Balance Disorders
In addition to the rare hereditary forms of NDI, resulting in the inability of the kidney to respond to AVP and produce a concentrated urine, acquired forms of NDI occur much more frequently and arise as a consequence of drug treatments, electrolyte disturbances, and urinary tract obstruction ( Table 11.1 ). These conditions are discussed extensively in other chapters, and only an overview is provided here.
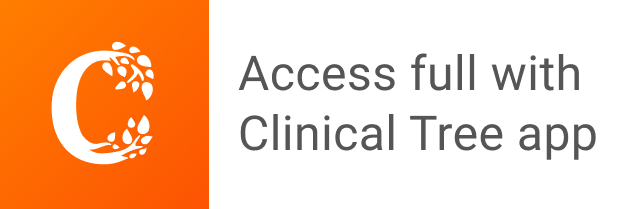