As kidney disease progresses, renal regulation of acid balance is lost and metabolic acidosis ensues, due primarily to impaired acid excretion. Initiation of renal replacement therapy addresses this problem not by removing H+ but by adding HCO3− to the body on a regular basis. Net addition of HCO3− during dialysis therapy is regulated not by any physiologic feedback mechanism but by the physical principles of diffusion and convection (5–7). This switch has major implications for acid–base homeostasis, changing the way one thinks about both normal and disordered acid balance.
Throughout this chapter, the term [HCO3−] is used both for serum and bath concentrations. In most instances, however, serum [HCO3−] is actually measured as serum [total CO2]. Keep in mind that serum [total CO2] includes dissolved CO2 as well as HCO3− and is on average about 1 mmol/L higher than serum [HCO3−].
GENERAL PRINCIPLES
Regardless of the alkali source added to the dialysis bath or infused during renal replacement therapy, a new equilibrium is achieved that is driven primarily by variations in endogenous acid production, just as in individuals with normal kidney function (5–7). The fundamental reason for this linkage is that the net gain of HCO3− is directly related to its transmembrane concentration gradient. Therefore, the lower the concentration of HCO3− in the body fluids at the onset of treatment, the greater will be the net entry of alkali. In patients receiving renal replacement therapy, the prevailing serum [HCO3−] is determined jointly by the dialysis treatment itself and by endogenous acid production. Because the dialysis prescription is fixed, the variable component is endogenous acid production, which likely accounts for most of the variability in serum [HCO3−] in patients with end-stage kidney disease (ESKD) (5–7). Although less flexible than functioning kidneys, this property of dialysis therapy allows for variations in endogenous acid production to be matched by variable amounts of net HCO3− addition during treatment. The details of how this equilibrium is achieved are discussed first for peritoneal dialysis (PD) and then for hemodialysis (HD).
ACID–BASE HOMEOSTASIS IN PERITONEAL DIALYSIS
In recent years, there has been a resurgence of PD as a treatment for ESKD. The number of incident PD patients increased from 7,588 in 2000 to 9,451 in 2012 (8). Monthly prevalent counts increased by 24% between January 2010 and October 2012, compared to only 9.6% increase in HD (9). This resurgence is likely due to the finding that PD is as beneficial as HD but less expensive and also to new financial incentives for initiation of PD (9).
Automated peritoneal dialysis (APD) is now more commonly used than continuous ambulatory peritoneal dialysis (CAPD) as a result of improvement in technology and better perceived quality of life (10); patients have more freedom during the day as the therapy is delivered primarily at night with the help of a machine that is easy to use. With this resurgence, attention has been directed at trying to identify the best solution to restore body alkali stores and at the same time minimize damage to the peritoneal membrane. In general, it is recommended that plasma bicarbonate be maintained in the high normal range: Protein catabolism is reduced at bicarbonate levels around 30 mmol/L and nutritional status seems to be improved (11). On the other hand, there is a concern that higher bicarbonate levels may be associated with soft tissue calcifications and adynamic bone disease, but these complications have not been shown to occur in PD so far (11).
Alkali Source in the Peritoneal Dialysis Solutions
As in the case of HD, the first PD solutions contained bicarbonate (12). At that time, commercially prepared dialysis solutions were not available for clinical use, and fresh solutions were prepared in the hospital at the time PD was performed. In order to avoid precipitation of calcium carbonate if bicarbonate solutions were to be stored for longer periods of time, calcium was added shortly before inflow into the abdomen (12). As commercial solutions were developed in the 1950s, another problem became apparent. The high pH in bicarbonate-based PD solutions facilitated caramelization of glucose during the sterilization process and generation of glucose degradation products that were thought to contribute to peritoneal membrane damage after prolonged exposure. In short order, bicarbonate was removed from most PD solutions and replaced with acetate or lactate, organic anions that are absorbed and metabolized to bicarbonate in the body. Acetate-containing solutions were abandoned quickly because of the untoward effects on the peritoneal membrane from local vasodilation. These effects included increased membrane permeability with loss of ultrafiltration, and encapsulating peritoneal sclerosis (13). Lactate quickly became the standard alkali source because it is rapidly metabolized to HCO3− while serum lactate concentration remains low. Initially, solutions contained sodium lactate at a concentration 35 mmol/L, but almost all standard solutions now contain at a concentration of 40 mmol/L because serum [HCO3−] is higher using the latter concentration (14–16) (TABLE 25.1). Lactate-based solutions have been well tolerated, but concerns remain about damage to the peritoneal membrane after long-term exposure to the low pH of the solution when first introduced into the abdomen. Within 15 minutes, of course, pH rises in the peritoneal space due to rapid entry of HCO3− across the peritoneal membrane (FIGURE 25.2) (13).
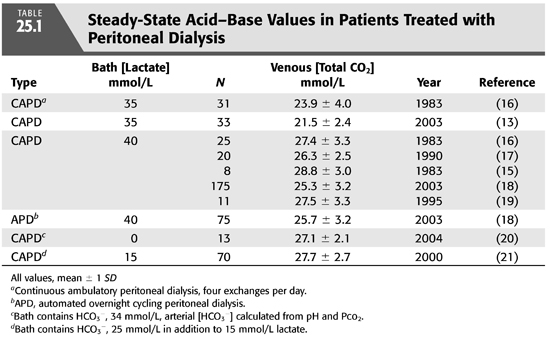
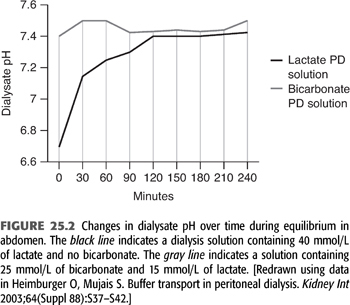
The search for biocompatible PD solutions has brought bicarbonate-based solutions back into the forefront (13,20–22). Packaging the PD solution in a two-compartment bag system allows for separation of HCO3− from glucose, calcium, and magnesium salts. The two bags are mixed just prior to infusion into the abdomen, and rapid equilibration of the mixed solution with body PCO2 keeps the pH in a range at which calcium and magnesium salts do not precipitate. This solution can be used in both APD and CAPD. Another advantage of the bicarbonate-based solutions is the lower incidence of in-flow pain due to the physiologic pH of the solution as compared to low initial pH in lactate-based solutions (22).
Buffer Transport in Peritoneal Dialysis Using a Lactate-Containing Solution
During PD, the peritoneal cavity is filled with fresh dialysis solution, which is allowed to dwell for a variable amount of time. The standard lactate-based PD fluid composition is shown in TABLE 25.2. During the dwell, HCO3− and lactate cross the peritoneal membrane down their respective concentration gradients causing a gradual decline in dialysate [lactate] and increase in dialysate [HCO3−]. The rate of HCO3− movement into the bath diminishes as the concentration rises in the dialysate, but even after a 6-hour dwell, equilibrium is not achieved (FIGURE 25.3) (19). At the end of the dwell, the spent dialysate is drained, and fresh PD fluid is infused into the peritoneal cavity. PD is in most instances a continuous procedure; PD fluid is present in the peritoneal space 24 hours a day, 7 days a week. As a result, serum [HCO3−] is stable and does not fluctuate as it does with intermittent HD (see later).
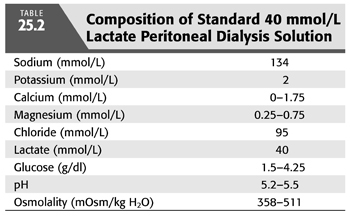
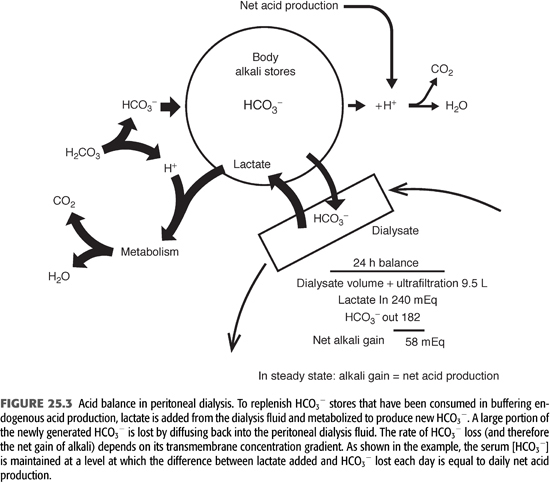
Because blood lactate concentration is approximately 1 mmol/L, lactate diffuses from the peritoneal cavity into the systemic circulation across the peritoneal membrane. The absorbed lactate is metabolized primarily in the liver to generate an equivalent amount of new HCO3−. As a result of rapid metabolism, serum [lactate] does not increase notably during PD, allowing for a favorable concentration gradient to be maintained during the dwell. When the PD fluid is left in the peritoneal cavity for 6 hours, approximately 75% of the lactate is absorbed and metabolized, yielding an equivalent amount of new bicarbonate (19). A portion of the newly generated HCO3− is consumed in buffering endogenous acids produced by body metabolism on a daily basis. Because the PD solution contains no HCO3−, a large portion of the newly generated HCO3− also diffuses from the patient into the peritoneal cavity down its concentration gradient (5,7,23). Its rate of diffusion is determined by the same principles that govern lactate transport, and its concentration in the peritoneal cavity increases during the dwell. After 6 hours, PD fluid [HCO3−] is about 80% of the serum level (Figure 23.3) (19). The net alkali gained for any given period is equal to the difference between the systemically added lactate and the loss of HCO3− into the PD fluid. (5,7,19).
Based on the relative diffusion rates of HCO3− and lactate, one can estimate the net alkali gain for a standard CAPD prescription (23). In the model shown in Figure 23.3, serum [HCO3−] is 24 mmol/L and the patient is receiving four 2-L exchanges, each with a dialysate lactate concentration of 40 mmol/L each day. For this prescription, total lactate content in the PD fluid each day will be 8 × 40 = 320 mmol/d, and total lactate added to the body fluids is 320 × 0.75 = 240 mmol/d. If dialysate [HCO3−] is 80% of the serum concentration at the end of the dwell period, dialysate [HCO3−] will be 24 × 0.8 = 19 mmol/L. Assuming the additional removal of 1.5 L of fluid during a 24-hour period of dialysis, the total spent dialysate volume will be 8 + 1.5 = 9.5 L. Total HCO3− content in the spent dialysate will be 9.5 × 19 = 182 mmol/d. Total systemic alkali gain is therefore 240 − 182 = 58 mmol/d. If this net alkali gain exceeds the rate of endogenous acid production, serum [HCO3−] will rise, increasing the amount of HCO3− lost, until net alkali gain matches endogenous acid production. At this point, an equilibrium will be achieved in which net alkali gained will equal net acid production. These theoretical considerations are supported by direct measurements, showing that patients treated with PD are in acid balance, with serum [HCO3−] related inversely to endogenous acid production (19).
Bicarbonate-Based Solutions
Bicarbonate-based PD solutions are now commercially available in Europe and Canada, using a two-bag system to prevent precipitation of calcium and magnesium salts during storage. When this system is used, acid–base status is improved in patients with low serum [HCO3−] when bath [HCO3−] is increased from 34 to 39 mmol/L (14,20). Newer solutions are now available containing a mixture of bicarbonate and lactate (B/L) (11,13,21). The B/L solutions are also packed in a two-bag system. The B/L dialysis solutions made by Baxter contain [HCO3−] at 25 mmol/L and [lactate] at either 10 or 15 mmol/L. Unfortunately, neither bicarbonate nor B/L solutions are available for use in the United States.
The proposed advantage of the B/L solutions is a physiologic pH and PCO2, thereby reducing glucose-degradation products (11). Clinical experience with B/L solutions showed efficient acid–base control and reduction in infusion pain due to physiologic pH of the solution (pH of 7.4) compared to 5.5 in lactate-only solutions (10,13,21,22). A modest but significant increase in ultrafiltration was observed in patients using a B/L solution compared to lactate (21).
Effect of Automated Peritoneal Dialysis on Serum [HCO3−]
As APD is the predominant form of PD, it is important to consider its effect on the patient’s acid–base status. In APD, patients are exposed to higher total volume of dialysis solutions, but most of the dwells are shorter in duration. Current experience shows that good control of acid–base balance can be obtained regardless of whether CAPD or APD is used (FIGURE 25.4). As seen in the figure, most patients achieve a plasma bicarbonate concentration of 22 to 30 mmol/L, irrespective of modality status, and there is no notable difference in average serum [HCO3−] (10,18).
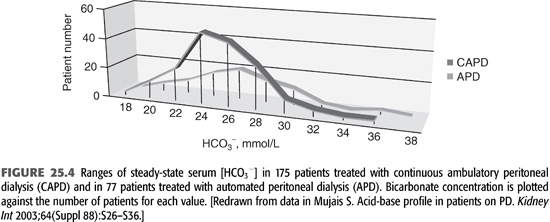
Lactate and bicarbonate are transported faster across the peritoneal membrane in high transporters compared to low transporters. For this reason in CAPD, serum bicarbonate concentration might be slightly higher in high transporters compared to low transporters, but the difference has no physiologic consequence (18). In APD, there is no difference in serum [HCO3−] among different type of transporters due to higher volume of dialysate used (10).
ACID–BASE HOMEOSTASIS IN HEMODIALYSIS
Conventional HD is an intermittent treatment, in contrast to the continuous nature of PD. As a result, there is no day-to-day steady state with regard to alkali stores and serum [HCO3−]. As shown in FIGURE 25.5, alkali is added rapidly during a 3- to 4-hour treatment three times a week. This added alkali then gradually dissipates during the period between treatments, as HCO3− is consumed by endogenous acid production and diluted by fluid retention (7). Thus, from an acid–base perspective, HD patients undergo a continuous back-and-forth fluctuation from a postdialysis serum [HCO3−] in the high normal or slightly alkaline range to a predialysis serum [HCO3−] in a range that is usually slightly below normal. Given that serum [HCO3−] falls by as much as 7 to 8 mmol/L from the end of one dialysis to the beginning of the next, it is important to note when acid–base status is assessed. By convention, one usually reports the predialysis value and, ideally, the nadir value obtained after the longest interval between treatments (TABLE 25.3).
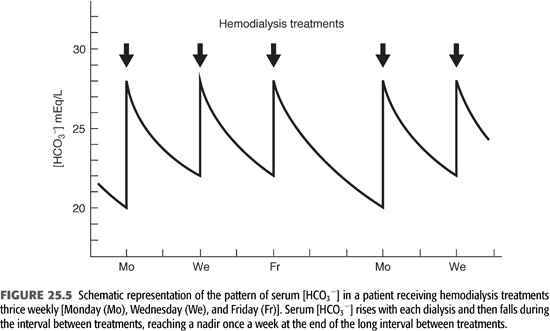
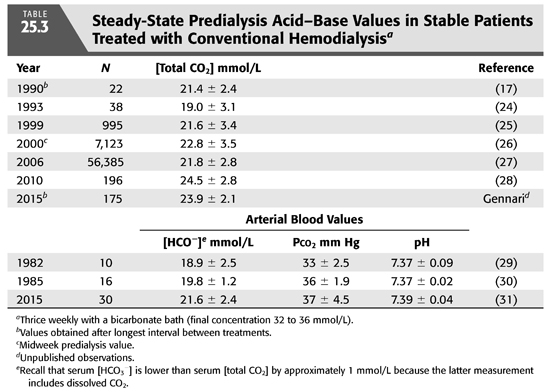
Alkali Source
When HD was first developed, the bath solution contained HCO3− in a concentration of 26 mmol/L (32), but the concentration was empirically increased to 35 mmol/L over the next decade to improve alkali transfer (33). To prevent precipitation of CaCO3, bath pH had to be adjusted to 7.4 by aeration with a carbon dioxide/oxygen gas mixture. Acetate was first introduced as an alkali precursor in 1964 (34). This organic anion was chosen because it was metabolized readily by most tissues in the body (35), allowing for rapid HCO3− production during treatment. Because acetate obviated the need for aerating the bath with CO2, it allowed for a central bath solution preparation system that could be used for multiple patients, and it quickly replaced HCO3− as an alkali source.
This change created a bidirectional dynamic similar to that for PD with lactate, although much more rapid and impressive in magnitude. Almost as quickly as the acetate added from the bath to the patient was metabolized to HCO3− during HD, the new HCO3− diffuses back into the bath (6). As a result, the only way to add substantial amounts of HCO3− to the body stores was to add sufficient acetate to overcome its rate of metabolic conversion and raise its concentration in the blood by the end of the treatment. After the dialysis session, this unmetabolized acetate (as high as 3 to 4 mmol/L) is converted to HCO3− and the new HCO3− is now retained by the patient. By trial and error, a bath acetate concentration of 37 mmol/L was settled on to achieve this goal. This concentration represented a compromise between providing sufficient substrate for HCO3− generation during each treatment and limiting the rate of acetate delivery to an amount that could be metabolized without producing toxic levels (36–38).
Despite the ease of bath preparation, alkali repletion using acetate is a remarkably inefficient process. During a 4-hour HD treatment, for example, more than 700 mmol of HCO3− is lost into the bath (6,37). This loss limits alkali replenishment, so that patients receiving this form of dialysis have predialysis serum [HCO3−] values of less than 18 mmol/L, on average (6,17,37,39). As blood-flow rates and dialyzer membrane permeability increased, the amount of acetate delivered also increased and serum acetate concentration often reached toxic levels, causing hypotension and other symptoms (30,36,38,40–42). An additional problem with using acetate-based dialysis solutions was CO2 loss from the patient to the bath, an effect that decreased ventilatory drive and contributed to dialysis-induced hypoxemia (42,43).
Because of these problems, HCO3− was again introduced as the source for alkali replenishment in the early 1980s (29,43,44). Reintroduction of HCO3− into the bath solution was made possible by a new technology, the use of a proportioning system that allowed continuous production of dialysate from concentrated salt solutions either in a central delivery system or individually for each patient. In such a system, NaHCO3 is added to the rest of the bath solution just before delivery to the dialysis membrane. At the moment of HCO3− addition, the pH of the bath is prevented from rising too high by the presence of a small amount of acetic acid. The acetic acid reacts rapidly with the added HCO3−, yielding carbonic acid and acetate anions. The final bath solution after NaHCO3 addition has a pH of approximately 7.05 and contains HCO3− (most commonly at a concentration of 35 mmol/L), acetate (4 mmol/L in most systems), and dissolved CO2 (4 mmol/L, equivalent to a PCO2 of 133 mm Hg). The PCO2 in the bath falls rapidly as it diffuses across the dialysis membrane, raising the PCO2 in the postfilter blood to only approximately 50 mm Hg (37). The inward diffusion of CO2 reverses the loss from the patient that occurred with acetate dialysis (see earlier) but has no notable impact on systemic PCO2 or ventilation (43,44). The acetate produced by this reaction also diffuses across the dialysis membrane into the blood, providing a small additional source of new HCO3− as it is metabolized.
Reintroduction of HCO3− into the HD bath solution increased predialysis serum [HCO3−] by 3 to 4 mmol/L (29,30,42,45–47) and decreased patient symptoms dramatically (29,30,45,48). By 1990, this technology essentially completely replaced acetate HD. Bath solutions currently used for conventional HD most commonly combine a 39-mmol/L HCO3− solution with a solution containing 4 mmol/L of acetic acid, yielding a final bath [HCO3−] of 35 mmol/L and [acetate] of 4 mmol/L when mixed. One formulation uses sodium diacetate, which yields an [acetate] of 8 mmol/L. The acetate anions cross the dialysis membrane into the blood and are rapidly metabolized, producing 4 or 8 mmol/L of new HCO3−. This additional HCO3−, however, has no impact on end-dialysis [HCO3−] because, to the extent the new HCO3− is not consumed by buffering or organic acid production and is retained in the blood, it simply reduces the transmembrane HCO3− gradient and slows the rate of HCO3− entry from the bath. The end-dialysis [HCO3−] is determined by the bath [HCO3−] and by the magnitude of the increase in organic acid production during the treatment engendered by the abrupt alkalinization of the body fluids (see later). Because of continued concerns about the effects of acetate on body metabolism even at low concentrations, citric acid has replaced acetic acid in many dialysis units as the organic acid used to control bath pH before HCO3− addition. This trivalent acid is effective at lower concentrations, and the citrate anions produced are rapidly metabolized to generate bicarbonate. In a standard citrate-based dialysis bath solution, metabolism of citrate yields 2.7 mmol/L of HCO3−.
Acid–Base Balance
Although the transient events are more complex than in PD, a similar equilibrium is established between net acid production and alkali addition in patients receiving intermittent HD. The amount of HCO3− added from the bath to the patient during an HD session is primarily dependent on the dialysance of this anion (a function of dialysis membrane surface area and permeability) and the transmembrane concentration gradient (FIGURE 25.6). Because the bath [HCO3−] is fixed, as are the surface area and permeability of the membrane, the variable factor in this relationship is serum [HCO3−]. The serum [HCO3−] at the beginning of each treatment is determined primarily by the rate of acid production in the interdialytic period and to a lesser extent by fluid retention (see later). The lower the initial value, the greater the initial rate of HCO3− addition, and vice versa.
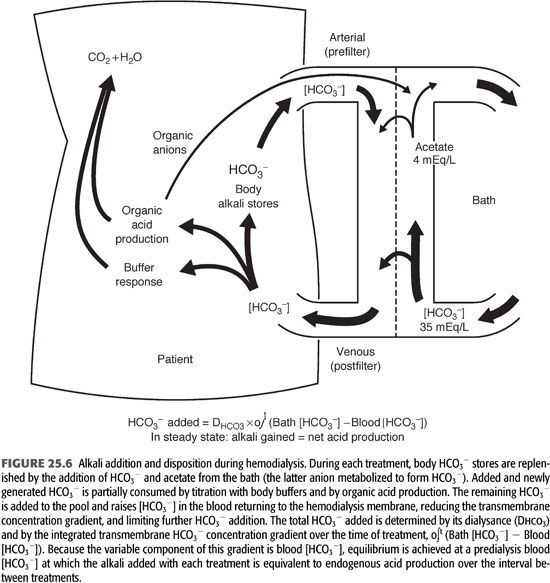
The total amount of HCO3− added during each HD treatment is determined not only by the initial transmembrane concentration gradient but also by the rate of change in the gradient as the treatment progresses. To the extent that newly added HCO3− is retained in the extracellular fluid (ECF) compartment, its concentration in the blood will rise, diminishing the gradient across the dialysis membrane. The minute-to-minute change in this gradient is determined by the extent to which the added HCO3− is consumed by the production of new H+ in the body (FIGURE 25.6). The response to rapid alkalinization of the body fluids includes not only the release of H+ from nonbicarbonate buffers but also the stimulation of cellular organic acid production (4,7,47,48).
Increases in the production of organic acids that dissociate at the pH of the body fluid can produce almost unlimited amounts of new H+ to titrate and remove added HCO3− from the extracellular compartment. If the organic anions produced by this titration were retained, they would eventually be metabolized and thereby regenerate the HCO3− titrated by their formation. Organic anions, however, readily cross the dialysis membrane and are lost into the dialysate (FIGURE 25.6) (46,47). At blood-flow rates much lower than are now customarily used, losses of 30 to 50 mmol of organic anions have been measured during a standard HD treatment (46,47). Because many more organic acids are produced than were measured in these studies, and because blood-flow and therefore clearance rates have increased, it seems likely that organic anion losses are now even higher (7). The magnitude of this response to added HCO3− remains undefined, but it is clear that organic acid production and loss of the organic anions formed can have a major influence on net alkali addition during HD.
The transmembrane HCO3− concentration gradient could also be influenced if the blood returning to the dialyzer contained an admixture of slowly mixing pools with differing HCO3− concentrations, but whether this occurs to any measurable degree is unknown. In addition, there may be ionic constraints on HCO3− diffusion, despite the presence of a favorable chemical concentration gradient, as it competes with Cl− and negatively charged proteins (Gibbs-Donnan effect) for charge balance with the cations in the blood, particularly during the latter half of the treatment. These factors probably influence net alkali addition to a much smaller extent than the buffer and organic acid responses, but their contribution remains undefined.
Assuming the factors controlling alkali addition during HD are constant from treatment to treatment, the amount of HCO3− added with each treatment is determined primarily by the predialysis serum [HCO3−]. This value, in turn, is determined by the rate of endogenous acid production in the period between treatments and to a lesser degree by the amount of fluid retained. Eventually, a new equilibrium is achieved in which the interplay between intradialytic and interdialytic events serves to maintain predialysis serum [HCO3−] relatively constant. One should emphasize, of course, that the predialysis value for serum [HCO3−] is the nadir in a descending trend, with the peak value occurring immediately after dialysis and falling gradually until the next session (FIGURE 25.5). Because of the complex nature of the response to alkali addition during HD and its variability from patient to patient, as well as differences in net acid production and fluid retention between treatments, predialysis serum [HCO3−] varies more widely in patients receiving intermittent HD than in individuals with normal kidney function (25,26).
Determinants of Postdialysis Serum [HCO3−]
FIGURE 25.7 shows the pattern and magnitude of the increase in serum [HCO3−] during a single HD treatment in seven stable patients. This figure shows the increase in serum [HCO3−] induced by 4 hours of HD (7). The striking feature shown in this figure is that serum [HCO3−] changes little, if at all, during the last 2 hours of dialysis, despite a 4- to 6-mmol/L concentration gradient (after Gibbs-Donnan adjustment) for HCO3− entry across the dialysis membrane. This pattern has been confirmed by other investigators (44,49). While the observations shown in FIGURE 25.7 are characteristic, serum [HCO3−] actually falls during dialysis in a small subset of patients (7). The fall in these patients most likely is due to a surge in lactic acid production, resulting from tissue hypoxia and hypotension.
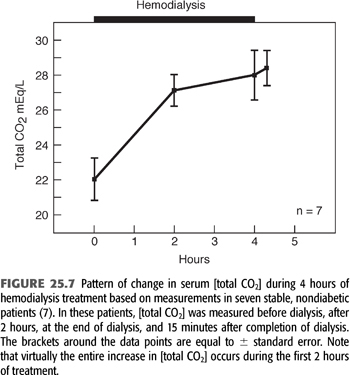
Although the factors responsible for determining end-dialysis serum [HCO3−] remain to be completely elucidated, this value is of critical importance because it sets the platform from which [HCO3−] slowly falls in the period between treatments. Assuming no intervening events, the postdialysis serum [HCO3−] is normally determined primarily by the specific dialysis [HCO3−] prescribed for any given patient.
Determinants of Predialysis Serum [HCO3−]
In the interval between HD sessions, the two major determinants of the rate of fall in serum [HCO3−] are the rate of endogenous acid production and the rate of fluid retention. Endogenous acid production between treatments is determined primarily by diet; patients ingesting a diet low in sulfur-containing proteins will have low rates of endogenous acid production and vice versa (1,2). Although there is no information on the effect of specific diets on predialysis serum [HCO3−], a clear inverse correlation between normalized protein catabolic rate (an indirect measure of dietary protein intake) and predialysis serum [HCO3−] is evident in patients receiving HD (25–27,50).
On the basis of these observations and a few assumptions, one can estimate the magnitude of the effect of variations in endogenous acid production on predialysis serum [HCO3−]. If one assumes that all the acid produced is retained and that the buffer space for the retained H+ is 50% of body weight [an assumption supported by measurements in HD patients (50)], such an analysis predicts that variations in acid production will alter predialysis serum [HCO3−] by as much as 6 mmol/L (TABLE 25.4). Given these assumptions, variations in daily acid production can mean the difference between a low normal and frankly acidotic predialysis serum [HCO3−] in patients receiving exactly the same dialysis prescription. Although the major determinant of endogenous acid production is dietary protein intake, the catabolic state of the patient will also influence acid production.
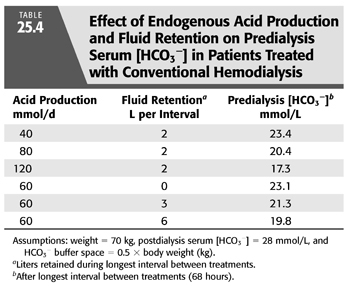
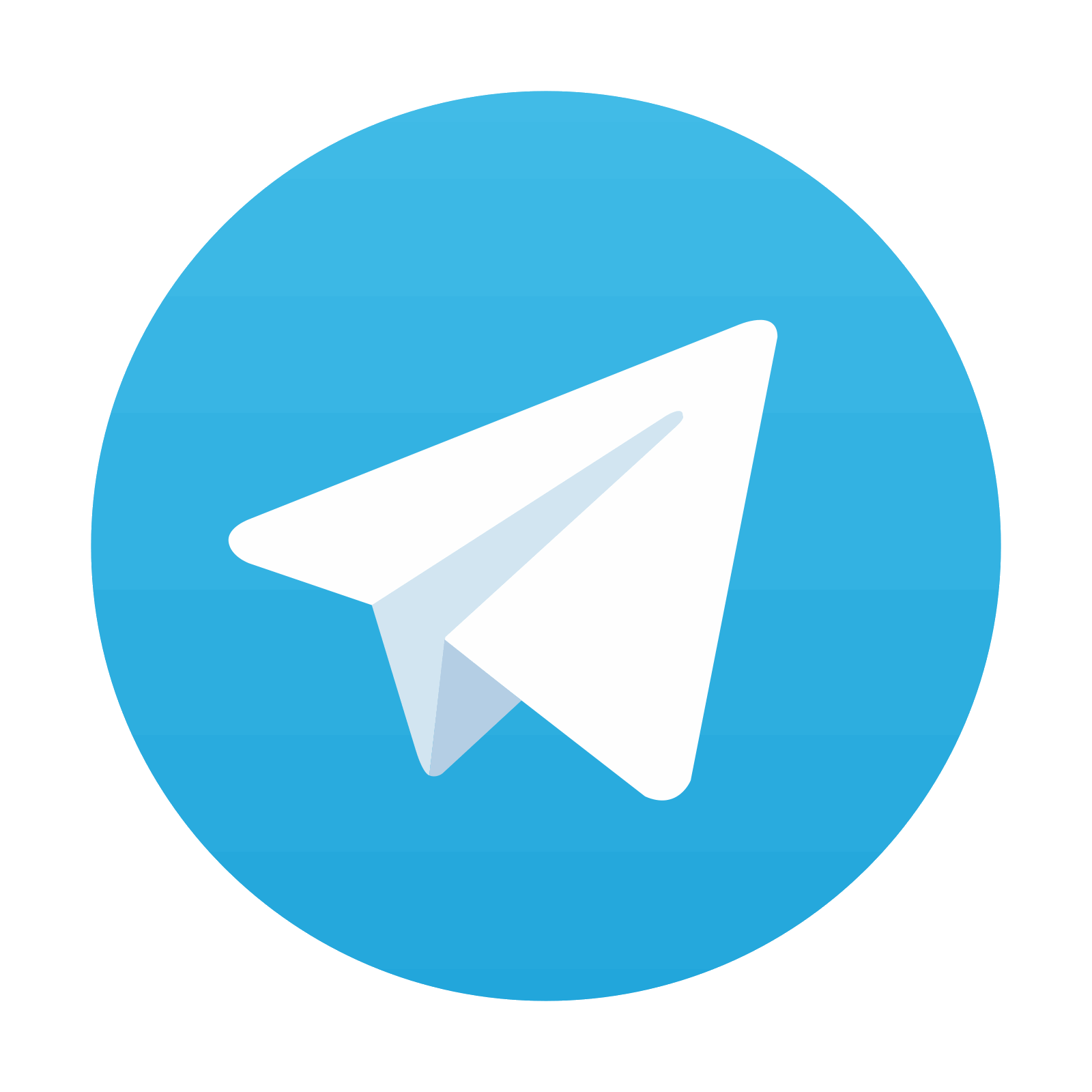
Stay updated, free articles. Join our Telegram channel
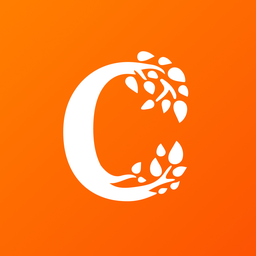
Full access? Get Clinical Tree
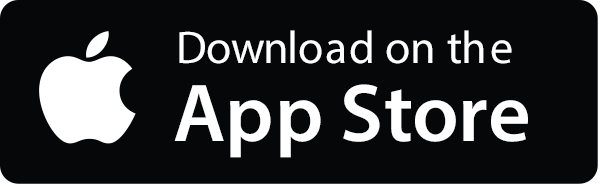
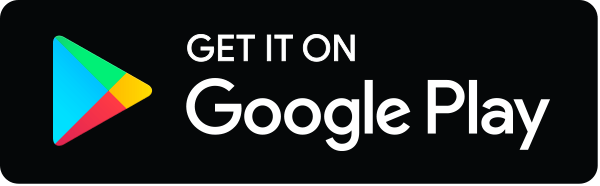