Chapter Outline
RENIN-ANGIOTENSIN-ALDOSTERONE SYSTEM, 325
Classical Renin-Angiotensin-Aldosterone System, 326
Expanded Renin-Angiotensin-Aldosterone System: Enzymes, Angiotensin Peptides, and Receptors, 331
Renin-Angiotensin-Aldosterone System in Kidney Pathophysiology, 334
ENDOTHELIN, 335
Structure, Synthesis, and Secretion of the Endothelins, 335
Endothelin Receptors, 336
Physiologic Actions of Endothelin in the Kidney, 336
Role of Endothelin in Essential Hypertension, 336
Role of Endothelin in Renal Injury, 337
Endothelin System in Chronic Kidney Disease and Diabetic Nephropathy, 337
Endothelin System and Other Kidney Diseases, 338
Safety Profile of Endothelin Receptor Antagonists, 338
NATRIURETIC PEPTIDES, 339
Structure and Synthesis of the Natriuretic Peptides, 339
Natriuretic Peptide Receptors, 341
Neutral Endopeptidase, 341
Actions of the Natriuretic Peptides, 342
Natriuretic Peptides as Biomarkers of Disease, 343
Therapeutic Uses of Natriuretic Peptides, 344
Combination Angiotensin Receptor Blockers and Neutral Endopeptidase Inhibitors, 345
Other Natriuretic Peptides, 346
KALLIKREIN-KININ SYSTEM, 346
Components of the Kallikrein-Kinin System, 346
Plasma and Tissue Kallikrein-Kinin System, 347
Renal Kallikrein-Kinin System, 348
Kallikrein-Kinin System in Renal Disease, 349
UROTENSIN II, 351
Synthesis, Structure, and Secretion of Urotensin II, 351
Physiologic Role of Urotensin II, 351
Urotensin II in the Kidney, 352
Observational Studies of Urotensin II in Renal Disease, 352
Interventional Studies of Urotensin II in the Kidney, 352
Vasoactive peptides, arising from both the systemic circulation and local tissue-based generation, play important roles in kidney physiology, not only in the regulation of renal blood flow but also in electrolyte exchange, acid-base balance, and diuresis. More recent interest has focused on the role of these peptide systems in kidney development and in the pathogenesis of organ injury.
Renin-Angiotensin-Aldosterone System
In their now seminal 1898 report, Niere und Kreislauf, Robert Tigerstedt and Per Bergman, while working at the Karolinska Institute in Sweden, described the prolonged vasopressor effects of crude kidney extracts. Although recognizing the impurity of the extract, Tigerstedt named the unidentified active substance “renin,” based on its organ of origin. More than 110 years later, our understanding of the renin-angiotensin-aldosterone system (RAAS) continues to evolve with insights into its pivotal role in pathophysiologic and physiologic processes. Underlying this effort to fully understand the RAAS is not only a desire for knowledge but a profound appreciation of the therapeutic importance of its blockade. Much of this insight is derived from work in 1985 by Anderson and colleagues, who defined the renoprotective effects of angiotensin-converting enzyme (ACE) inhibition in a rodent model of progressive kidney disease.
Classical Renin-Angiotensin-Aldosterone System
The classical view of the RAAS focuses on the endocrine aspects of this peptidergic system. Angiotensinogen synthesized by the liver enters the circulation, where it is cleaved to form angiotensin I by renin, a peptidase that is secreted from the juxtaglomerular apparatus (JGA) of the kidney. The terminal two amino acids of angiotensin I are then removed to form angiotensin II, as it traverses through the circulation, exposed to ACE, a peptidase expressed on endothelial cells, particularly in the pulmonary vasculature. Angiotensin II, the principal effector molecule of the RAAS, then binds to its type 1 receptor, resulting in vasoconstriction, sodium retention, thirst, and aldosterone secretion. This traditional view of the RAAS is still valid but has been considerably augmented, not only by the discovery of new enzymes, peptides, and receptors but also by an appreciation that in addition to its endocrine paradigm, the RAAS also has an independently functioning local tissue-based component that acts through paracrine, autocrine, and possibly intracrine mechanisms ( Figure 13.1 ).

Angiotensinogen
Angiotensinogen is primarily, though by no means exclusively, synthesized in the liver, particularly the pericentral zone of the hepatic lobules. In humans it is coded by a single gene, composed of five exons and four introns, that spans approximately 13 kb of genomic sequence on chromosome 1 (1q42-q43). It is translated to a 453–amino acid globular glycoprotein with a molecular weight between 45 and 65 kd, depending on the extent of its glycosylation, which then undergoes posttranslational cleavage of a 24– or 33–amino acid signal peptide, giving rise to the mature circulating form of angiotensinogen.
Structurally angiotensinogen bears substantial homology to the serpin superfamily of protease inhibitors and like many members of its family behaves as an acute phase reactant in the inflammatory setting, reflecting the presence of an acute phase response element that binds the transcription factor nuclear factor κ-light-chain-enhancer of activated B cells (NF-κB).
Renin
Like the gene encoding angiotensinogen, the gene encoding renin is located on the long arm of chromosome 1 (1q32) and contains 10 exons and 9 introns, similar to other aspartyl proteases. Unlike humans and rats, which have only a single renin gene, the mouse has two, Ren1 and Ren2 , expressed primarily in the submandibular gland and kidney, respectively.
Following its synthesis as a 406–amino acid preprohormone, the 23–amino acid leader sequence of preprorenin is cleaved in the rough endoplasmic reticulum, giving rise to prorenin (also called inactive renin and “big” renin), which may be then rapidly secreted directly from the Golgi apparatus or from protogranules. Alternatively, and virtually exclusively in the JGA, prorenin may be packaged into mature, dense granules that instead of being immediately secreted, undergo further processing to the active enzyme, renin (active renin). In contrast to the more constitutive secretion of prorenin, release of renin-containing granules is tightly regulated.
Mature, active renin is a variably glycosylated 37- to 40-kd aspartyl protease that is active at neutral pH, and in contrast to the more promiscuous activities of most other proteases in this class, has only a single known substrate, cleaving the decapeptide angiotensin I from the amino-terminal of angiotensinogen. Whereas the kidney produces both renin and prorenin, a range of extrarenal tissues, including the adrenals, gonads, and placenta, produce prorenin and contribute to its presence in plasma. However, as evidenced by the near-total absence of active renin in anephric patients, the kidney, and the JGA in particular, appears to be the only source of circulating renin in humans.
Factors that chronically stimulate renin secretion, such as a low-sodium diet and ACE inhibition, lead to an increase in the number of renin-secreting cells rather than an increase in cell size or the number of granules that each JGA cell contains. This expansion of the renin-secreting mass occurs proximally by metaplastic transformation of smooth muscle cells within the walls of the afferent arteriole. Although sometimes mentioned, ectopic renin expression within the extraglomerular mesangium appears to be an extraordinarily rare event.
Prorenin Activation
Prorenin is maintained as an inactive zymogen through the occupation of its catalytic cleft by its prosegment. Removing this prosegment by either proteolytic or nonproteolytic means yields active renin, a term that denotes its enzymatic activity rather than its amino acid sequence ( Figure 13.2 ).

Within the dense core secretory granules of the JGA, acidification by vacuolar adenosine triphosphatases (ATPases) provides the optimal pH for the prosegment-cleaving enzymes: proconvertase 1 and cathepsin B and may also assist the pH-dependent, non-enzymatic activation of prorenin as well. Although various peptidases such as trypsin, plasmin, and kallikrein can also cleave the prosegment of prorenin in vitro, these do not appear to contribute to the generation of renin in the in vivo setting. Although traditionally viewed as occurring only in the JGA, cell culture–based studies suggest that proteolytic activation of renin can also occur in cardiac and vascular smooth muscle cells by as-yet-unidentified serine proteases. The significance of these findings in the intact organism, however, remains to be established.
In addition to proteolytic cleavage of its prosegment, prorenin can also be reversibly activated nonenzymatically by a conformational change such that the prosegment no longer occupies the enzymatic cleft. Under usual circumstances, less than 2% of prorenin is in this open active conformation. This process can, however, be induced by acid (pH < 4.0) and to a lesser extent by cold. In a later study the putative (pro)renin receptor (see later) was also shown to nonproteolytically activate prorenin.
Regulation of Renin Secretion
Mechanical, neurologic, and chemical factors regulate the activity of the RAAS by modulating renin secretion.
Renal Baroreceptor
The existence of a renal baroreceptor mechanism was first conceptualized by Skinner and associates to explain how renin secretion increases when afferent arteriolar perfusion pressure falls. Studies in conscious dogs show that changes in renal perfusion pressure have only a small effect on renin secretion until a threshold of approximately 90 mm Hg is reached, below which renin secretion abruptly increases, doubling with every 2– to 3–mm Hg fall in pressure. Accordingly, reduction in pressure below this level profoundly stimulates renin secretion, thereby acutely activating the RAAS and resulting in a range of angiotensin II–dependent phenomena that collectively serve to restore systemic pressure. Despite the importance of the baroreceptor function, several decades of research have not identified precisely how the pressure signal is transduced into renin release, though postulated mediators include stretch-activated calcium channels, endothelins (ETs), and prostaglandins.
Neural Control
The JGA is endowed with a rich network of noradrenergic nerve endings and their β 1 receptors. Stimulation of the renal sympathetic nerve activity leads to renin secretion that is independent of changes in renal blood flow, glomerular filtration rate (GFR), or Na + resorption. Moreover, this effect can be blocked surgically (by denervation) and pharmacologically (by the administration of β-adrenoreceptor blockers). The role of cholinergic, dopaminergic, and adrenergic activation is controversial, though these agents have also been shown to modulate renin release under certain circumstances.
Tubular Control
Chronic diminution in luminal NaCl delivery to the macula densa is a potent stimulus for renin secretion, reflecting a coordinate interaction between a range of mediators, including adenosine, nitric oxide, and prostaglandins, that affect not only renin release but also its transcription. This mechanism is thought to account for the chronically high plasma renin activity (PRA) in subjects who adhere to a low-salt diet.
Metabolic Control
The tricarboxylic acid cycle provides a final common pathway by which carbohydrates, fatty acids, and amino acids converge in the process of adenosine triphosphate (ATP) generation by aerobic electron transfer. Although the tricarboxylic acid cycle operates within mitochondria, its intermediates can be detected within the extracellular space, increasing in abundance when local energy supply and demand are mismatched or when cells are exposed to hypoxia, toxins, or injury. Succinate, for instance, has been shown to stimulate renin release, and its intravenous administration leads to hypertension, though the mechanisms underlying this effect have only recently been unravelled. In 2004, He and colleagues reported that α-ketoglutarate and succinate are ligands for the previously orphaned G protein–coupled receptors GPR90 and GPR99, respectively, and that succinate-induced hypertension is abolished in GPR91-deficient mice. Notably, in other studies, GPR91 was localized to the apical plasma membrane of macula densa cells, where succinate stimulation was shown to activate p38 and extracellular signal–regulated kinases 1 and 2 (ERK1/2) mitogen-activated protein (MAP) kinases, inducing cyclo-oxygenase-2–dependent synthesis of prostaglandin E 2 , a well-established paracrine mediator of renin release. Moreover, the ability of tubular succinate to induce juxtaglomerular renin secretion suggests that this phenomenon is likely an important determinant of JGA function in both physiologic and pathophysiologic settings.
Other Local Factors
In addition to the factors discussed earlier, a large range of locally active factors have also been shown to alter renin secretion. These include peptides (atrial natriuretic peptide, kinins, vasoactive intestinal polypeptide, ET, calcitonin gene–related peptide), amines (dopamine and histamine), and arachidonic acid derivatives.
Plasma Prorenin and Renin
Under usual circumstances the plasma concentration of prorenin is approximately 10 times greater than renin. In some patients with diabetes, however, the plasma prorenin level is disproportionately increased, where it predicts the development of diabetic nephropathy (including microalbuminuria) and retinopathy.
In addition to its role in the research setting, measurement of plasma renin concentration (PRC) is an important clinical assay, providing important information, for example, when evaluating patients with possible hyperaldosteronism, assessing volume status, and in predicting the response to, or monitoring drug adherence to, an ACE inhibitor or angiotensin receptor blocker (ARB). In broad terms, PRC is determined by either activity or immunologic assay methods. The most commonly used method involves the measurement of PRA. With this method the rate at which angiotensin I is produced from plasma angiotensinogen is assayed. To prevent the degradation of angiotensin I or its conversion to angiotensin II, inhibitors of angiotensinase and ACE are added to the assay. Accordingly, PRA is not only dependent on renin and endogenous angiotensinogen concentrations but will also overestimate the extent of inhibition by renin inhibitors because of the displacement of protein-bound drug by the peptidase inhibitors. The latter scenario may be diminished by using an antibody capture method in which anti–angiotensin I antibody, instead of peptidase inhibitors, is used to protect angiotensin I from further catabolism.
The nomenclature of renin assays can be quite confusing in that PRC may be measured by both activity and immunologic assays. With the plasma renin concentration activity assay (PRCa), exogenous angiotensinogen is added to the assay, thereby avoiding the influence that endogenous levels of the substrate might have. However, PRCa may also be affected by the presence of renin inhibitors; as with PRA, this effect may be reduced by antibody capture methodology. In the plasma renin concentration immunologic assay (PRCi), the concentrations of renin and prorenin in its active, open conformation are assessed, so that the PRCi assay, like PRA and PRCa, is time and temperature dependent because lower temperatures will increase the proportion of prorenin in its active conformation. Moreover, renin inhibitors, by binding to the active site of prorenin in its open conformation, prevent the refolding of the prosegment and may therefore lead to an overestimation of PRCi.
Angiotensin-Converting Enzyme
ACE is a zinc-containing dipeptidyl carboxypeptidase that cleaves the terminal histidyl-leucine from angiotensin I to form the octapeptide angiotensin II. In contrast to the single-substrate specificity of renin, ACE is not specific, cleaving the two terminal acids from peptides with the C′-terminal sequence R 1 -R 2 -R 3 -OH, in which R 1 is the protected (noncleaved) amino acid, R 2 is any nonproline l -amino acid, and R 3 is any nondicarboxylic (cysteine, ornithine, lysine, arginine) l -amino acid with a free carboxy-terminal. Importantly, therefore ACE also catalyzes the inactivation of bradykinin. Although encoded by a single ACE gene, two distinct tissue-specific messenger RNAs (mRNAs) are transcribed, each with different initiation and alternative splice sites. The somatic form, present in almost all tissues, is a 1306–amino acid, 140- to 160-kd glycoprotein with two active sites, whereas the 90- to 100-kd testicular or germinal form is found exclusively in postmeiotic male germ cells, contains a single active site, and appears to be involved with spermatogenesis. The somatic form of ACE is widely distributed with activity present not only in tissues but also in most biologic fluids. In the human kidney, ACE is present to the greatest extent within proximal and distal tubules; however, both the magnitude of expression and its site-specific distribution may be altered by disease.
Angiotensin Type 1 Receptor
The angiotensin type 1 (AT 1 ) receptor mediates most of the known physiologic effects of angiotensin II. The gene for this widely distributed 359–amino acid, 40-kDa, seven-transmembrane G protein–coupled receptor is located on chromosome 3 in humans.
Within the kidney, AT 1 receptors are widely expressed. In the glomerulus, they are found in both afferent and efferent arterioles as well as in the mesangium, in endothelium, and on podocytes. Consistent with the role of angiotensin II in Na + resorption, AT 1 receptors are highly abundant on the brush borders of proximal tubular epithelial cells. Prominent expression has also been found in renal medullary interstitial cells, located between the renal tubules and vasa recta, where angiotensin II is purported to have a potential role in the regulation of medullary blood flow.
Angiotensin II binding to the AT 1 receptor initiates cell signaling by several different pathways that have been mostly studied in vascular smooth muscle cells. These include G protein–mediated pathways and the activation of tyrosine kinases, reduced nicotinamide adenine dinucleotide (NADH)/reduced nicotinamide adenine dinucleotide phosphate (NADPH) oxidases, and serine/threonine kinases.
G Protein–Mediated Signaling
In the classical G protein–mediated pathway, AT 1 receptor ligand binding leads to activation of phospholipases C, D, and A 2 . Phospholipase C rapidly hydrolyses phosphatidylinositol bisphosphate to inositol trisphosphate and di-acyl glycerol (DAG), initiating calcium release from intracellular stores and protein kinase C (PKC) activation, respectively. Phospholipase D similarly generates DAG and activates PKC, whereas phospholipase A 2 leads to the formation of various vasoactive and proinflammatory arachidonic acid derivatives.
Reactive Oxygen Species
Although reactive oxygen species were previously regarded as toxic waste products, emerging evidence indicates that they may also act as second messengers, activating not only other cell signaling cascades such as p38 MAP kinase but also a number of transcription pathways implicated in the pathogenesis of inflammatory and degenerative disease. Although the mechanisms by which the AT 1 receptor stimulates NADH/NADPH are not well understood, angiotensin II binding to this receptor results in the generation of both superoxide and hydrogen peroxide.
Tyrosine Kinases
Angiotensin II binding to the AT 1 receptor “transactivates” a number of nonreceptor tyrosine kinases (Src, Pyk2, FAK, and JAK), as well as the growth factor receptor tyrosine kinases for epidermal growth factor (EGF) and platelet-derived growth factor (PDGF). By binding to the AT 1 receptor, angiotensin II initiates the translocation of tumor necrosis factor-α (TNF-α)–converting enzyme (TACE) to the cell surface. TACE then cleaves TNF-α from its membrane-associated precursor (pro–TNF-α), allowing it to bind to EGF receptor (EGFR) on the cell surface. This ligand-receptor interaction then induces EGFR autophosphorylation and activates its downstream signaling pathways that include Akt, ERK1/2, and mammalian target of rapamycin (mTOR) ( Figure 13.3 ). The in vivo relevance of this transactivation pathway has been confirmed. Using mice that express a dominant negative form of EGFR, Lautrette and colleagues showed that despite similar blood pressures, mutant mice infused with angiotensin II had less proteinuria and renal fibrosis than did their wild-type counterparts. Consistent with these findings and the pivotal role of the RAAS in diabetic nephropathy, studies using the specific EGFR tyrosine kinase inhibitor PKI 166 have also shown a reduction in early structural injury in a rat model of diabetic nephropathy.

Like EGFR, the transactivation of the PDGF receptor (PDGFR) by the AT 1 receptor is also complex, involving the adaptor protein Shc. In addition to studies that have explored the angiotensin-PDGFR interaction in cell culture or organ baths, a later report has shown that despite continued hypertension, inhibition of the PDGFR kinase in vivo can also dramatically attenuate angiotensin II–induced vascular remodeling.
Angiotensin Type 1 Receptor Internalization
In addition to the conventional ligand-receptor–mediated pathways, a range of other signaling mechanisms that involve the AT 1 receptor have been described. These include the discovery of receptor-interacting proteins, heterologous receptor dimerization, and ligand-independent activation ( Figure 13.4 ). These new insights, though adding greater complexity to our understanding of the RAAS, also provide the potential for new therapeutic targets in disease prevention and management.

Following ligand binding and the initiation of signal transduction, AT 1 receptors are rapidly internalized, followed by either lysosomal degradation or recycling back to the plasma membrane. Several mechanisms account for AT 1 receptor internalization, including interaction with caveolae, phosphorylation of its carboxy-terminal by G protein–coupled receptor kinases, and association with the newly described AT 1 receptor interacting proteins. To date, two such interacting proteins, AT 1 receptor–associated protein (ATRAP) and AT 1 receptor–associated protein 1 (ARAP1), have been described. ATRAP interacts with the C terminus of AT 1 receptor, downregulating cell surface AT 1 receptor expression and attenuating angiotensin II–mediated effects. ARAP1 though somewhat similar to ATRAP, promotes AT 1 receptor recycling to the plasma membrane such that its kidney-specific overexpression induces hypertension and renal hypertrophy.
Angiotensin Type 1 Receptor Dimerization
In addition to their ability to induce cell signaling in their monomeric state, G protein–coupled receptors such as AT 1 receptor may also associate to form both homodimers and heterodimers. Beyond its constitutive homodimerization, AT 1 receptor may dimerize with angiotensin type 2 (AT 2 ) receptor and also form hetero-oligomers with receptors for bradykinin (B 2 ), epinephrine (β 2 ), dopamine (D1, D3, and D5), endothelin type B, Mas, and EGF that modulate their function.
Ligand-Independent Angiotensin Type 1 Receptor Activation
Without involvement of angiotensin II, cell stretch induces a conformational switch that initiates intracellular signaling pathways of the AT 1 receptor. As might be expected from an understanding of this mechanism, an AT 1 receptor blocker, acting as an inverse agonist, will abrogate these effects, as described in both cardiac and mesangial cells. A similar means of ligand-independent activation has also been shown to result from the binding of agonist antibodies to AT 1 receptors in some women with preeclampsia and in certain cases of renal allograft rejection.
Physiologic Effects of Angiotensin II in the Kidney
The traditional actions of angiotensin II relate primarily to its effects on vascular tone and fluid balance that are mediated by its actions on the vasculature, heart, kidney, brain, and adrenal glands by the AT 1 receptor. In vascular smooth muscle, stimulation of AT 1 receptors by angiotensin II induces cell contraction and consequent vasoconstriction. In the adrenal cortex, this ligand-receptor interaction stimulates aldosterone release, thereby promoting sodium resorption in the distal nephron. Moreover, angiotensin II will directly enhance sodium retention by the proximal tubule, and in the brain it will stimulate thirst and salt craving. Additional effects include sympathoadrenal stimulation and the augmentation of cardiac contractility. Together these effects serve to maintain extracellular fluid volume and systemic blood pressure. Given the central role that the kidney has in the regulation of these key aspects of mammalian homeostasis, it is not surprising that angiotensin II should have profound effects on renal physiology.
Hemodynamic Actions
The effects of exogenously administered angiotensin II are dose dependent. At low doses, angiotensin II infusion increases renal vascular resistance and lowers renal blood flow without affecting GFR so that the filtration fraction is increased. At higher doses of angiotensin II, renal vascular resistance is further increased, leading to an augmented reduction in renal blood flow and fall in GFR. However, because GFR is reduced to a lesser extent than renal plasma flow, the filtration fraction remains elevated. Such studies are consistent with the view that limited stimulation of the RAAS would mostly serve to enhance tubular sodium levels, as is seen, for instance, in societies unaccustomed to contemporary diets. Greater activation of the RAAS, on the other hand, as might be found in the setting of severe volume depletion, would result in angiotensin II–dependent reduction in renal blood flow that would aid in sustaining systemic blood pressure while further stimulating sodium resorption.
Kidney micropuncture has been used extensively to explore the intrarenal sites of the effects of angiotensin II on vascular resistance. These studies demonstrate that although angiotensin II increases both afferent and efferent arteriolar resistance, glomerular capillary hydraulic pressure (P GC ) is consistently elevated, and the glomerular ultrafiltration coefficient (Kf) is reduced. Moreover, as predicted by mathematical modeling, the glomerular hypertension induced by angiotensin II does not lead to acute proteinuria, because the structural barriers to macromolecular passage remain intact. Chronic angiotensin II infusion with sustained intraglomerular hypertension, by contrast, leads to glomerular capillary damage and substantial proteinuria.
Tubular Transport
Sodium
Consistent with its importance in the regulation of volume status, angiotensin II has profound effects on renal Na + handling. The proximal tubule is responsible for the resorption of approximately two thirds of the sodium from the glomerular filtrate, and binding sites for angiotensin II are particularly abundant in the proximal tubule with immunohistochemical localization of the AT 1 receptor to both apical and basolateral surfaces. At picomolar concentrations, angiotensin II stimulates the luminal Na + -H + -exchanger, the basolateral Na + -HCO 3 − cotransporter, and sodium-potassium adenosine triphosphatase (Na + -K + -ATPase). However, at concentrations higher than 10 −9 M, angiotensin II inhibits the very same transporters. The mechanisms underlying this dose-dependent effect of angiotensin II on Na + transport, which seem to also occur in the loop of Henle, are incompletely understood. In the distal tubule the effects of angiotensin II on Na + transport are site dependent. In the early distal tubule, for instance, angiotensin II stimulates apical Na + -H + exchange, whereas in the late distal tubule it stimulates the amiloride-sensitive sodium channel.
Acid-Base Regulation
The kidney has a key role in the maintenance of physiologic pH by regulating the secretion and resorption of acids and bases. As for Na + , angiotensin II also has substantial effects on acid-base transport in the proximal tubule, distal tubule, and collecting duct. Interest has focused in particular on its actions in the collecting duct. In the collecting duct, angiotensin II not only stimulates Na + -H + -exchangers and Na + -HCO 3 − cotransporters but has also been shown to stimulate the vacuolar hydrogen ion adenosine triphosphatase (H + -ATPase) in intercalated A cells via its AT 1 receptor. Moreover, elegant and detailed electron microscopic studies have helped to unravel the mechanisms by which angiotensin II exerts its effects at this site, revealing translocation of the H + -ATPase from the cytoplasm to the apical surface in response to ligand stimulation.
Expanded Renin-Angiotensin-Aldosterone System: Enzymes, Angiotensin Peptides, and Receptors
Angiotensin Type 2 Receptor
In humans the AT 2 receptor is a 363–amino acid protein that maps to the X chromosome and is highly homologous to its rat and mouse counterparts. Like AT 1 receptor, AT 2 receptor is also a seven-transmembrane G protein–coupled receptor, though it shares only 34% homology.
Despite substantial research, the actions of AT 2 receptor are still not well understood and remain somewhat controversial. In general, however, the actions of AT 2 receptor stimulation oppose those of AT 1 receptor. For instance, whereas AT 1 receptor vasoconstricts and promotes Na + retention, AT 2 receptor stimulation leads to vasodilation and natriuresis, consistent with its abundance on the epithelium of the proximal tubule. The vasodilatory effects of AT 2 receptor stimulation are mediated by increasing nitric oxide synthesis and cyclic guanosine monophosphate (cGMP) by bradykinin-dependent and bradykinin-independent mechanisms. Its natriuretic effects, however, seem to be dependent on conversion of angiotensin II to angiotensin III by aminopeptidase N.
Like the activity of the AT 1 receptor, the activity of the AT 2 receptor may also be modulated by oligomerization, association with various interacting proteins, and ligand-independent effects.
(Pro)Renin Receptor
In 2002 an apparently novel, 350–amino acid, single-transmembrane protein that binds both renin and prorenin with high affinity was identified. Ligand binding to this protein was shown to induce a fourfold increase in the catalytic cleavage of angiotensinogen as well as stimulating intracellular signaling with activation of MAP kinases ERK1/2, leading to it being named the (pro)renin receptor ([P]RR). The designation (pro)renin refers to its ability to interact with both renin and prorenin.
Given its localization to the mesangium in initial studies, its actions in augmenting local angiotensin II production, and its ability to increase mesangial transforming growth factor-β (TGF-β) production, the (P)RR has understandably been implicated in the pathogenesis of kidney disease. However, despite the appeal, it has been difficult to reconcile this view of the (P)RR with a number of other experimental findings, regarding not only its potentially pathogenetic role, but also its pattern of distribution within the kidney and its homology to other proteins. For instance, given the purported pathogenetic role of the (P)RR, the increased abundance of renin that follows the use of ACE inhibitors and ARBs would be expected to be adverse, yet these classes of drugs have been repeatedly shown to be renoprotective. Secondly, although the (P)RR was initially localized to the glomerular mesangium, later, highly detailed studies have shown that it is primarily expressed in the collecting duct. Thirdly, although initially reported as having no homology with any known membrane protein, database interrogation shows that the (P)RR is identical to two other proteins: endoplasmic reticulum–localized type 1 transmembrane adaptor precursor (CAPER) and ATPase, H + transporting, lysosomal accessory protein 2 (ATP6ap2), a protein that associates with the vacuolar H + -ATPase. Indeed, the predominant expression of the (P)RR at the apex of acid-secreting cells in the collecting duct, in conjunction with its co-localization and homology with an accessory subunit of the vacuolar H + -ATPase, suggest that the (P)RR may function primarily in urinary acidification. However, the vacuolar H + -ATPase is not restricted to the kidney but is widely distributed in the plasma membrane and the membranes of organelles in several tissues where it functions, not only in urinary acidification but also in endocytosis, conversion of proinsulin to insulin, and osteoclast bone resorption. Although the prevailing data indicate that (P)RR is an accessory subunit of the vacuolar H + -ATPase that also binds renin and prorenin, the precise functions of the prorenin- and renin-binding subunit remain to be unravelled in the kidney and elsewhere.
Angiotensin-Converting Enzyme 2
In 2000, two groups independently reported the existence of the first ACE homolog, angiotensin-converting enzyme 2 (ACE2), an apparently novel zinc metalloprotease but with considerable homology (40% identity and 61% similarity) to ACE. The gene encoding ACE2, located on the X chromosome (Xp22) contains 18 exons, several of which bear considerable similarity to the first 17 exons of human ACE. Its transcript is 3.4 kb, generating an 805–amino acid peptide that is most highly expressed in kidney, heart, and testis but is also present in plasma and urine. In contrast to ACE, ACE2 functions as a carboxypeptidase, removing the terminal phenylalanine from angiotensin II to yield the vasodilatory heptapeptide angiotensin-(1-7). ACE2 may also indirectly lead to the formation of angiotensin-(1-7) by cleaving the C-terminal leucine from angiotensin I, thereby generating angiotensin-(1-9), which may then give rise to angiotensin-(1-7) under the influence of ACE or neutral endopeptidase (NEP). Thus, ACE2 contributes to both angiotensin II degradation and angiotensin-(1-7) synthesis. Accordingly, ACE and ACE2 were initially viewed as having opposing actions with regard to vascular tone and tissue injury. However, emerging data suggest that the situation is far from clear. For instance, although lentivirus-induced overexpression of ACE2 in the heart exerted a protective influence following experimental myocardial infarction, a later study of cardiac ACE2 overexpression led to cardiac dysfunction and fibrosis, despite lowering systemic blood pressure.
In the kidney, ACE2 co-localizes with ACE and angiotensin receptors in the proximal tubule, whereas in the glomerulus it is predominantly expressed within podocytes and to a lesser extent in mesangial cells, contrasting the endothelial predilection of ACE at that site. Numerous studies have explored changes in ACE2 expression in human kidney disease, as well as in a range of animal models, reporting both increased and decreased levels. Therefore it is uncertain whether increased ACE2 might be detrimental or a beneficial response to injury. With this in mind, the findings of intervention studies are of particular importance.
In experimental diabetic nephropathy, for instance, pharmacologic ACE2 inhibition with MLN-4760 led to worsening albuminuria and glomerular injury ; similar findings were obtained in ACE2 knockout mice that were crossed with the Akita model of type 1 diabetes. As might be expected from these findings, augmenting ACE2 activity by the infusion of human recombinant protein (hrACE2) has been shown to attenuate diabetic kidney injury in the Akita mouse. In this study, hrACE2 not only improved kidney structure and function but also showed that the protective effects were likely due to reduction in angiotensin II and an increase in angiotensin-(1-7) signaling. Finally, in addition to its role in the RAAS, ACE2 has also been shown to be the receptor for the severe adult respiratory syndrome coronavirus.
Angiotensin Peptides
Angiotensin III, or Angiotensin-(2-8)
Formed by the actions of aminopeptidase A, the heptapeptide angiotensin III, also known as angiotensin-(2-8), like angiotensin II, also known as angiotensin-(1-8), exerts its effects by binding to AT 1 receptors and AT 2 receptors. Initially, Angiotensin III was thought to have a predominant role in regulating vasopressin release. However, later studies indicate that while angiotensin III is equipotent to angiotensin II with regard to its effects on blood pressure, aldosterone secretion, and renal function, its metabolic clearance rate is approximately five times as rapid.
Angiotensin IV, or Angiotensin-(3-8)
Angiotensin IV is generated from angiotensin III by the actions of aminopeptidase M. Although some of its actions are mediated by the AT 1 receptor, the majority of the biologic effects of angiotensin IV are thought to result from its binding to insulin-regulated aminopeptidase. Angiotensin IV was previously viewed as inactive, but there has been considerable interest in its actions in the central nervous system (CNS), where it not only enhances learning and memory but also possesses anticonvulsant properties and protects the brain from ischemic injury.
In addition to its CNS effects, angiotensin IV has also been implicated in atherogenesis, principally related to its ability to activate NF-κB and upregulate several proinflammatory factors, which include monocyte chemoattractant protein-1 (MCP-1), intercellular adhesion molecule-1, interleukin-6 (IL-6), and TNF-α, as well as enhancing the synthesis of prothrombotic factor plasminogen activator inhibitor-1. In the kidney, angiotensin IV is reported to have variable effects on blood flow and natriuresis.
Angiotensin-(1-7)
The ostensibly vasodilatory and antitrophic angiotensin-(1-7) may be formed by the actions of several endopeptidases, which include removal of the terminal tripeptide of angiotensin I by NEP, cleavage of the C-terminal phenylalanine of angiotensin II by ACE2, and the excision of the dipeptidyl group from the C terminus of angiotensin-(1-9) by ACE. Evolving evidence indicates that the actions of this heptapeptide are mediated by its binding to the previously orphaned G protein–coupled receptor MasR . Angiotensin-(1-7) induces vasodilation by a number of mechanisms, which include the amplification of the effects of bradykinin, stimulating cGMP synthesis, and inhibiting the release of norepinephrine. In addition, angiotensin-(1-7) inhibits vascular smooth muscle proliferation and prevents neointima formation following balloon injury to the carotid arteries. Contrasting these findings, however, is a report that exogenous angiotensin-(1-7), rather than ameliorating diabetic nephropathy, as might have been predicted based on the prevailing paradigm, actually accelerated the progression of the disease.
Angiotensin-(2-10)
In addition to angiotensin II and the other C-terminal cleavage products discussed earlier, angiotensin I, or angiotensin-(1-10), may also give rise to a number of other potentially biologically active peptides that result from removal of amino acids from its N terminus. Of these, angiotensin-(2-10), produced by the actions of aminopeptidase A, has been found to modulate the pressor activity of angiotensin II in rodents.
Angiotensin-(1-12)
Angiotensin-(1-12) is a dodecapeptide, first isolated in rat intestine but also found to be present in the kidney and heart, that is cleaved from angiotensinogen by a heretofore unidentified nonrenin enzyme. Notably, in the kidney, angiotensin-(1-12), akin to other components of the intrarenal RAAS, is primarily localized to the proximal tubular epithelium. Although its biologic activity is incompletely understood, its main mode of action is thought to be mediated by its ability to serve as a precursor to angiotensin II by the site-specific and possibly species-specific actions of ACE and chymase. Other pathways may, however, also contribute to the overall effects of angiotensin-(1-12), which in the rat kidney may also include the formation of angiotensin-(1-7) and angiotensin-(1-4) by neprilysin.
Angiotensin A and Alamandine
Identified by mass spectroscopy, angiotensin A and alamandine are characterized by the decarboxylation of N-terminal aspartic acid to alanine in angiotensin II and angiotensin-(1-7), respectively. Although the extremely low concentrations of angiotensin A suggest that it is unlikely to play a physiologic role, this may not be the case for alamandine, which circulates in human plasma and at increased concentrations in patients with end-stage kidney disease (ESKD). With the ability of alamandine to lower blood pressure and reduce fibrosis, the actions of alamandine resemble those of angiotensin-(1-7). However, rather than exerting its effects via the Mas receptor, the actions of alamandine occur through a related receptor, the Mas-related G protein–coupled receptor (MrgD).
Intrarenal Renin-Angiotensin-Aldosterone System
In the traditional view of the RAAS, angiotensin II functions as a hormone that, in classical endocrine fashion, circulates systemically to act at sites distant from those where it was formed. However, since the cloning of its components, it has become increasingly clear that there is an additional local, tissue-based RAAS that functions quasi-independently from its systemic counterpart, acting in paracrine, autocrine, and possibly even intracrine modes. This is most clearly seen in the kidney, where pioneering work of several groups has shown that not only does the kidney possess all the necessary molecular machinery to synthesize angiotensin II and other bioactive angiotensin peptides but that their concentrations in glomerular filtrate, tubule fluid, and the interstitium are frequently between 10- and 1000-fold higher than in plasma.
Within the kidney, renin-expressing cells have traditionally been considered to be terminally differentiated and confined to the JGA. However, in a series of elegant studies using a fate-mapping Cre-loxP system, Sequeira Lopez and coworkers showed that renin-expressing cells are precursors to a range of other cell types in the kidney, including those of the arteriolar media, mesangium, Bowman’s capsule, and proximal tubule. Although normally quiescent, these cells may undergo metaplastic transformation to synthesize renin when homeostasis is challenged. Such threats include not only those related to volume depletion but also tissue injury. For instance, in the setting of single-nephron hyperfiltration and consequent progressive dysfunction that follows renal mass reduction, Gilbert and colleagues noted the de novo expression of renin mRNA and angiotensin II peptide in tubular epithelial cells.
In addition to resident kidney cells, infiltrating mast cells may contribute to activation of the local RAAS in disease. Traditionally associated with allergic reactions and host responses to parasite infestation, mast cells have been increasingly recognized for their role in inflammation, immunomodulation, and chronic disease. In the kidney, interstitial mast cell infiltration accompanies most forms of chronic kidney disease (CKD), in which their abundance correlates with the extent of tubulointerstitial fibrosis and declining GFR, though not proteinuria. Mast cells have been shown to synthesize renin, such that their degranulation will release large quantities of both renin and chymase, accelerating angiotensin II formation in the local environment.
Intracrine Renin-Angiotensin-Aldosterone System
Peptide hormones traditionally bind to their cognate receptors on the plasma membrane and produce their effects through the generation of secondary intermediates. However, emerging evidence suggests that certain peptides may also act directly within the cell’s interior, having arrived there by either internalization or intracellular synthesis. For instance, angiotensin II has not only been localized within the cytoplasm and nucleus, but its introduction into the cytoplasm was shown more than a decade ago to have major effects on intracellular calcium currents. Uptake of angiotensin II from the extracellular space likely contributes to its intracellular activity; however, later studies have focused predominantly on its endogenous synthesis. Consistent with the potential role for intracellular angiotensin II, transgenic mice that express an enhanced cyan fluorescent protein–angiotensin II fusion protein that lacks a secretory signal, so that it is retained intracellularly, develop hypertension with microthrombi in glomerular capillaries and small vessels. To date, numerous canonical and noncanonical pathways in the cytoplasm, nucleus, and mitochondria have been implicated in the intracrine RAAS, providing a new forefront for the role of the RAAS in physiology, pathophysiology, and therapeutics.
Renin-Angiotensin-Aldosterone System in Kidney Pathophysiology
In a critical series of experiments in the 1980s, Brenner, Hostetter, and colleagues studied the hemodynamic effects of renal mass ablation in 5/6 nephrectomized rats, a well-established model of progressive kidney disease. In the setting of nephron loss, those glomeruli that remain undergo compensatory enlargement with increased single nephron GFR (SNGFR) and elevations in P GC , ultimately leading to glomerulosclerosis and loss of function. That this phenomenon might be related to angiotensin II was suggested by previous work in which angiotensin II infusion was demonstrated to also result in elevated P GC . Together these studies suggested that intraglomerular hypertension, as a consequence of the action of angiotensin II, was a pivotal factor underlying the inexorable progression of kidney disease and that strategies that reduce P GC should lead to its amelioration. Indeed, in proof-of-concept studies, blockade of angiotensin II formation with the ACE inhibitor enalapril was shown to dilate the glomerular efferent arteriole and to reduce P GC and disease progression in 5/6 nephrectomized rats. In contrast, combination therapy with hydralazine, reserpine, and hydrochlorothiazide, though equally effective in lowering systemic blood pressure, failed to ameliorate intraglomerular hypertension and disease progression. These studies were soon followed by similar ones in other disease models, particularly diabetes, which, like the 5/6 nephrectomized rat, is also characterized by increased SNGFR and elevated P GC .
Fibrosis
During the past 20 years considerable research has focused on many of the nonhemodynamic effects of angiotensin II. For instance, in addition to their effects on P GC , ACE inhibitors and ARBs are also highly effective in reducing interstitial fibrosis and tubular atrophy, which are close correlates of progressive kidney dysfunction. Underlying these effects is the ability of angiotensin II to potently induce expression of the profibrotic and proapoptotic growth factor TGF-β in a range of kidney cell types. Consistent with these in vitro studies, TGF-β overexpression is seen in both the glomerular and tubulointerstitial compartments in 5/6 nephrectomized and diabetic rats, in which studies also showed that both ACE inhibitors and ARBs were effective at reducing TGF-β and disease progression. Similarly, in human diabetic nephropathy, the ACE inhibitor perindopril was found to reduce TGF-β mRNA in a sequential renal biopsy study, and losartan was shown to lower urinary TGF-β excretion.
Proteinuria
The development of proteinuria is both a cardinal manifestation of glomerular injury and a pathogenetic factor in the progression of renal dysfunction. Although P GC remains an important factor in determining the transglomerular passage of albumin, later work has focused on the potential contribution of the podocyte. Indeed, podocyte injury is a cardinal manifestation of proteinuric renal disease, in which foot process effacement has been shown to be prevented by both ACE inhibition and angiotensin receptor blockade. Other studies have focused on nephrin, a podocyte slit pore membrane protein, because of its crucial role in the development and function of the glomerular filtration barrier. Of note, podocytes express the AT 1 receptor and respond to the addition of angiotensin II to the cell culture medium by dramatically decreasing their expression of nephrin. Consistent with these findings, the reduction in nephrin expression in patients with diabetic nephropathy was shown to be ameliorated by ACE inhibitor treatment for 2 years.
Inflammation, Immunity, and the Renin–Angiotensin-Aldosterone System
Inflammatory cell infiltration is a long-recognized feature of CKD that is attenuated in rodent models by agents that block the RAAS. In the in vitro setting, angiotensin II activates NF-κB by both AT 1 receptor– and AT 2 receptor–dependent pathways, stimulating the expression of a number of potent chemokines such as MCP-1 and RANTES (regulated on activation, normal T expressed, and secreted), as well as cytokines, such as IL-6. In addition to angiotensin II, angiotensin-(1-7), acting via the Mas receptor, activates NF-κB, inducing proinflammatory effects in the kidney under both basal and disease settings.
In addition to macrophages, mast cells, and other components of the innate immune system, the adaptive immune system also appears to be involved in the pathogenesis of angiotensin II–mediated organ injury. Of note, suppression of the adaptive immune system prevents the development of angiotensin II–dependent hypertension in experimental models, and adoptive transfer of CD4 + CD25 + regulatory T cells is able to ameliorate angiotensin II–dependent injury.
Diabetes Paradox
Despite the fact that patients with long-standing diabetes characteristically have low plasma renin levels, suggesting that the RAAS is not activated by the disease, agents that block the RAAS are the mainstay of therapy in diabetic nephropathy. Compounding this apparent paradox, whereas PRA is normal or low in diabetes, plasma prorenin levels are characteristically elevated. This dichotomy suggests differences in cell-specific responses to diabetes, because the JGA is the primary source of renin secretion, whereas prorenin is secreted by a much wider range of cell types. In a recent commentary, Peti-Peterdi and associates have ventured to explain the (pro)renin paradox of diabetes. Whereas early diabetes would lead to augmented succinate levels and enhanced JGA renin release, elevated angiotensin II levels would thereafter suppress JGA renin secretion. In contrast to this negative feedback at the JGA, angiotensin II has been shown to have the opposite effect in the tubule, with diabetes causing a 3.5-fold increase in collecting duct renin that could be reduced by AT 1 receptor blockade.
Endothelin
ETs are potent vasoconstrictors that, although expressed primarily in the vascular endothelium, are also notably present within the renal medulla. The biologic effects of the ET system are mediated by two receptors, endothelin types A (ET-A) and B (ET-B). In the kidneys these receptors contribute to the regulation of renal blood flow, salt and water balance, and acid-base homeostasis, as well as potentially mediating tissue inflammation and fibrosis. An important therapeutic role has emerged for ET receptor antagonism in the treatment of pulmonary hypertension ; ET receptor antagonists have been granted regulatory authority approval for this indication in the United States and in Europe. ET receptor blockade as a therapeutic strategy has been investigated in a range of renal diseases. Clinical trials have demonstrated the antiproteinuric and antihypertensive properties of ET receptor antagonists. However, adverse side effects, most notably fluid retention and hepatotoxicity, and issues related to trial design have impeded the development of ET receptor blockers for these indications.
Structure, Synthesis, and Secretion of the Endothelins
ETs consist of three 21–amino acid isoforms that are structurally and pharmacologically distinct: ET-1, ET-2, and ET-3. The dominant isoform in the cardiovascular system is ET-1. Differences in the amino acid sequence among the isopeptides are minor. All three isoforms share a common structure with a typical hairpin-loop configuration resulting from two disulfide bonds at the amino-terminal and a hydrophobic carboxy-terminal containing an aromatic indol side chain at Trp 21 ( Figure 13.5 ). Both the carboxy-terminal and the two disulfide bonds are responsible for the biologic activity of the peptide.

ETs are synthesized from preprohormones by posttranslational proteolytic cleavage mediated by furin and other enzymes. Dibasic pair–specific processing endopeptidases, which recognize Arg-Arg or Lys-Arg paired amino acids, cleave preproETs, reducing their size from approximately 203 to 39 amino acids. Subsequent proteolytic cleavage of the largely biologically inactive big ETs is mediated by endothelin-converting enzymes (ECEs), the key enzymes in the ET biosynthetic pathway. ECEs are type II membrane-bound metalloproteases whose amino acid sequence is significantly homologous to that of NEP 24.11.
Secretion of ET-1 is dependent on de novo protein synthesis, which is constitutive. However, a range of stimuli may also increase ET synthesis through both transcriptional and posttranscriptional regulation ( Table 13.1 ). Once it is synthesized, ET-1 is secreted by endothelial cells into the basolateral compartment, toward the adjacent smooth muscle cells. Because of its abluminal secretion, plasma levels of ET-1 do not necessarily reflect its production.
Stimulation | |
Vasoactive peptides | Growth factors |
Angiotensin II | Epidermal growth factor |
Bradykinin | Insulin-like growth factor |
Vasopressin | TGF-β |
Endothelin 1 | Coagulation |
Epinephrine | Thromboxane A 2 |
Insulin | Tissue plasminogen activator |
Glucocorticoids | Other |
Prolactin | Calcium |
Inflammatory mediators | Hypoxia |
Endotoxin | Shear stress |
Interleukin-1 | Phorbol esters |
TNF-α | Oxidized low-density lipoproteins |
Interferon-β | |
Inhibition | |
ANP | Prostacyclin |
BNP | Protein kinase A activators |
Bradykinin | Nitric oxide |
Heparin | ACE inhibitors |
Within the kidneys, ET-1 expression is most abundant in the inner medulla. In fact, this region possesses the highest concentration of ET-1 of any tissue bed. In addition to the inner medullary collecting ducts (IMCDs), ETs have also been described in glomerular endothelial cells, glomerular epithelial cells, mesangial cells, vasa recta, and tubular epithelial cells. The kidney also synthesizes ET-2 and ET-3, although at much lower levels than ET-1. As with ET-1, ECE mRNA is also more abundant in the renal medulla than in the cortex under normal conditions. However, in disease states such as chronic heart failure, there is upregulation of ECE mRNA primarily within the cortex. In human kidneys, ECE1 has been localized to endothelial and tubular epithelial cells in the cortex and medulla.
Endothelin Receptors
ETs bind to two seven-transmembrane domain G protein–coupled receptors, ET-A and ET-B. Within the vasculature, ET-A receptors are found on smooth muscle cells, where they mediate vasoconstriction. Although ET-B receptors localized on vascular smooth muscle cells can also mediate vasoconstriction, they are also expressed on endothelial cells, where their activation results in vasodilation through the production of nitric oxide and prostacyclin. In addition to their role in mediating vascular tone, ET-B receptors also act as clearance receptors for ET-1, particularly in the lung, where ET-B receptor–binding accounts for approximately 80% of clearance. In the kidney the ET-B receptor has predominantly renoprotective effects, including natriuresis and vasodilation.
Expression of both ET-A and ET-B receptors in the kidney is most prominent within the IMCDs, although binding of ET-1 also occurs in smooth muscle cells, endothelial cells, renomedullary interstitial cells, thin descending limbs, and medullary thick ascending limbs. ET-A receptors are localized to several renovascular structures, including vascular smooth muscle cells, arcuate arteries, and pericytes of descending vasa recta, as well as glomeruli. ET-B receptors, although prominently represented within the medullary collecting system, have also been demonstrated in proximal convoluted tubules, collecting ducts of the inner cortex, medullary thick ascending limbs, and podocytes.
Physiologic Actions of Endothelin in the Kidney
The ETs have several effects on normal renal function, including regulation of renal blood flow, sodium and water balance, and acid-base homeostasis. Although ET-1 has hemodynamic effects in almost all vessels, sensitivity varies between different vascular beds. The renal vasculature, along with the mesenteric vessels, is the most sensitive, with vasoconstriction occurring at picomolar concentrations of ET-1, increasing renal vascular resistance and decreasing renal blood flow. However, long-lasting vasoconstriction, which is mediated by the ET-A receptor, may be preceded by a transient ET-B receptor–mediated vasodilation. Because of the site-specific distribution of ET receptors, ET-1 may exert different vasoconstrictive and vasodilatory effects in different regions of the kidneys. For example, by inducing nitric oxide release from adjacent tubular epithelial cells, ET-1 may actually increase blood flow in the renal medulla, where ET-B receptors predominate.
In addition to effects on renal blood flow, the ET system also plays a direct role in renal sodium and water handling. In the renal medulla, ET is regulated by sodium intake and exerts its natriuretic and diuretic effects through the ET-B receptor. In addition to natriuretic and diuretic effects, the ET-B receptor may also contribute to acid-base homeostasis by stimulating proximal tubule Na + -H + -exchanger isoform 3. Although the role of ET-B receptor activation in urinary sodium excretion has been appreciated for some time, later evidence suggests that renal medullary ET-A receptors may also mediate natriuresis. This may partly explain the edema that can occur as a side effect of ET-A or dual ET receptor antagonism.
Role of Endothelin in Essential Hypertension
Given its potent vasoconstrictive properties, it is unsurprising that ET-1 has been implicated in the pathogenesis of hypertension. In preclinical models of hypertension, ET antagonism may ameliorate heart failure, vascular injury, and renal failure, as well as decreasing stroke. ET-A receptor antagonism has also been shown to normalize blood pressure in rats exposed to eucapnic intermittent hypoxia, analogous to sleep apnea in humans.
PreproET-1 mRNA is increased in the endothelium of subcutaneous resistance arteries in patients with moderate to severe hypertension. However, plasma ET-1 levels are not universally elevated, with an increase more commonly in the presence of end-organ damage or in salt-depleted, salt-sensitive patients with a blunted renin response. A major component of this increase in disease is often decreased clearance by the kidney. These findings suggest that certain patient subgroups may be more responsive to ET receptor blockade than others. Females appear to be relatively protected from the pressor effects of ET-1 by virtue of both increased ET-B expression and a blunted hemodynamic response to ET-A activation.
Clinical trials of the antihypertensive effects of ET receptor antagonism have been hampered by difficulties with selectivity for the ET-A receptor, study design, dosing regimens, and adverse events. Because the ET-B receptor exerts diuretic and natriuretic effects, induces vasodilation, and clears ET-1, selective ET-A receptor antagonists may be expected to demonstrate a more favorable antihypertensive profile. Mixed ET (ET-A and ET-B) and specific ET-A receptor antagonists are distinguished by their in vitro binding affinities. Mixed ET (ET-A and ET-B) antagonists demonstrate selectivity for ET-A of less than 100-fold, and ET-A selective antagonists have an affinity for the ET-A receptor of 100-fold or greater. However, it has been suggested that an affinity of 1000-fold or higher may be required to induce ET-A receptor–specific effects in vivo. In an early study, treatment of patients with essential hypertension with the nonselective ET receptor antagonist bosentan decreased blood pressure as effectively as enalapril, without reflex neurohumoral activation, over a 4-week period. Similarly, in 115 patients with resistant hypertension on three or more agents, the selective ET-A receptor antagonist darusentan significantly reduced blood pressure at 10 weeks. In a subsequent study of 379 individuals with resistant hypertension, darusentan treatment for 14 weeks reduced blood pressure by approximately 18/10 mm Hg with no evidence of dose dependence across a range of 50 to 100 mg/day. However, in a second study of similar design, a large placebo effect meant that darusentan treatment failed to achieve its primary end point of change in office blood pressure, and the development of the drug for this indication was halted. Interestingly, in both of these studies ambulatory blood pressure monitoring revealed a reduction in systolic blood pressure with active treatment. However, also in both studies, peripheral edema or fluid retention was more common in patients treated with darusentan than in those receiving placebo.
Role of Endothelin in Renal Injury
Increasing evidence indicates that, beyond its effects on vascular tone, the ET system is also directly involved in the pathogenesis of fibrotic injury in CKD. In patients with CKD, plasma ET-1 concentrations are elevated, due to both increased production and decreased renal clearance, and urinary levels of ET-1 are also increased, indicative of increased renal ET-1 expression. However, as seen in the studies of hypertension, an unfavorable side-effect profile and possibly also dosing issues related to study design have impeded the development of ET receptor antagonists for the treatment of CKD.
One mechanism for increased renal ET-1 in CKD is a direct effect of urinary protein on tubular epithelial ET-1 expression. Beyond direct effects of urine protein, a number of proinflammatory factors induce ET-1 expression in the kidney, including hypoxia, angiotensin II, thrombin, thromboxane A 2 , TGF-β, and shear stress (see Table 13.1 ).
Several distinct mechanisms may account for the injurious effects of ET-1 on the kidney. Locally derived ET-1 has direct hemodynamic effects, increasing P GC at high doses and causing vasoconstriction of the vasa recta and peritubular capillaries, with a resultant reduction in tissue oxygen tension. ET-1 also acts as a chemoattractant for inflammatory cells, which may express the peptide themselves, stimulating interstitial fibroblast and mesangial cell proliferation and mediating the production of a number of factors associated with collagenous matrix deposition, including TGF-β, matrix metalloproteinase 1, and tissue inhibitors of metalloproteinases 1 and 2. Finally, ET-1 can induce cytoskeletal remodeling in both mesangial cells and podocytes. ET-1 induces mesangial cell contraction and may promote transformation from a quiescent to an activated state. In podocytes, increased passage of protein across the filtration barrier causes cytoskeletal rearrangements and coincident upregulation of ET-1, which may act in an autocrine manner to further propagate ultrastructural injury in the same cells.
Endothelin System in Chronic Kidney Disease and Diabetic Nephropathy
ET receptor antagonists have been employed to study the role of ETs in renal pathophysiology in a range of experimental models, including the rat remnant kidney, lupus nephritis, and diabetes. In the remnant kidney model of progressive renal disease, although beneficial effects have been reported with nonselective ET receptor antagonists, selective ET-A receptor inhibition appears to be superior, with concomitant inhibition of ET-B receptors potentially abrogating any beneficial effects.
Evidence for an effect of glucose itself on ET synthesis and secretion is conflicting. Mesangial cell p38 MAP kinase activation in response to ET-1, angiotensin II, and PDGF is enhanced in the presence of high glucose levels. In contrast, mesangial contraction in response to ET-1 is diminished under high-glucose conditions. Circulating ET-1 concentrations are elevated in animal models of both type 1 and type 2 diabetes, although receptor levels are usually not affected. In experimental diabetic nephropathy, increased expression of ET-1 and its receptors has been found in glomeruli and in tubular epithelial cells, although increased expression of ET receptors has not been a universal finding. Diabetes also causes an increase in renal ECE1 expression, the effect being synergistic with that of radiocontrast media.
A number of studies have investigated the effect of both nonselective and selective ET-A receptor inhibitors in experimental diabetic nephropathy. In streptozotocin-diabetic rats, the nonselective ET receptor antagonist bosentan has provided conflicting results, whereas another nonselective ET receptor inhibitor, PD142893, improved renal function when administered to streptozotocin-diabetic rats that were already proteinuric. In Otsuka Long Evans Tokushima Fatty (OLETF) rats, a model of type 2 diabetes, selective ET-A receptor blockade attenuated albuminuria, without affecting blood pressure, whereas ET-B receptor inhibition had no effect. In a study of streptozotocin-diabetic apolipoprotein E–knockout mice, the renoprotective effects of the predominant ET-A receptor antagonist avosentan were comparable or superior to the ACE inhibitor quinapril. Diabetic ET-B receptor–deficient rats develop severe hypertension and progressive renal failure, supporting a protective role for the ET-B receptor in diabetic nephropathy.
Reactive oxygen species accumulation plays a major role in the pathogenesis of diabetic complications and diabetic nephropathy in particular, and several observations suggest that the ET system may contribute to oxidative stress. In low-renin hypertension, ET-1 increases superoxide in carotid arteries, and ET-A receptor blockade decreases vascular superoxide generation. Similarly, ET-1 infusion increases urinary 8-isoprostane prostaglandin F 2α excretion in rats, indicative of increased generation of reactive oxygen species. In contrast, however, other preclinical studies have suggested a predominantly proinflammatory role for ET-1 in diabetic nephropathy. For instance, the selective ET-A receptor antagonist ABT-627 prevented the development of albuminuria in streptozotocin-diabetic rats without an improvement in markers of oxidative stress but with a reduction in macrophage infiltration and urinary excretion of TGF-β and prostaglandin E 2 metabolites.
Both plasma and urinary ET-1 levels are increased in patients with CKD, with plasma ET-1 levels correlating inversely with estimated GFR. In a study of hypertensive patients with CKD, both selective ET-A receptor blockade and nonselective ET receptor inhibition lowered blood pressure. However, whereas ET-A receptor blockade increased both renal blood flow and effective filtration fraction and decreased renal vascular resistance, dual blockade had no effect. These observations suggest that, although activation of the ET-B receptor does contribute to systemic vascular tone, its role in mediating renal vasodilation likely predominates.
In a study of 22 persons with CKD who did not have diabetes, intravenous infusion of the ET-A receptor antagonist BQ-123 reduced pulse wave velocity and proteinuria to a greater extent than the calcium channel antagonist nifedipine, which comparably lowered blood pressure. These findings suggest a mechanism of action for the antiproteinuric effect observed that is potentially independent from blood pressure. In a subsequent study by the same investigators, 27 subjects with proteinuric CKD were treated with the ET-A receptor antagonist sitaxsentan for 6 weeks in a three-way crossover study design. Sitaxsentan treatment was associated with a reduction in blood pressure, proteinuria, and pulse wave velocity, whereas nifedipine reduced pulse wave velocity and blood pressure but had no effect on urine protein excretion. A fall in GFR with sitaxsentan therapy observed in this study is analogous to that seen with RAAS blockade. Although no clinically significant adverse effects were seen, sitaxsentan development has subsequently been halted due to hepatotoxicity.
The effect of the ET-A receptor antagonist avosentan was examined in a placebo-controlled trial of 286 patients with diabetic nephropathy and macroalbuminuria in addition to standard treatment with an ACE inhibitor or angiotensin II receptor blocker. At 12 weeks, avosentan was found to decrease urine albumin excretion rate without affecting blood pressure. However, a subsequent large phase III study examining the effects of avosentan, on top of RAAS blockade, in 1392 persons with type 2 diabetes and nephropathy was terminated after a median duration of 4 months due to adverse events. In that study, despite a reduction of greater than 40% in albumin to creatinine ratio with avosentan, adverse events, predominantly fluid overload and congestive heart failure, occurred more frequently in those receiving active therapy than in those receiving placebo. Given the relatively high dose of avosentan used in the study, it remains to be determined whether lower doses of avosentan would attenuate albuminuria without the increase in fluid overload.
Endothelin System and Other Kidney Diseases
In addition to diabetic and nondiabetic CKD, the role of the ET system has also been investigated in a number of other experimental models, including acute renal ischemia, cyclosporine-induced nephrotoxicity, and renal allograft rejection. Overall, these studies have suggested some degree of renoprotection with either selective ET-A or nonselective ET receptor inhibitors.
Endotoxemia
ET-1 may play a role in sepsis-mediated acute kidney injury, although again experimental findings have been conflicting, dependent to some extent on the ET receptor antagonist employed. For example, in a rat model of early normotensive endotoxemia, neither an ET-A receptor antagonist nor combined ET-A and ET-B receptor blockade improved GFR, whereas ET-B receptor blockade alone resulted in a marked reduction in renal blood flow. In contrast, in a porcine model of endotoxemic shock, the dual ET receptor antagonist tezosentan attenuated the decrease in renal blood flow and increase in plasma creatinine level.
Systemic Lupus Erythematosus
Urinary ET-1 excretion is correlated with disease activity in patients with systemic lupus erythematosus (SLE), and serum from patients has been shown to stimulate ET-1 release from endothelial cells in culture. In accordance with a pathogenetic role for the ET system in SLE, the ET-A receptor antagonist FR139317 attenuated renal injury in a murine model of lupus nephritis.
Hepatorenal Syndrome
Plasma ET-1 concentrations are increased in individuals with cirrhosis and ascites and in patients with type 2 hepatorenal syndrome (diuretic-resistant or refractory ascites with slowly progressive renal decline), in which there is systemic vasodilation with paradoxical renal vasoconstriction. To investigate the therapeutic potential of ET receptor antagonism in this setting, the combined ET-A and ET-B receptor blocker tezosentan was administered to six patients in an early-phase clinical trial. In this study, treatment was discontinued early in five patients, one because of systemic hypotension and four because of concerns about worsening renal function. These adverse effects are consistent with a dose-dependent decline in renal function in patients with acute heart failure treated with tezosentan, and they highlight the need for caution with the use of ET receptor antagonists in certain patient populations.
Preeclampsia
A role for ET-1 in the development of preeclampsia is suggested by the observations that infusion of fms-like tyrosine kinase 1 and TNF-α into pregnant rats induced ET-A–dependent hypertension, whereas ET-A receptor antagonism attenuated placental ischemia-induced hypertension in a rat model. Despite the mechanistic role of ET-1 in the pathogenesis of preeclampsia, however, ET receptor antagonists are very unlikely to be used in this condition given their known teratogenicity.
Safety Profile of Endothelin Receptor Antagonists
The therapeutic development of ET receptor antagonists has been impeded by the adverse side-effect profile of currently available agents. Most notable has been the development of fluid retention, peripheral edema, and congestive heart failure despite the use of predominant ET-A receptor antagonists. The mechanisms that underlie the fluid retention associated with ET-A receptor antagonism are not fully resolved. It has been suggested that the use of comparatively high doses of ET-A receptor antagonists may have resulted in concurrent ET-B receptor blockade. However, inhibition of nephron ET-A receptors may also be implicated. Hepatotoxicity may be a class effect or may be restricted to particular subclasses of ET receptor antagonist. For instance, a rise in hepatic transaminase levels has been observed with both bosentan and sitaxsentan, which are both sulfonamide-based agents, but not with ambrisentan or darusentan, which are propionic acid–based. Finally, as discussed earlier, teratogenicity would preclude the use of this class of agents in pregnancy.
Natriuretic Peptides
The natriuretic peptides (NPs) are a family of vasoactive hormones that play a role in salt and water homeostasis. The family consists of at least five structurally related but genetically distinct peptides, including atrial natriuretic peptide (ANP), brain natriuretic peptide (BNP), C-type natriuretic peptide (CNP), Dendroaspis natriuretic peptide (DNP), and urodilatin. ANP was originally isolated from human and rat atrial tissues in 1984. Since then, the NP family has been expanded to include several other members, all of which share a common 17–amino acid ring structure that is stabilized by a cysteine bridge and that contains several invariant amino acids. BNP and CNP were both originally identified in porcine brain, whereas DNP was first isolated from the venom of the green mamba snake, Dendroaspis angusticeps. Urodilatin is an NH 2 -terminally extended form of ANP that was initially described in human urine. NP inactivation occurs through at least two distinct pathways, binding to a clearance receptor (natriuretic peptide receptor [NPR]–C) and enzymatic degradation. Other peptides that may be involved in salt and water balance include guanylin, uroguanylin, and adrenomedullin.
ANP and BNP act as endogenous antagonists of the RAAS mediating natriuresis, diuresis, vasodilation, and suppression of sympathetic activity, as well as inhibiting cell growth and decreasing secretion of aldosterone and renin. The role of NPs in cardiovascular and renal disease, particularly BNP, has led to their adoption into clinical practice as indicators of disease states and, to some extent, as therapeutic agents.
Structure and Synthesis of the Natriuretic Peptides
Atrial Natriuretic Peptide
ANP is a 28–amino acid peptide comprising a 17–amino acid ring linked by a disulfide bond between two cysteine residues and a COOH-terminal extension that confers its biologic activity ( Figure 13.6 ). The gene for ANP, natriuretic peptide precursor type A (NPPA), is found on chromosome 1p36 and encodes the precursor preproANP, which is between 149 and 153 amino acids in length according to the species of origin. Human preproANP consists of 151 amino acids and is rapidly processed to the 126–amino acid proANP. ANP is identical in mammalian species except for a single amino acid substitution at residue 110, which is isoleucine in the rat, rabbit, and mouse, and methionine in the human, pig, dog, sheep, and cow.

ANP synthesis occurs primarily within atrial cardiomyocytes, where it is stored as proANP, the main constituent of the atrial secretory granules. The major stimulus to ANP release is mechanical stretch of the atria secondary to increased wall tension. However, ANP synthesis and release may also be stimulated by neurohumoral factors such as glucocorticoids, ET, vasopressin, and angiotensin II, partly through changes in atrial pressure and partly through direct cellular effects. Although ANP mRNA levels are approximately thirty to fiftyfold higher in the cardiac atria than in the ventricles, ventricular expression is dramatically increased in the developing heart and in conditions of hemodynamic overload such as heart failure and hypertension. Beyond the heart, the peptide has also been demonstrated in the kidney, brain, lung, adrenal gland, and liver. In the kidney, alternate processing of proANP adds four amino acids to the NH 2 terminus of the ANP peptide to generate a 32–amino acid peptide, proANP 95-126, or urodilatin.
ANP is stored, primarily as proANP, in the secretory granules of the atrial cardiomyocytes and is released by fusion of the granules with the cell surface. During this process proANP is cleaved to an NH 2 -terminal 98–amino acid peptide (ANP 1-98) and the COOH-terminal 28–amino acid biologically active fragment (ANP 99-126). Both fragments circulate in the plasma, with further processing of the NH 2 -terminal fragment leading to the generation of peptides ANP 1-30 (long-acting NP), ANP 31-67 (vessel dilator), and ANP 79-98 (kaliuretic peptide), all of whose biologic actions may be similar to those of ANP.
Brain Natriuretic Peptide
The BNP gene is located approximately only 8 kb upstream of the ANP gene on the short arm of chromosome 1 in humans, which suggests that the two genes may share in common both evolutionary origin and transcriptional regulation. In contrast, CNP is found separately on chromosome 2. CNP is highly conserved across species, indicating that the peptide may represent the evolutionary ancestor of ANP and BNP.
BNP, like ANP, is synthesized as a preprohormone, between 121 and 134 amino acids in length, according to species of origin. Human preproBNP (134 amino acids) is cleaved to produce the 108–amino acid precursor proBNP. Further processing leads to the production of the 32–amino acid, biologically active BNP, which corresponds to the C terminus of the precursor, as well as a 76–amino acid N-terminal fragment (N-terminal proBNP [NT-proBNP]). Active BNP, NT-proBNP, and proBNP all circulate in the plasma. Circulating BNP contains the characteristic 17–amino acid ring structure closed by a disulfide bond between two cysteine residues, along with a 9–amino acid amino-terminal tail and a 6–amino acid carboxy tail (see Figure 13.6 ).
The term brain natriuretic peptide is somewhat misleading because the primary sites of synthesis of BNP are the cardiac ventricles, with expression also occurring to a lesser extent in atrial cardiomyocytes. Like ANP, expression of BNP is regulated by changes in intracardiac pressure and stretch. However, unlike ANP, which is stored and released from secretory granules, BNP is regulated at the gene expression level and is synthesized and secreted in bursts. BNP expression is increased in heart failure, hypertension, and renal failure. Its plasma half-life is approximately 20 minutes, in contrast to a circulating half-life of ANP of 2 to 5 minutes and a half-life of the biologically inactive NT-proBNP of 120 minutes. This difference is relevant to the utility of NP measurement as a biologic marker of cardiorenal disease. Changes in pulmonary capillary wedge pressure may be reflected by plasma BNP concentrations every 2 hours and NT-proBNP levels every 12 hours. The physiologic actions of BNP are similar to those of ANP, including effects on the kidney (natriuresis and diuresis), vasculature (hypotension), endocrine systems (inhibition of plasma renin and aldosterone secretion), and brain (central vasodepressor activity).
C-Type Natriuretic Peptide
As is the case for ANP and BNP, CNP is derived from a prepropeptide that undergoes posttranslational proteolytic cleavage. The initial translation product preproCNP is 126 amino acids in length and is cleaved to produce the 103–amino acid prohormone. Cleavage of proCNP yields two mature peptides made up of 22 and 53 amino acids, referred to as CNP and NH 2 -terminally extended form of CNP, respectively. Eleven of the 17 amino acids within the CNP ring structure are identical to those in the other NPs, although, uniquely, CNP lacks an amino tail at the carboxy-terminal (see Figure 13.6 ).
CNP functions in an autocrine/paracrine manner with effects on vascular tone and muscle cell growth. Accordingly, plasma concentrations of CNP are very low, although they are increased in heart failure and renal failure. CNP is present in the heart, kidney, and endothelium, and its receptor is also expressed in abundance in the hypothalamus and pituitary gland, suggesting that the peptide may also play a role as a neuromodulator or neurotransmitter. Because CNP is present in primarily noncardiac tissues, regulation of its expression is distinct from that of ANP and BNP and is controlled by a number of vasoactive mediators, including insulin, vascular endothelial growth factor, TGF-β, TNF-α, and interleukin-1β.
The principal enzymes responsible for the conversion of proANP, proBNP, and proCNP to their active forms are the serine proteases, corin and furin. Corin converts proANP to ANP, furin converts proCNP to CNP, and both corin and furin cleave proBNP. Corin is highly expressed in the heart and to a lesser extent in the kidney. In response to pressure overload, corin-deficient mice develop hypertension together with cardiac hypertrophy and dysfunction. In the kidney, corin co-localizes with ANP, and decreased urinary corin excretion has been observed in patients with CKD. Studies combining observations made in organ-specific corin-deficient mice together with human correlative experiments have identified a role for impaired uterine corin/ANP function in the pathogenesis of preeclampsia.
Dendroaspis Natriuretic Peptide
The physiologic role of DNP has been controversial ever since its original identification in the venom of the green mamba snake, D. angusticeps, in 1992. DNP is a 38–amino acid peptide that shares the 17–amino acid ring structure common to all NPs but that has unique N- and C-terminal regions (see Figure 13.6 ). Immunoreactivity for DNP has been reported in human plasma and atrial myocardium, and DNP has also been described in rat and rabbit kidney, rat colon, rat aortic vascular smooth muscle cells, and pig ovarian granulosa cells. DNP binds to natriuretic peptide receptor A (NPR-A) and the clearance receptor NPR-C, which may be of particular relevance given the apparent resistance of the peptide to enzymatic degradation. In dogs, either under normal conditions or in a pacing-induced heart failure model, administration of synthetic DNP decreased cardiac filling pressures and increased GFR, natriuresis, and diuresis, as well as lowering blood pressure, suppressing renin release, and increasing plasma and urine cGMP. Despite these propitious findings, several aspects of the biologic role of DNP remain contentious. In particular, the gene for the peptide has not been identified in mammals, nor has it yet been identified in the green mamba. Immunoreactivity experiments can be subject to artifact, and there are no reports of the fractionation of DNP from human samples. These uncertainties have led some authors to question whether DNP is, in fact, expressed at all in humans.
Urodilatin
Urodilatin is a structural homolog of ANP, synthesized in kidney distal tubular cells and differentially processed to a 32–amino acid NH 2 -terminally extended form of ANP, sharing the same 17–amino acid ring structure and COOH-terminal tail. The peptide is not found in the plasma, acting in a paracrine manner within the kidney on receptors in the glomeruli and IMCDs to promote natriuresis and diuresis. Urodilatin is upregulated in diabetic animals and in the remnant kidney and is relatively resistant to enzymatic degradation, which may explain its more potent renal effects.
Natriuretic Peptide Receptors
NPs mediate their biologic effects by binding to three distinct guanylyl cyclase NPRs. The terminology can be somewhat confusing because NPR-A binds ANP and BNP and natriuretic peptide receptor B (NPR-B) binds CNP, whereas NPR-C acts as a clearance receptor for all three peptides.
NPR-A and NPR-B are structurally similar but share only 44% homology in the extracellular ligand-binding segment, likely responsible for the difference in ligand specificity. Both NPR-A and NPR-B have a molecular weight of approximately 120 kDa and consist of a ligand-binding extracellular domain, a single transmembrane segment, an intracellular kinase domain, and an enzymatically active guanylyl cyclase domain. The kinase homology domain (KHD) of NPR-A and NPR-B shares 30% homology with protein kinases but has no kinase activity. Ligand binding of NPR-A and NPR-B prevents the normal inhibitory action exerted by the KHD on the guanylyl cyclase domain, allowing the generation of cGMP, which acts as a second messenger responsible for most of the biologic effects of the NPs.
NPR-C, in contrast to NPR-A and NPR-B, lacks both the KHD and the catalytic guanylyl cyclase domain and therefore does not signal through a second messenger system. Instead, the receptor contains the extracellular ligand-binding segment, a transmembrane domain, and a 37–amino acid cytoplasmic domain containing a G protein–activating sequence. In NPR-C–knockout mice, blood pressure is reduced and the plasma half-life of ANP is increased, supporting the role of NPR-C as a clearance receptor. NPR-C binds all members of the NP family with high affinity and is the most abundantly expressed of the NPRs, present in the kidney, vascular endothelium, smooth muscle cells, and heart, representing approximately 95% of the total receptor population. Preferential binding of NPR-C to ANP over BNP may explain the relatively increased plasma half-life of BNP. NPR-C clears NPs from the circulation through a process of receptor-mediated endocytosis and lysosomal degradation before rapid recycling of the internalized receptor to the cell surface. Although the primary function of NPR-C is as a clearance receptor, ligand binding may exert biologic effects on the cell through G protein–mediated inhibition of cyclic adenosine monophosphate.
The biologic effects of NPs are largely dependent on the distribution of their receptors. NPR-A mRNA is present mainly in the kidney, especially in the IMCD cells, although the receptor is also notably present within the glomeruli, renal vasculature, and proximal tubules. The distribution of NPR-B overlaps with that of NPR-A, with the receptor found in the kidney, vasculature, and brain. However, consistent with the paracrine effects of CNP on vascular tone, mitogenesis, and cell migration, NPR-B is expressed in greater abundance than NPR-A within the vascular endothelium and smooth muscle, whereas expression levels are relatively lower within the kidney.
Neutral Endopeptidase
Receptor-mediated endocytosis probably accounts for approximately 50% of clearance of the NPs from the circulation; catalytic degradation by the enzyme NEP is responsible for the majority of the rest, and only a minor contribution is attributed to direct renal excretion. Receptor clearance probably plays an even smaller role in conditions associated with chronically elevated NP levels, because of increased receptor occupancy and downregulation of NPR-C expression.
NEP is a membrane-bound zinc metalloproteinase, originally termed enkephalinase because of its ability to degrade opioid receptors in the brain. The enzyme has structural and catalytic similarity with other metallopeptidases, including aminopeptidase, ACE, ECE, and carboxypeptidases A, B, and E. ANP, BNP, and CNP are therefore not the only substrates for NEP, with enzymatic activity demonstrated against a number of other vasoactive peptides, including ET-1, angiotensin II, substance P, bradykinin, neurotensin, insulin B chain, calcitonin gene–related peptide, and adrenomedullin. The primary mechanism of action of NEP is to hydrolyze peptide bonds on the NH 2 side of hydrophobic amino acid residues. In the case of ANP, NEP cleaves the Cys -Phe bond to disrupt the ring structure and inactivate the peptide. The Cys-Phe bond of BNP is relatively insensitive to enzymatic cleavage.
NEP has a nearly ubiquitous tissue distribution, with expression demonstrated in the kidney, liver, heart, brain, lungs, gut, and adrenal gland. The metallopeptidase is present not only on the surface of endothelial cells, but also on smooth muscle cells, fibroblasts, and cardiac myocytes, although it is most abundant in the brush border of the proximal tubules of the kidneys, where it rapidly degrades filtered ANP, preventing the peptide from reaching more distal luminal receptors.
Actions of the Natriuretic Peptides
Renal Effects of the Natriuretic Peptides
The natriuretic and diuretic actions of the NPs are consequences of both vasomotor and direct tubular effects. Both ANP and BNP cause an increase in glomerular capillary hydrostatic pressure and a rise in GFR by inducing afferent arteriolar vasodilation and efferent arteriolar vasoconstriction. These contrasting effects of the NPs on the afferent and efferent arterioles differ from the actions of classical vasodilators such as bradykinin. In addition to direct effects on vascular tone, ANP can also increase GFR through cGMP-mediated mesangial cell relaxation.
Plasma levels of ANP that do not increase GFR can induce natriuresis, illustrating the potential for direct tubular effects, which may involve either locally produced NPs acting in a paracrine manner, such as urodilatin, or circulating NPs. A number of mechanisms may be responsible for the natriuresis, including direct effects on sodium transport in tubular epithelial cells and indirect effects through inhibition of renin secretion following increased sodium delivery to the macula densa. NPs also antagonize vasopressin in the cortical collecting ducts. It is likely that similar mechanisms underlie the response to ANP, BNP, and urodilatin. In contrast, CNP has little natriuretic or diuretic effect, which may indicate a requirement for the presence of the carboxy-terminal extension of the peptide for renal effects.
The NPs may have antifibrotic effects within the kidney as evidenced by an increase in renal fibrosis in NPR-A–knockout mice after unilateral ureteral obstruction. In cultured proximal tubular cells, ANP attenuates high glucose–induced activation of TGF-β 1 , Smad, and collagen synthesis, illustrating the potentially antifibrotic properties of the peptide in the context of diabetic nephropathy.
Cardiovascular Effects
All NPs have vasodilatory and hypotensive properties. Heterozygous mutant mice with a disrupted proANP gene display evidence of salt-sensitive hypertension, whereas hypotension is a feature of transgenic mice overexpressing ANP. In a patient population, a variant in the ANP promoter was associated with both lower levels of plasma ANP and increased susceptibility to early development of hypertension. However, a caveat to the hypotensive role of ANP is the observation that infusion of high concentrations of ANP can actually induce a rise in blood pressure, suggesting activation of counterregulatory baroreceptors.
There are two major direct mechanisms by which ANP may lower blood pressure. First, it increases vascular permeability with a shift of fluid from the intravascular to extravascular compartments by capillary hydraulic pressure. Second, ANP increases venous capacitance, promoting natriuresis and lowering preload. In addition, ANP and BNP antagonize the vasoconstrictive effects of the RAAS, ET, and the sympathetic nervous system by decreasing sympathetic peripheral vascular tone, suppressing the release of catecholamines and reducing central sympathetic outflow. By lowering the activation threshold of vagal afferents, ANP prevents the vasoconstriction and tachycardia that normally follow a reduction in preload, leading to a sustained drop in blood pressure. CNP is a more potent vasodilator than either ANP or BNP. In fact, CNP relaxes human subcutaneous resistance arteries, whereas ANP and BNP have no effect.
NPs have a number of other effects on the cardiovascular system distinct from their action on vasomotor tone. For example, NPs play a major role in cardiac remodeling. Mice genetically deficient for ANP exhibit an increase in cardiac mass, whereas heart size is diminished in mice transgenically overexpressing ANP. The antimitogenic and antitrophic effects of NPs, which appear to be cGMP-mediated, have also been demonstrated in a range of cultured cell types, including cultured vascular cells, fibroblasts, and myocytes, and in vivo in response to balloon angioplasty. Further evidence for the role of ANP in mediating cardiac hypertrophy comes from population studies, in which variants in either the ANP promoter (associated with reduced circulating ANP) or in the NPR-A gene have both been associated with left ventricular hypertrophy. BNP has been shown to have antifibrotic properties within the heart. In vitro BNP antagonizes TGF-β–induced fibrosis in cultured cardiac fibroblasts, and in vivo targeted genetic disruption of BNP in mice is associated with an increase in cardiac fibrosis, in the absence of either hypertension or ventricular hypertrophy.
Cardiac CNP is increased in heart failure, where it may play a role in ventricular remodeling. Comparison of plasma CNP levels in samples taken from the aorta and renal vein at the time of diagnostic heart catheterization has demonstrated that CNP is indeed synthesized and secreted by the kidney. Moreover, this effect was found to be blunted in patients with heart failure, potentially contributing to renal sodium retention.
Other Effects of the Natriuretic Peptides
Even though they do not cross the blood-brain barrier, NPs exert important CNS effects that may augment their peripheral actions. ANP, BNP, and particularly CNP are all expressed within the brain. Circulating NPs may also exert central effects through actions at sites that are outside the blood-brain barrier. The NPR-B receptor is expressed throughout the CNS, reflecting the wide distribution of CNP, whereas the NPR-A receptor is expressed in areas adjacent to the third ventricle, which indicates a role of peripherally circulating ANP and BNP, as well as centrally expressed peptides. Complementing their natriuretic and diuretic effects, NPs inhibit both salt appetite and water drinking. ANP also prevents release of vasopressin and possibly adrenocorticotropic hormone from the pituitary, whereas sympathetic tone is increased by the actions of the NPs on the brainstem.
Clinical and experimental evidence suggests that NPs play a role in mediating metabolism. Circulating levels of NPs are decreased in obese individuals and among patients with the metabolic syndrome, correlating inversely with both plasma glucose and fasting insulin levels. In accordance with these epidemiologic observations, infusion of ANP activates hormone-sensitive lipase from fat cells, indicative of lipolysis. In vitro, ANP inhibits preadipocyte proliferation, the lipolytic properties of the peptide being mediated by cGMP phosphorylation.
Knockout mouse studies have revealed that CNP plays a predominant role in the regulation of skeletal growth, regulating cartilage homeostasis and endochondral bone formation. In mice genetically deficient in either CNP or its receptor NPR-B, there is lack of growth of longitudinal bones and vertebrae and a shortened life span as a consequence of respiratory insufficiency secondary to abnormal ossification of the skull and vertebrae. Mutations in the NPR-B gene have also been reported in patients with the autosomal recessive skeletal dysplasia, Maroteaux type acromesomelic dysplasia, whereas obligate carriers of the mutations have heights that are below predicted levels.
Natriuretic Peptides as Biomarkers of Disease
Both ANP and BNP have been studied as clinical biomarkers of heart failure and renal failure. The short half-life of ANP (2 to 5 minutes) restricts its applicability. However, the biologically inactive NH 2 -terminal 98–amino acid peptide ANP 1-98 does not bind to NPR-A or NPR-C and so remains in the circulation longer than ANP. In heart failure, levels of ANP 1-98 closely reflect the degree of renal function. Plasma concentrations of the midregional epitopes of the stable prohormones of both ANP and adrenomedullin predict the progression of renal decline in patients with nondiabetic CKD. The prognostic performance of midregional proANP is not superior to that of NT-proBNP or BNP in patients undergoing hemodialysis, and measurement of ANP or one of its prohormone derivatives is currently not part of routine clinical care. Signal peptides from both ANP and BNP are present in venous blood and rise rapidly following myocardial infarction, which suggests that their detection may aid in the diagnosis of cardiac ischemia. Commercial assays are widely available for measurement of either BNP or the biologically inactive peptide fragment NT-proBNP. Correspondingly, since 2000, measurement of circulating BNP and NT-proBNP levels has been incorporated into several clinical practice guidelines for the management of heart failure. Important differences distinguish BNP and NT-proBNP from each other as clinical biomarkers. NT-proBNP is not removed from the circulation by binding to the clearance receptor NPR-C, and hence its circulating half-life of approximately 2 hours is significantly longer than that of BNP (approximately 20 minutes). In addition, both BNP and NT-proBNP are affected by renal impairment, with the magnitude of the effect being relatively greater for NT-proBNP.
Brain Natriuretic Peptide and N-Terminal Pro–Brain Natriuretic Peptide as Biomarkers of Heart Failure
Measurement of circulating levels of either BNP or NT-proBNP has been demonstrated to be effective in guiding clinical practice in several aspects of the management of heart failure, including diagnosis, screening, prognosis, and monitoring of therapy. The primary role of BNP measurement in the assessment of dyspnea is as a “rule-out” test; a plasma BNP level lower than 100 pg/mL has a negative predictive value for heart failure of 90%. In the ProBNP Investigation of Dyspnea in the Emergency Department (PRIDE) study, an NT-proBNP level lower than 300 pg/mL was optimal in ruling out heart failure, with a negative predictive value of 99%. In interpreting plasma levels of BNP and NT-proBNP a number of other biologic variables should be taken into account. NP levels rise with age and are higher in females, the latter effect possibly secondary to estrogen regulation, because hormone replacement therapy increases BNP levels. Conversely, NP levels fall with increasing obesity.
Role of Brain Natriuretic Peptide and N-Terminal Pro–Brain Natriuretic Peptide as Biomarkers in Renal Disease
The interpretation of NP concentrations in patients with renal disease merits special consideration. NP levels are increased in individuals with impaired renal function. This increase is likely to be the result of multiple factors and not solely the consequence of increased intravascular volume. Other factors that contribute to increased NP levels include decreased NP responsiveness, subclinical ventricular dysfunction, hypertension, left ventricular hypertrophy, subclinical ischemia, myocardial fibrosis, and RAAS activation, as well as decreased filtration and reduced clearance by NPR-C and NEP.
Although, based on observational studies, it has been widely considered that renal clearance plays a greater role in the removal of NT-proBNP from the circulation than BNP, one study has challenged this view. By measuring both NT-proBNP and BNP in the renal arteries and veins of 165 subjects undergoing renal arteriography, investigators found that both NT-proBNP and BNP are equally dependent on renal clearance. However, the NT-proBNP/BNP ratio did increase with declining GFR, which suggests that the two peptides may be differentially cleared at GFRs less than 30 mL/min/1.73 m 2 .
Even though both BNP and NT-proBNP are affected by renal impairment, their clinical utility for the prediction of heart failure persists in CKD patients, in the context of appropriately adjusted reference ranges. For example, in the Breathing Not Properly study, BNP cutpoint values were approximately threefold higher to diagnose heart failure in patients with an estimated GFR below 60 mL/min relative to the conventional cutpoint value of 100 pg/mL. In a cohort of 831 patients with dyspnea and a GFR below 60 mL/min, both BNP and NT-proBNP were effective predictors of heart failure, although NT-proBNP was superior in predicting mortality. In asymptomatic patients with CKD, both BNP and NT-proBNP were equivalent and effective in indicating the presence of left ventricular hypertrophy or coronary artery disease.
In patients with CKD, BNP and NT-proBNP predict progression of renal decline and cardiovascular disease and mortality. In a population of patients with CKD who are not undergoing dialysis, NT-proBNP, but not BNP, was an independent predictor of death, whereas in 994 black patients with hypertensive kidney disease (GFR of 20 to 65 mL/min/1.73 m 2 ), NT-proBNP predicted cardiovascular disease and mortality, particularly among individuals with proteinuria. In pediatric patients with CKD, both BNP and proBNP (but not troponins I and T) were indicative of left ventricular hypertrophy or dysfunction.
BNP and NT-proBNP have been studied extensively in dialysis patients both as prognostic indicators and as markers of volume status. The low molecular weight of BNP (3.5 kDa) and NT-proBNP (8.35 kDa) is such that both may be cleared by high-flux dialysis. Nevertheless, in contrast to ANP, which falls sharply after either hemodialysis or peritoneal dialysis, levels of BNP and NT-proBNP are less affected. The role of NP levels as indicators of volume status in either hemodialysis or peritoneal dialysis recipients is confounded by the common coexistence of left ventricular abnormalities. Both BNP and NT-proBNP levels are predictive of mortality, heart failure, and coronary artery disease in the dialysis population. However, no definite cutpoint values for diagnosing heart failure in patients undergoing dialysis have been defined.
Therapeutic Uses of Natriuretic Peptides
Even though NP levels are increased in heart failure, their biologic effects are blunted. Intravenous administration of recombinant NPs increases their circulating levels several-fold, overcoming this resistance. As such, two recombinant NPs are currently available as therapeutic agents for the treatment of heart failure. Recombinant ANP (carperitide) is available in Japan for the treatment of pulmonary edema. Recombinant BNP (nesiritide) is licensed in several countries, including the United States, for the treatment of acute decompensated heart failure.
Recombinant Atrial Natriuretic Peptide
ANP has a short half-life and a high total body clearance. Its intravenous administration causes a reduction in blood pressure, diuresis, and natriuresis in healthy individuals, although this response is reduced in the setting of acute heart failure. In a 6-year open-label study of 3777 patients with acute heart failure treated with carperitide, 82% were reported to improve clinically. Early experimental studies also suggested a potential benefit of exogenous ANP in acute renal failure; however, results in patients have generally been disappointing. Nevertheless, the peptide may have a limited role in selected patient populations. For example, low-dose carperitide preserved renal function in patients undergoing abdominal aortic aneurysm repair and reduced the incidence of contrast-induced nephropathy in patients after coronary angiography. However, a meta-analysis suggested that recombinant ANP has no effect on mortality in patients with acute kidney injury, although a trend toward a reduction in the need for renal replacement therapy was shown. In a separate meta-analysis of studies conducted in patients undergoing cardiovascular surgery, ANP infusion decreased peak serum creatinine level, incidence of arrhythmia, and need for renal replacement therapy, whereas both ANP and BNP decreased the length of intensive care unit and hospital stay. Among 367 high-risk individuals undergoing coronary artery bypass graft, recombinant ANP decreased the incidence of major adverse cardiovascular and cerebrovascular events and the need for dialysis, immediately and up to 2 years postoperatively, although survival was unaffected. Similarly, among patients with CKD undergoing coronary artery bypass graft, those receiving recombinant ANP experienced a smaller rise in serum creatinine level, fewer cardiac events, and lower requirement for dialysis, although mortality did not differ from those who did not receive ANP.
Recombinant Brain Natriuretic Peptide
Nesiritide is recombinant human BNP, manufactured from Escherichia coli and identical in structure to native human BNP, with a mean terminal half-life in patients with heart failure of 18 minutes. Intravenous administration of nesiritide lowers pulmonary and systemic vascular resistance, decreases right atrial pressure, and increases cardiac output (presumably through effects on ventricular afterload) in a dose-dependent manner. In the kidney, nesiritide increases renal blood flow and GFR through both direct vasodilatory effects and indirect effects on cardiac output and norepinephrine inhibition. Diuresis and natriuresis may also occur, although these are modest and may not be seen at the approved doses. Additional effects of nesiritide may also include inhibition of renin secretion in the kidney and aldosterone production in the heart and adrenal gland.
In response to meta-analysis data suggesting that nesiritide treatment may be associated with a worsening of renal function and an increase in the rate of early death, the Acute Study of Clinical Effectiveness of Nesiritide in Decompensated Heart Failure (ASCEND-HF) trial was initiated. In this study of 7141 patients hospitalized with acute heart failure, nesiritide neither increased nor decreased the rate of death or rehospitalization, rates of worsening renal function were unaffected, and there was a small but nonsignificant improvement in self-reported rates of dyspnea. Based on these results, the investigators concluded that nesiritide cannot be recommended for routine use in the broad population of patients with acute heart failure.
Therapeutic Uses of Other Natriuretic Peptides
The effects of urodilatin (ularitide) have been assessed in both heart failure and acute renal failure. However, the diuretic effect of urodilatin appears to be attenuated in heart failure patients, reflecting a blunted response as observed for ANP and BNP. Similarly, as with ANP and BNP, hypotension appears to be a dose-limiting side effect of ularitide therapy. In the Safety and Efficacy of an Intravenous Placebo-Controlled Randomized Infusion of Ularitide in a Prospective Double-blind Study in Patients with Symptomatic, Decompensated Chronic Heart Failure (SIRIUS II) study, a phase II trial of 221 patients hospitalized for decompensated heart failure, a single 24-hour infusion of ularitide preserved short-term renal function. The NP vessel dilator may offer theoretical advantages for the treatment of acute decompensated heart failure in comparison with current NP-based therapies. In particular, vessel dilator may produce a greater and more sustained natriuresis than ANP or BNP, without a blunted response in heart failure patients, and may also improve renal function in the setting of experimental acute kidney injury. An alternative therapeutic approach is the development of novel chimeric peptides. For example, researchers have synthesized a peptide (CD-NP) that represents fusion of the 22–amino acid peptide CNP together with the 15–amino acid linear C terminus of DNP. In vitro, this peptide activates cGMP and attenuates cardiac fibroblast proliferation. In vivo, CD-NP is both natriuretic and diuretic and increases GFR with less hypotension than BNP.
Neutral Endopeptidase Inhibition
Notwithstanding concerns regarding the safety, efficacy, and cost-effectiveness of recombinant NP therapy, a major limitation is the requirement for systemic administration, which is unsuitable for chronic treatment. Alternative methods to increase the biologic activity of NPs may offer a more feasible approach for chronic therapy. In particular, inhibition of the enzymatic degradation of NPs by NEP has been the focus of drug discovery efforts for a number of years. NEP is a zinc metallopeptidase with catalytic similarity to ACE and with a wide tissue distribution, although abundant at the proximal tubule brush border. The enzyme has activity against a number of substrates, including the vasodilating peptides ANP, BNP, CNP, substance P, bradykinin, and adrenomedullin and the vasoconstrictors angiotensin II and ET-1. Several pharmacologic NEP inhibitors have been investigated (e.g., candoxatril, thiorphan, and phosphoramidon). Although these agents do in general increase plasma levels of the NPs and, under some experimental conditions, induce natriuresis and diuresis with peripheral vasodilation, clinical trials in hypertension and heart failure have generally been disappointing. Specifically, sustained antihypertensive effects have not been demonstrated, and some studies have reported a paradoxical rise in blood pressure. The biologic actions of the NPs are, however, restored in the presence of an inhibited RAAS, and this has led to the development of compounds that simultaneously inhibit both NEP and ACE, termed vasopeptidase inhibitors (VPIs).
Vasopeptidase Inhibitors
The rational design of metallopeptidase inhibitors (e.g., mixanpril [ S21402 ], CGS30440 , aladotril, MDL 100173, sampatrilat, and omapatrilat) is possible because of the similar structural characteristics of the catalytic sites of both ACE and NEP. These VPIs have theoretical advantages over antagonists of either enzyme in isolation, and a number of preclinical studies have demonstrated their efficacy in experimental models of cardiovascular and renal disease. However, phase III clinical studies have not been able to demonstrate superiority of vasopeptidase inhibition over ACE inhibition, and safety concerns exist over an increase in the incidence of angioedema. As a result, development and clinical application of these compounds have been limited.
Although effective against both ACE and NEP, VPIs differ in their relative potencies for each enzyme. For example, the most extensively studied VPI, omapatrilat, has broadly equivalent potency for NEP (affinity constant [K i ] = 8.9 nmol) and for ACE (K i = 6.0 nmol), whereas the VPI gemopatrilat has an efficacy almost 100 times greater for ACE (K i = 3.6 nmol) than for NEP (K i = 305 nmol). The broad substrate specificities of VPIs can also be expected to result in an increase in ET, adrenomedullin, and bradykinin and a reduction in the synthesis of angiotensin-(1-7). Omapatrilat lowers blood pressure and attenuates heart failure in experimental models, associated with dose-dependent increases in ANP and cGMP excretion, diuresis, natriuresis, and decreased plasma renin activity (although a paradoxical increase in plasma renin activity has also been reported). In the remnant kidney model, VPIs have consistently been found to be as effective as or better than ACE inhibitors, with similar benefits reported in diabetic apolipoprotein E–knockout mice.
In contrast to the promising findings in preclinical models, trials of VPIs in human disease have, on the whole, been disappointing. In the Inhibition of Metalloproteinase in a Randomized Exercise and Symptoms Study (IMPRESS) in heart failure of patients with New York heart Association classes II to IV congestive heart failure, there was no difference between omapatrilat and lisinopril in the primary end point of maximum exercise tolerance at 12 weeks. The Omapatrilat Cardiovascular Treatment vs. Enalapril (OCTAVE) trial randomly assigned 25,302 hypertensive patients to receive omapatrilat or enalapril. Omapatrilat treatment was associated with a reduction in systolic blood pressure that was 3.6 mm Hg greater than that associated with enalapril. However, angioedema occurred in 2.17% of patients treated with omapatrilat, compared with 0.68% in the group treated with enalapril. This concerning side effect was even more common among black patients. In the Omapatrilat Versus Enalapril Randomized Trial of Utility in Reducing Events (OVERTURE), there was no difference between enalapril and omapatrilat in the primary end point of combined risk for death or hospitalization for heart failure requiring intravenous treatment, although a post hoc analysis showed a significant 9% reduction in cardiovascular death or hospitalization. In this trial the incidence of angioedema was again increased in the omapatrilat group compared to patients treated with enalapril (0.8% vs. 0.5%). In general, the side effects of VPIs are qualitatively similar to those of ACE inhibitors, including cough (in approximately 10% of patients), dizziness, and postural hypotension. Flushing appears more commonly with VPIs than with ACE inhibitors, which may be a consequence of increased circulating adrenomedullin concentrations.
Combination Angiotensin Receptor Blockers and Neutral Endopeptidase Inhibitors
To circumvent the adverse effects experienced with VPIs, a novel drug class has been developed that combines the action of both an ARB and an NEP inhibitor, termed an angiotensin receptor–neprilysin inhibitor (ARNi). For instance, the compound LCZ696 is a single molecule comprised of molecular moieties of the ARB valsartan and the NEP inhibitor prodrug AHU377 in a 1 : 1 ratio. In a study of 1328 patients, LCZ696 conferred greater blood pressure lowering than valsartan alone with no cases of angioedema reported. In a phase II study of 149 individuals with heart failure with preserved ejection fraction, LCZ696 lowered NT-proBNP to a greater extent than valsartan and was well tolerated. The efficacy of LCZ696 in comparison to enalapril for the treatment of systolic heart failure was assessed in the Prospective comparison of ARNI with ACEI to Determine Impact on Global Mortality and morbidity in Heart Failure trial (PARADIGM-HF); the study reported that LCZ696 was superior to enalapril in reducing the risks for death and for hospitalization for heart failure. Other molecules combining ARB and NEP inhibition are also currently under development.
Other Natriuretic Peptides
Guanylin and Uroguanylin
The existence of intestinal NPs has been suggested by initial observations that sodium excretion is greater after an oral salt load than after an intravenous salt load. These intestinal peptides include guanylin and uroguanylin. However, a study of 15 healthy volunteers found that sodium excretion was similar in response to either oral or intravenous sodium load during either a low- or high-sodium–containing diet. Moreover, serum concentrations of either prouroguanylin or proguanylin were unchanged following either oral or intravenous sodium load and showed no correlation with sodium excretion. Collectively, these observations challenge the notion of a gastrointestinal-renal natriuretic axis mediated by the guanylin peptide family. It thus appears likely that the natriuretic, kaliuretic, and diuretic effects of guanylin and uroguanylin, which occur without change in GFR or renal blood flow, are mediated by local production of the peptides within the kidney.
Adrenomedullin
Adrenomedullin is a 52–amino acid peptide originally isolated from human pheochromocytoma cells, although mainly synthesized by vascular smooth muscle cells, endothelial cells, and macrophages and present in the plasma, vasculature, lungs, heart, and adipose tissue. The peptide is upregulated in patients with cardiovascular disease and has positive inotropic and vasodilatory properties. Systemic administration of adrenomedullin induces a nitric oxide–dependent natriuresis and increase in GFR both under normal conditions and in patients with congestive heart failure, also decreasing plasma aldosterone level without affecting renin activity.
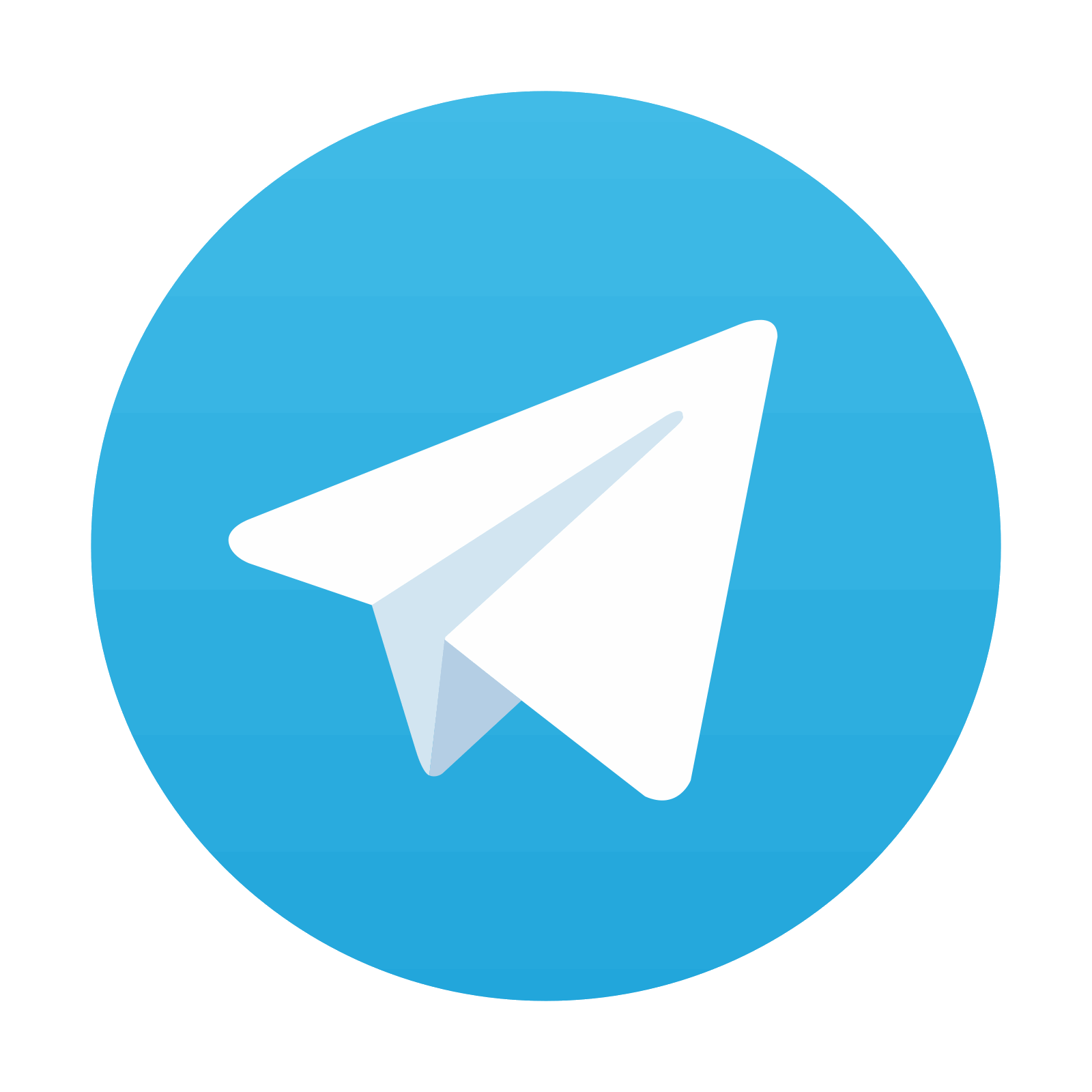
Stay updated, free articles. Join our Telegram channel
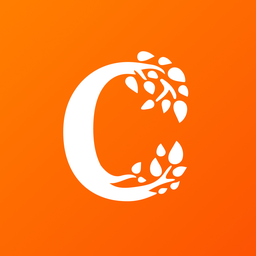
Full access? Get Clinical Tree
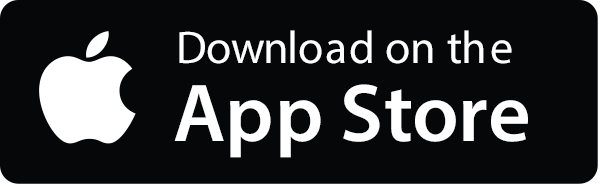
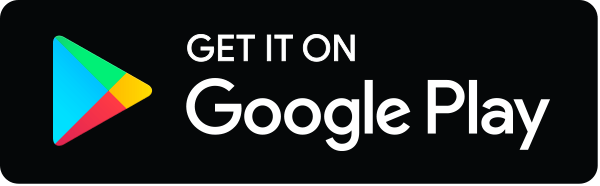