Wesley M. White, MD, Jihad H. Kaouk, MD The management of renal cell carcinoma has undergone a paradigm shift over the last two decades. The widespread use and refinement of modern imaging during this period has led to a dramatic increase in the number of incidentally discovered and low-stage renal lesions (Jayson and Sanders, 1998; Volpe et al, 2004). This fortunate trend has allowed urologists to explore alternative and less invasive forms of therapy, among them nephron-sparing surgery and renal ablative technologies (Colombo and Kaouk, 2007; Pinto, 2009). Cancer-specific survival, preservation of renal function, avoidance of treatment-related morbidity, and patient-centered quality-of-life outcomes now constitute the ultimate goals for management of early-stage renal cell carcinoma. The preponderance of evidence-based data champions the role of partial nephrectomy in the treatment of localized unilateral small renal masses (Herr, 1999; Fergany et al, 2000). Nephron-sparing surgery, in this setting (tumors < 4 cm), offers comparable oncologic outcomes (>95% cancer-specific survival) as compared with radical nephrectomy and is associated with improved preservation of renal function, superior cardiac outcomes, and improved overall survival (Thompson et al, 2008; Huang et al, 2009; Zini et al, 2009). Moreover, nephron-sparing surgery avoids overtreatment of indolent or benign tumors, a particularly germane concern, because nearly 20% of malignant-appearing, small renal masses are pathologically benign (Frank et al, 2003). For these reasons, open partial nephrectomy is currently considered the gold standard treatment for radiographically enhancing small renal masses (Novick, 2004). Despite its aforementioned long-term advantages, open partial nephrectomy is nevertheless associated with significant short-term morbidity, namely substantial postoperative pain, prolonged hospitalization, and a protracted convalescence (Gill et al, 2007). Because a large proportion of patients who are candidates for partial nephrectomy demonstrate concomitant co-morbidities or are of advanced age, this short-term morbidity must be taken into consideration during patient counseling, and less invasive alternatives, if appropriate, should be discussed. Laparoscopic partial nephrectomy (LPN) replicates the principles of open nephron-sparing surgery but with less attendant surgical morbidity (Gill et al, 2002). Indeed, laparoscopic partial nephrectomy offers markedly improved convalescence and equivalent disease-free survival as compared with open partial nephrectomy (Aron and Gill, 2007; Andonian et al, 2008; Marszalek et al, 2009). However, laparoscopic partial nephrectomy remains a technically challenging procedure with significantly higher complication rates, even among experienced surgeons at centers of excellence (Gill et al, 2007). Further, renal hilar occlusion is nearly universally required during LPN to minimize blood loss and facilitate tumor resection in a bloodless field. In expert hands, warm ischemia times during LPN approach 30 minutes (Kural et al, 2009; Thomas et al, 2009). Although controversial and dependent on the inherent renal function status, warm ischemia times that approach or exceed 30 minutes may place the kidney at considerable risk for postischemic injury (Thompson et al, 2007; Lane et al, 2008). Irrespective of the surgical approach chosen, nephron-sparing surgery is underutilized in the United States owing to the comparative risks and attendant technical demands associated with the procedure (Abouassaly et al, 2009). Recent Surveillance, Epidemiology, and End Results (SEER) data found that partial nephrectomy is performed in only 11.1% of patients, with the remaining 88.9% undergoing radical nephrectomy (Miller et al, 2008). Furthermore, when adjusted for patient demographics and tumor size, surgeon preference was significantly more important than patient characteristics with regard to the type of operation selected. Energy-based, in-situ tumor ablation was developed in an attempt to broaden the minimally invasive treatment options available to patients with ostensibly localized, low-stage renal tumors (Goel and Kaouk, 2008a). Focal ablative therapy confers myriad advantages compared with extirpative surgery. Principally, renal tumor ablation is associated with fewer complications, less morbidity, and a shortened convalescence (Desai et al, 2005). Second, prospective studies have demonstrated no significant impact on renal function following treatment (Shingleton and Sewell, 2003; Raman et al, 2008). Although a modicum of parenchymal loss does occur, renal hilar occlusion is not performed during tumor ablation, and postischemic injury is therefore avoided. Next, ablation offers considerable treatment flexibility. That is, tumors may be treated open, laparoscopically, or percutaneously with the approach tailored to the specifics of the patient and tumor (Rukstalis et al, 2001; Deane and Clayman, 2006). Finally, renal tumor ablation is technically less demanding to perform than either open or laparoscopic partial nephrectomy. Complex reconstruction is not required following treatment, and dissection and isolation of the renal hilum is not requisite. Given these advantages, ideal candidates for ablative treatment have traditionally included patients with small multifocal tumors, those with one or more tumors in a solitary kidney in which surgical warm ischemia would place the kidney at risk, and those who desired treatment but were not appropriate candidates for extirpative surgery (Lehman and Landman, 2008). Although well tolerated and technically less challenging than alternative extirpative options, concern exists regarding the oncologic efficacy of ablative techniques. A recent meta-analysis demonstrated a higher relative risk of local recurrence with cryoablation (RR = 7.45) and radiofrequency ablation (RR = 18.23) as compared with partial nephrectomy, but with no significant difference in progression to metastatic disease between the three groups (Kunkle et al, 2008). However, nonuniform criteria were used to define tumor recurrence in these series and pathology was not obtained for all specimens. Key Points: Management of the Small Renal Mass The concept of applying cold temperatures to destroy tissue is credited to an English physician, Dr. James Arnott (1797 to 1883). Using a combination of ice and salt, he was able to topically palliate tumors of the cervix and breast with variable success. Over the course of the ensuing decades, investigators developed more sophisticated, reproducible, and pragmatic methods of handling and applying topical cryogens (Gage, 1998). In 1963, a neurosurgeon from New York, Dr. Irving S. Cooper, introduced a probe capable of achieving a controlled temperature of −196° C in deep-seated tissues (Cooper, 1963). Using pressurized liquid nitrogen, this self-contained probe was employed to ablate otherwise inoperable brain tumors, as well as portions of the thalamus in patients with Parkinson disease. Cooper’s innovative concept ushered in a new era in cryosurgery in which larger volumes of tissue in less accessible locations could be successfully and reproducibly ablated. Although Cooper’s experience provided the proof in principle for cryoablation, its practical application for abdominopelvic tumors was not immediately realized. Early cryogen delivery systems could not unfailingly control the extent of tissue death owing to a generalized lack of cryoprobe sophistication and an unreliable system of monitoring the freezing process (Weber and Lee, 2005). As such, irreparable collateral damage was often a consequence of treatment, and the technique was transiently abandoned. It was not until the development of cryoablation under real-time image guidance with intraoperative ultrasonography that the procedure became a safe and pragmatic treatment choice. The application of intraoperative ultrasonography during cryosurgery addressed many of its aforementioned short-comings (Onik et al, 1984, 1985). First, ultrasonography offered an excellent method for evaluating the patient’s anatomy. The relative size and location of the area to be treated could be visualized and documented, and the entirety of the organ could be evaluated for occult metastatic disease. Second, precise probe placement could be assured by following its echogenic tip under ultrasound guidance. Lastly and most importantly, the freezing process could finally be monitored in real time by observing the formation and propagation of the highly echogenic leading edge of the iceball (Fig. 56-1). Because animal studies had already confirmed a close correlation between the sonographically visible iceball and the zone of cell death, surgeons now had a reliable and reproducible method of targeting and destroying tumors without attendant collateral damage (Steed et al, 1997; Campbell et al, 1998; Weber et al, 1998). Following treatment under image guidance, the next significant breakthrough with cryoablation was the introduction of argon gas–driven treatment systems (Rewcastle et al, 1999). Through the mid- to late 1990s, cryoablation was performed exclusively with liquid nitrogen-based systems that were relatively inefficient. Newer argon gas systems were developed, ones that use the Joule-Thomson principle (low temperatures are achieved by the rapid expansion of high-pressure, inert gas) to generate temperatures of −185.7° C within the treatment tissues. These systems require shorter treatment times and allow for smaller, more proficient cryoprobes that can be employed laparoscopically with fused ultrasound guidance or percutaneously under computed tomography (CT), magnetic resonance imaging (MRI), or ultrasound guidance. The majority of commercially available cryoablation units (CryoHit, Galil Medical, Plymouth Meeting, PA; CryoCare, CryoCare CS, Endocare, Irvine, CA; SeedNet, Oncura, Philadelphia, PA) now employ argon gas–based systems as described. The mechanism of tissue injury with cryoablation is multifaceted, reflecting direct cellular damage during the freezing phase and indirect reperfusion injury during the thawing phase (Mazur, 1977; Ishiguro and Rubinsky, 1994; Hoffman and Bischof, 2002). During rapid freezing or freezing at extremely low temperatures, ice crystals form within the intracellular spaces, causing cell death by direct injury to either the cellular membrane or structures within the cell. With gradual freezing, extracellular ice formation creates an osmotic gradient that results in intracellular fluid moving into the extracellular space. The result of this fluid shift is dehydration, cell membrane rupture, and subsequent cell death. During the thawing phase, reperfusion injury occurs that results in microcirculatory failure and small-vessel thrombosis (Weber et al, 1997; Kahlenberg et al, 1998). Although speculative, this may represent the dominant mechanism of cell kill during cryosurgery. The summative pathologic consequence of treatment is coagulative necrosis and fibrous scar formation. Key Points: Cryoablation Cushing and Bovie (1928) introduced the Bovie knife, an instrument that employed pulsed or continuous monopolar radiofrequency current to cauterize or cut tissue, respectively. Although this forerunner to modern electrocautery revolutionized and defined contemporary surgical techniques, it also provided the foundation for present-day radiofrequency ablation (RFA). Radiofrequency energy effects cell kill by transferring alternating radiofrequency current (450 to 1200 kHz) through insulated interstitial needle electrodes (Raj et al, 2003). This energy transfer generates ionic friction and agitation within tissue that results in heating (Organ, 1976-1977; LeVeen, 1997; Rehman et al, 2004). When tissue is heated in excess of 60° C, irreversible coagulative necrosis and tissue desiccation occurs (Hsu et al, 2000). Much like cryoablation, the adequacy and reproducibility of tissue destruction during RFA is dependent upon several parameters: See Chapter 55 for these modalities. Intravenous contrast (iodinated or gadolinium contrast, depending on the imaging technique chosen and the patient’s renal function) is administered and the lesion identified and characterized. A 20-gauge “finder needle” or access sheath is placed near the expected location of the tumor, and imaging is repeated to confirm localization. It is important to note the depth of the finder needle for subsequent biopsy and probe placement. Using this finder needle as a guide, an 18-gauge Tru-Cut (Cardinal Health, Dublin, OH) core biopsy needle is inserted percutaneously, and positioning is again confirmed with repeat imaging. Specimens are obtained and sent for permanent section. The cryoprobe(s) or RFA probe is/are next inserted in a manner similar to that of the core biopsy needle. Cryoprobe positioning and positioning of the RFA probe, and prongs are again confirmed with repeat imaging. Treatment is carried out in a fashion similar to that described for laparoscopic cryoablation/radiofrequency ablation (Fig. 56-2). Again, a “track burn” can be performed with percutaneous RFA. With percutaneous cryoablation, the cryoprobe is removed and repeat imaging performed to evaluate for potential bleeding. With either technique, a postablation contrasted imaging study is performed to determine the adequacy of treatment. Interpretation of treatment success following renal tumor ablation remains a controversial subject. Much of this confusion stems from a lack of uniformity with regard to follow-up protocols (frequency of follow-up and the type of imaging study chosen), as well as a lack of consensus regarding radiographic parameters of success and the role of renal biopsy. The aggregate duration of follow-up with either cryoablation or RFA is inadequate to determine if radiographic follow-up alone is a sufficient surrogate of treatment efficacy (Boorjian and Uzzo, 2009). Routine postablative biopsy may serve a role in corroborating radiographic findings. However, the interpretation of biopsy findings following ablation is highly contentious, and the overall utility of biopsy in this setting is unresolved. Selective biopsy is appropriate and indicated in cases of suspected recurrence. Given these ambiguities, urologists who employ ablative technologies should be familiar with existing and ongoing evidence-based literature and must place these findings in the context of clinical judgment and the overall well being of the patient. As no pathologic margins are rendered with in-situ ablation, imaging characteristics serve as a surrogate marker of treatment efficacy. In general, the complete loss of contrast enhancement on follow-up CT or MRI is considered evidence of complete tissue destruction and attendant treatment success (Matsumoto et al, 2004; McAchran et al, 2005). If a lesion demonstrates persistent contrast enhancement following treatment or requires more than one ablation session to achieve loss of contrast enhancement, this is considered incomplete ablation (Matin et al, 2006). Conversely, if a lesion demonstrates an initial complete loss of contrast enhancement and later demonstrates enlargement of the lesion and/or contrast enhancement, this is considered local tumor recurrence or progression (Novick et al, 2009). In addition to contrast-related characteristics, lesions that undergo cryoablation or RFA demonstrate characteristic but strikingly different appearances on follow-up imaging. The majority of lesions treated with cryoablation demonstrate a greater than 50% reduction in size in the first year following treatment (Deane and Clayman, 2006; Kawamoto et al, 2009). This contraction is due to cellular breakdown and phagocytosis. Conversely, lesions treated with RFA often do not demonstrate postablative contraction, and failure to do so should not be interpreted as a treatment failure. Enlargement of a lesion, regardless of the treatment modality or the enhancement characteristics, should be construed as an ominous sign of local tumor recurrence with biopsy and/or treatment (observation vs. repeat ablation vs. extirpative surgery) dictated by the size and location of the lesion, the response to the original treatment, and the patient’s overall and renal functional status.
Cryoablation
Mode of Action and Experimental Data
Radiofrequency Ablation
Mode of Action and Experimental Data
Surgical Technique
Transperitoneal/Retroperitoneal Laparoscopic Renal Cryoablation/Radiofrequency Ablation
Percutaneous Renal Cryoablation/Radiofrequency Ablation under Ultrasonography, CT, or MRI
Interpretation of Success and Follow-up after Tumor Ablation
Radiographic Interpretation of Success
Stay updated, free articles. Join our Telegram channel
Full access? Get Clinical Tree
Ablative Therapy for Renal Tumors
• Widespread use of cross-sectional imaging has led to a dramatic increase in the number of incidentally discovered, low-stage renal lesions.
1. Treatment Temperature. Based on experimental evidence, it is clear that a certain threshold temperature must be reached in order to effect adequate tumor destruction. Although susceptibility to cryoablation is dependent upon the tissue treated, it is widely estimated that the critical temperature for irreparable tissue destruction is between −20° C and −50° C (Neel, 1971; Tatsutani et al, 1996; Woolley et al, 2002). In general, normal renal parenchyma is destroyed at −19.4° C with tumor cells requiring lower temperatures, likely owing to their more fibrous nature (Chosy et al, 1998; Larson et al, 2000). Tissue with high-collagen and elastin content appear to be the most resilient to freezing (Shepherd and Dawber, 1984). For this reason, the renal collecting system and renal vasculature tolerate cryoablation without appreciable long-term adverse effects (Sung et al, 2003). Conversely, the urethra, bowel, and ureter are more cryosensitive and are thus subject to stricture, sloughing, and perforation if protective measures are not employed (Wong et al, 1997). With the use of modern cryoablation units, core treatment temperatures of −130° C to −150° C are achieved. Currently, the minimum preferred target temperature during renal cryoablation is at or below −40° C.
2. Double Freeze-Thaw Cycle. In-vivo animal studies initially demonstrated adequate cell kill in normal tissue employing a single freeze-thaw cycle (Weber et al, 1997). However, when applied to tumor cells in small animal models, the single freeze-thaw cycle was found to be inferior to a double freeze-thaw cycle with respect to adequacy of tissue ablation and local tumor control. This finding was confirmed by Woolley and associates (2002) who prospectively compared treatment outcomes in 16 female dogs who underwent either a single or double freeze-thaw cycle. A significantly larger and more adequate area of liquefaction necrosis was noted following a double freeze-thaw cycle. As such, a double freeze-thaw cycle is currently the standard of care during renal tumor cryoablation.
3. Determining an Adequate Ablation Zone. Campbell and colleagues (1998) demonstrated that the aforementioned threshold temperature of −40° C (as evidenced by tissue necrosis) was achieved 3.1 mm inside the edge of the evolving iceball. To guarantee that the tumor is completely ablated with a margin of normal tissue, the iceball is generally carried 5 to 10 mm beyond the edge of the tumor when viewed under real-time imaging (Gill et al, 2000). In addition, thermosensors may be positioned at the tumor margin to ensure that adequate treatment temperatures (−40° C) are achieved at the periphery (Rukstalis et al, 2001). The ability to shape the iceball by placing multiple probes at different locations and to deliver varying amounts of flow through these probes such that target temperatures are consistently achieved constitutes a distinct advantage over single-probe ablation devices such as radiofrequency ablation (Weber and Lee, 2005). Currently, cryoablation of renal tumors should be performed under real-time imaging with the treatment area approximately 1 cm beyond the margin of the tumor.
4. Duration of Treatment. Little data exists regarding the optimal duration of freezing during cryoablation. Auge and colleagues (2006) performed a prospective study in nine female farm pigs in which cryoablation was performed for either 5, 10, or 15 minutes. When the specimens were examined histologically, the pigs treated for 5 minutes demonstrated inadequate tissue necrosis and experienced more postprocedural bleeding. Pigs that were treated for 15 minutes exhibited uniform and consistent necrosis but with a high rate of tissue fracture. The pigs treated in the 10-minute cohort demonstrated adequate cell kill and minimal complications. Although the optimal duration of freezing is not known, in our clinical experience, most tumors can be adequately treated with an initial freeze cycle of 8 to 10 minutes and a second freeze cycle of approximately 6 to 8 minutes.
5. Active versus Passive Thaw. Modern argon-based cryoablation units employ helium gas to actively thaw tissue following iceball formation. Alternatively, the flow of argon gas may be discontinued and the tissue allowed to passively thaw without the use of helium. Although active thawing is clearly more efficient and translates into shorter overall operative times, scant data is available regarding the superiority of tissue destruction with active versus passive thawing (Desai and Gill, 2002). Experimental data from Woolley and colleagues (2002) suggests no difference in the degree or reproducibility of tissue destruction with active or passive thaw processes. Conversely, Klossner and colleagues (2007) examined variables that impacted cryosurgical outcomes using human prostate PC-3 and LNCaP cultures and found passive thawing yielded greater tissue destruction owing to increased solute effects and increased recrystallization at temperatures between −20° C and −30° C. Unfortunately, no prospective clinical data exists that specifically addresses the issue. We prefer to use a passive thaw following our initial freeze cycle such that temperatures of less than −40° C are maintained for a longer period of time. We employ an active thaw following the second freeze cycle such that potential bleeding can be more rapidly addressed following probe removal.
• Cryoablation employs argon gas–based systems to achieve treatment temperatures of less than −40° C.
1. Amount of RF Energy/Treatment Temperature: The volume of tissue necrosis is directly correlated to the total amount of RF energy deposited into the tissue less local and systemic heat loss (Zevas and Kuwayama, 1972; McGahan and Dodd, 2001). As stated, alternating RF current creates cellular agitation and, as a result of electrical impedance of the tissue, local heating. Provided that electrical impedance remains low, an expanding sphere of tissue damage emanates outward from the treatment probe. If current is administered too rapidly or the amount of RF energy applied is too high, charring occurs, which reduces the water content of the tissue, abruptly increases impedance, decreases energy transfer, and halts the heating process (Djavan et al, 2000; Finelli et al, 2003). The lesion volume is then either smaller than desired or lacks uniformity. In general, treatment temperatures well above 105° C are avoided for this reason (Goldberg et al, 2000). In clinical practice, a mean treatment temperature of 105° C is preferred (Carraway et al, 2009).
2. Duration of RF Treatment: In general, irreversible protein denaturation and cell kill occurs if a temperature of 46° C is maintained for 1 hour, or 50° C is maintained for between 4 to 6 minutes (Goldberg and Dupuy, 2001). Tissue destruction is nearly instantaneous when treatment temperatures exceed 60° C. As most modern RFA treatment systems consistently achieve temperatures at or above 100° C, the duration of treatment would not appear to be a defining parameter. With modern temperature-based and impedance-based generators, the duration of ablation is largely determined by the treatment system and the size of the lesion. Although no clinical data explicitly addresses the optimal duration of RF treatment, tumors less than 2 cm are typically treated with two cycles of 5 minutes; tumors 2 to 3 cm are treated with two cycles of 7 minutes; and tumors greater than 3 cm are treated with two cycles of 8 minutes (Park et al, 2006a).
3. Wet versus Dry RF: Radiofrequency energy can be applied using needles placed directly into tissue (dry RFA), or with ionic solutions perfused into tissues to decrease carbonization and act as an energy conductor (wet RFA). Early dry RFA systems were highly successfully in treating small lesions but encountered tissue impedance and an attendant lack of efficacy in treating lesions greater than 4 cm. In the late 1990s, Lorentzen and colleagues (1997) and Goldberg and colleagues (1996) independently introduced the concept of driving chilled saline through the shaft of the treatment needle (wet RFA). By cooling the tip of the probe, they discovered that tissue charring immediately adjacent to the electrode was minimized; impedance and ionic agitation was preserved as a consequence, and a larger overall treatment lesion was ultimately created. The success of wet RFA in producing a reliably larger area of necrosis was confirmed in subsequent studies (Collyer et al, 2001; Leveillee and Hoey, 2003; Pereira et al, 2004). Internally cooled ablation probes and the use of hypertonic saline have both been proposed as an alternative to cooled saline and have demonstrated mixed success (Miao et al, 1997; Muvner et al, 2002).
4. Single-Tine versus Multitine Electrode: The original RF needle electrodes were dry RF devices, with varying amounts of insulation at the distal tip governing the approximate size of the treatment lesion. That is, the size of the lesion and the approximate depth of treatment could be modified by exposing varying amounts of tissue to the uninsulated needle. However, the initial ablation probes were capable of treating tumors no greater than 2 cm (McGahan et al, 1993). Larger tumors or the acquisition of an adequate tumor margin required additional treatment probes or re-treatment of overlapping regions. In an attempt to achieve a larger overall treatment volume, LeVeen (1997) introduced an insulated monopolar probe with multiple uninsulated prongs that function as RF antennae for the wider dispersion of current. These prongs are deployed in an even fashion to create a spherical lesion. When high impedance is encountered at one prong, current is redirected to areas of lower impedance. Clinical validation studies have demonstrated more complete necrosis and superior treatment outcomes with multitine electrodes (Rossi et al, 1998; Curley et al, 2000; Rehman et al, 2004).
5. Monopolar versus Bipolar RF: RF energy can be delivered through bipolar or monopolar electrodes. Monopolar RF devices achieve this effect by dispersing current from the device through the patient to a grounding pad on the skin of the patient. Conversely, bipolar RF devices generate current between two electrodes (one active and one negative) in the target tissue. The purported advantage of bipolar energy is that significantly higher temperatures are induced compared with those of monopolar devices (Nakada et al, 2003). It is unclear whether this theoretical advantage translates into improved operative outcomes.
6. Heat-Based versus Impedance-Based Generators: RF energy can be delivered using impedance or temperature-based feedback. As is implied by the name, impedance-based systems (Cool-tip, Valleylab, Boulder, CO; RF 3000, Boston Scientific, Natick, MA) constantly monitor the level of impedance at the tip(s) of the electrode(s) and terminate treatment when “infinite impedance” is encountered. This implies complete desiccation and charring such that electrical current is unable to pass through tissue. Conversely, temperature-based systems (RITA Model 1500X, AngioDynamics, Queensbury, NY) determine completion of treatment when the tissues adjacent to the probe have reached a target temperature for a predetermined duration of time. Experimental data is contradictory. Rehman and colleagues (2004) noted areas of incomplete necrosis with impedance-based systems and complete necrosis with temperature-based systems. However, a dedicated animal study by Gettman and colleagues (2002a) demonstrated similar efficacy in ablating renal tissue with the two types of generators. There is no explicit clinical data that supports the superiority of impedance or temperature-based systems.
7. “Heat-Sink” Phenomenon: Treatment efficacy is dependent on the total amount of RF energy applied to the tissue, multiplied by local tissue interactions, less systemic and local heat loss (the “bioheat transfer equation”) (Pennes, 1948; Goldberg et al, 2000). When the target zone is highly vascularized or is adjacent to large vessels, thermal energy is preferentially dispersed to the comparatively cooler blood within these vessels. This heat-sink effect may therefore spare tumor cells in close proximity to large blood vessels and lead to treatment failures. In response to this shortcoming, investigators within and outside urology have evaluated and employed methods to temporarily interrupt blood flow to the target organ (Corwin et al, 2001; Chang et al, 2004). The collective experience with hepatovascular occlusion (by transcatheter arterial embolization/balloon occlusion or the Pringle maneuver) during RFA has been favorable with significantly larger treatment areas and improved overall outcomes (Curley et al, 1999; Rossi et al, 2000). Success with renovascular occlusion has not yet been demonstrated. No significant difference in lesion size was noted between the clamped and unclamped cohorts in two separate studies (Corwin et al, 2001; Marcovich et al, 2002). Given the risk of postischemic injury and the technical complexity of hilar dissection and cross-clamping, renal vascular occlusion is not routinely performed during RFA.
8. Treatment under Image Guidance: Perhaps the most significant limitation of RFA, to date, has been the inability to reliably monitor treatment under image guidance. With cryoablation, the evolving iceball can be monitored in real time and its hyperechoic edge used as direct clinical evidence of treatment adequacy. Owing to the low intrinsic contrast between untreated and ablated tissue, as well as the formation of gas bubbles during heating, monitoring the RF ablation zone with ultrasonography, CT, and/or MRI has proven difficult (Rendon et al, 2001; Renshaw, 2004). Certainly, accurate probe positioning can be achieved with intraoperative ultrasonography (laparoscopic RFA) or under ultrasonography, CT, or MRI guidance (percutaneous RFA). However, once the probe is appropriately positioned, the desired treatment zone becomes entirely dependent on the deployed diameter and configuration of the electrode. And although impedance and temperature-based feedback ensures tissue destruction at the tip(s) of the electrode(s), the global geometric treatment area cannot be directly appreciated as readily as with cryoablation. In order to compensate for these “indirect” cues and to ensure a negative treatment margin, an ablation zone 5 to 10 mm beyond the maximum radiographic tumor diameter is preferred (Carraway et al, 2009).
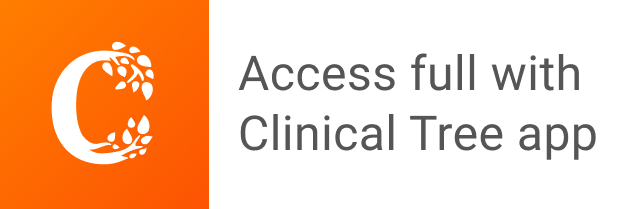