Chapter Outline
INDEPENDENT REGULATION OF WATER AND SALT EXCRETION, 258
CRITICAL ROLE OF PARALLEL ORGANIZATION OF STRUCTURES IN THE RENAL MEDULLA TO URINARY CONCENTRATING AND DILUTING PROCESS, 259
URINE CONCENTRATION AND DILUTION PROCESSES ALONG THE MAMMALIAN NEPHRON, 264
Sites of Urine Concentration and Dilution, 264
Mechanism of Tubule Fluid Dilution, 265
Mechanism of Tubule Fluid Concentration, 265
MOLECULAR PHYSIOLOGY OF URINARY CONCENTRATING AND DILUTING PROCESSES, 273
Aquaporin-1 Knockout Mice, 273
Aquaporin-2 Knockout Mice, 276
Aquaporin-3 and Aquaporin-4 Knockout Mice, 276
UT-A1/3 Urea Transporter Knockout Mice, 276
Na ++ -H ++ -Exchanger Isoform 3 and Na-K-2Cl Cotransporter Type 2 Knockout Mice, 278
Epithelial Sodium Channel Knockout Mice, 278
Kidney-Specific Chloride Channel 1 Knockout Mice, 278
Renal Outer Medullary Potassium Channel Knockout Mice, 278
Type 2 Vasopressin Receptor Knockout Mice, 278
Independent Regulation of Water and Salt Excretion
The kidney is responsible for numerous homeostatic functions. For example, body fluid tonicity is tightly controlled by regulation of renal water excretion; extracellular fluid volume is controlled by regulation of NaCl excretion; systemic acid-base balance is controlled by regulation of net acid excretion; systemic K + balance is controlled by regulation of K + excretion; and the body maintains nitrogen balance through regulation of urea excretion.
The independent regulation of water and solute excretion is essential for the homeostatic functions of the kidney to be performed simultaneously. This means that in the absence of changes in solute intake or metabolic production of waste solutes, the kidney is able to excrete different volumes of water upon changes in water intake. This ability to excrete the appropriate amount of water without marked perturbations in solute excretion (without disturbing the other homeostatic functions of the kidney) is dependent on renal concentrating and diluting mechanisms and forms the basis of this chapter ( Figure 10.1 ).

Renal water excretion is tightly regulated by the peptide hormone arginine vasopressin (AVP; also named antidiuretic hormone). Under normal circumstances, the circulating vasopressin level is determined by osmoreceptors in the hypothalamus that trigger increases in vasopressin secretion (by the posterior pituitary gland) when the osmolality of the blood rises above a threshold value, approximately 292 mOsm/kg H 2 O. This mechanism can be modulated when other inputs to the hypothalamus (e.g., arterial underfilling, severe fatigue, or physical stress) override the osmotic mechanism. Upon an increase in plasma osmolality, vasopressin is secreted from the posterior pituitary gland into the peripheral plasma. The kidney responds to the variable vasopressin levels by varying urine flow (i.e., water excretion). For example, during extreme antidiuresis (high vasopressin levels), water excretion is 100- or more-fold lower than during extensive water diuresis (low vasopressin levels). These major changes in water excretion are obtained without substantial changes in steady-state solute excretion. This phenomenon is dependent on the kidney’s ability to concentrate and dilute the urine. During low circulating vasopressin levels, urine osmolality is less than that of plasma (290 mOsm/kg H 2 O)—the diluting function of the kidney. In contrast, when the circulating vasopressin levels are high, urine osmolality is much higher than that of plasma—the concentrating function of the kidney.
Critical Role of Parallel Organization of Structures in the Renal Medulla to Urinary Concentrating and Diluting Process
The kidney’s ability to vary water excretion over a wide physiologic range, without altering steady-state solute excretion, cannot be simply explained as a consequence of the sequential transport processes along the nephron. The independent regulation of water and sodium excretion occurs in the renal medulla, where the nephron segments and vasculature (vasa recta) are arranged in complex but specific anatomic relationships, both in terms of which segments connect to which segments and their three-dimensional configuration. Thus it is necessary to consider the parallel interactions between nephron segments that occur as a result of its looped or hairpin structure. Figure 10.2 illustrates the regional architecture of the renal medulla and medullary rays.

Renal Tubules
Loops of Henle
The kidney generally contains two populations of nephrons, long-looped and short-looped, which merge to form a common collecting duct system (see Figure 10.2 ). Both types of nephrons have loops of Henle that are arranged in a folded or hairpin configuration. Short-looped nephrons generally have glomeruli that are located more superficially in the cortex and have loops that bend in the outer medulla. Long-looped nephrons generally have glomeruli that are located more deeply within the cortex and have loops that bend at various levels of the inner medulla. Long-looped nephrons also contain a thin ascending limb, a segment that is not present in short-looped nephrons. Thin ascending limbs are found only in the inner medulla. The inner-outer medullary border is defined by the transition from thin to thick ascending limbs. Thus the outer medulla contains only thick ascending limbs, regardless of the type of loop. The long-looped nephrons bend at various levels of the inner medulla from the inner-outer medullary border to the papillary tip. Thus progressively fewer loops of Henle extend to deeper levels of the inner medulla. Some mammalian kidneys, such as human kidneys, also contain cortical nephrons, which are nephrons whose loops of Henle do not reach into the medulla.
The loops of Henle receive tubule fluid from the proximal convoluted tubules. Tubule fluid exits the thick ascending limbs of both long- and short-looped nephrons, and from cortical nephrons in species that have them, and flows into distal convoluted tubules (DCTs). Thus the descending and ascending limbs of the loops of Henle have a countercurrent flow configuration and are composed of several different nephron segments (see Figure 10.2 ). The descending portion of the loop of Henle consists of the S2 proximal straight tubule in the medullary ray, the S3 proximal straight tubule (or pars recta) in the outer stripe of the outer medulla, and the thin descending limb in the inner stripe of the outer medulla and the inner medulla. The descending thin limb of short-looped nephrons differs structurally and functionally from the descending thin limb of long-looped nephrons.
The location of the descending thin limb of short-looped nephrons within the outer medulla is illustrated in Figure 10.3 (labeled in green). The descending thin limbs of short-looped nephrons surround the vascular bundles in the outer medulla and tend to be organized in a ringlike pattern (see Figure 10.3 inset ). Thin descending limbs of long-looped nephrons in the outer medulla differ morphologically and functionally from thin descending limbs of long-looped nephrons in the inner medulla. The histologic transition from the outer medullary to the inner medullary type of thin descending limbs of long-looped nephrons is gradual and often occurs at some distance into the inner medulla, rather than strictly at the inner-outer medullary border as is the case for the transition between thin and thick ascending limbs.

Pannabecker, Dantzler, and coworkers used immunohistochemical labeling and computer-assisted reconstruction to provide new detail about the functional architecture of the rat inner medulla. Figure 10.4 shows a computerized reconstruction of the inner medullary portion of several long-looped nephrons from rats that are labeled using antibodies to the water channel aquaporin-1 (AQP1, shown in red) and the kidney-specific chloride channel 1 (ClC-K1; shown in green). AQP1 is a marker of thin descending limbs of long-looped nephrons in the outer medulla, and AQP1 is detected in thin descending limbs of long-looped nephrons in the inner medulla for a variable distance. However, AQP1 was not found in thin descending limbs of loops of Henle that turn within the upper first millimeter of the inner medulla. Correspondingly, Zhai and colleagues determined that AQP1 was not detectable along the entire length of thin descending limbs of short-looped rat nephrons. In contrast, thin descending limbs of loops of Henle that turn below the first millimeter have three discernible functional subsegments: the upper 40% express AQP1, whereas the lower 60% do not. ClC-K1 is a marker of the thin ascending limb–type epithelium. It is first detected in the final approximately165 µm of the thin descending limb. Thus, ClC-K1 is detected before the bend of the loops of Henle, consistent with several morphologic studies that demonstrate that the descending limb to ascending limb transition occurs before the loop bend. A substantial portion of the inner medullary thin descending limb of long-looped nephrons did not express either AQP1 or ClC-K1, as indicated in gray.

The deepest portions of descending thin limbs have low water permeability and reduced AQP1. It has been proposed, but not demonstrated experimentally, that urine concentration would be improved by the presence of a urea-Na + or urea-Cl − cotransporter in the AQP1-null portion of the thin descending limb. These deep AQP1-null segments, prebend segments, and thin ascending limbs lie equally near the collecting ducts. However, the distal 30% of thin ascending limbs of the longest loops of Henle lie distant from collecting ducts. Based upon these findings, Pannabecker and colleagues hypothesize that descending and ascending thin limbs enter and exit collecting duct clusters in a manner that is important for the generation and maintenance of the osmolality gradient within the inner medulla. These anatomic relationships result in isolated interstitial nodal spaces that may facilitate preferential mixing of solutes and fluid within the inner medulla.
Pannabecker and colleagues have also found evidence for mixed descending- and ascending-type thin limbs of the loops of Henle in the inner medulla. The ascending portions of the loops of Henle consist of the thin ascending limbs (which are present only in the long-looped nephrons of the inner medulla), the medullary thick ascending limbs in the inner and outer stripes of the outer medulla, and the cortical thick ascending limbs in the medullary rays.
Distal Tubule Segments in the Cortical Labyrinth
After tubule fluid exits the loop of Henle through the cortical thick ascending limb, it enters the DCT, which is located in the cortical labyrinth. In most mammalian species, several distal tubules merge to form a connecting tubule arcade. The connecting tubular cells express both the vasopressin-regulated water channel, aquaporin-2 (AQP2), and the type 2 vasopressin receptor (V2R), suggesting that the arcades are sites of vasopressin-regulated water reabsorption, similar to collecting ducts (see later). Tubule fluid exits the connecting tubules within the arcades and enters the initial collecting tubules, located in the superficial cortex, and then into the cortical collecting ducts. In most rodent species that have been studied, several nephrons merge to form a single cortical collecting duct.
Collecting Duct System
The collecting duct system spans all regions of the kidney, starting in the cortex and running to the tip of the inner medulla (see Figure 10.2 ). The collecting ducts are arranged parallel to the loops of Henle in the medullary rays, outer medulla, and inner medulla. Like the loops of Henle, several morphologically and functionally discrete segments are contained within the collecting duct system. In general, the collecting ducts descend straight through the medullary rays and outer medulla without joining with other collecting ducts. However, several collecting ducts merge as they descend within the inner medulla, resulting in a progressive reduction in the number of inner medullary collecting ducts (IMCDs) from the inner-outer medullary border to the papillary tip. The tapered structure of the renal papilla results from the reduction in collecting duct number, accompanied by a progressive reduction in the number of loops of Henle, reaching the deepest levels of the inner medulla.
Detailed studies of inner medullary structure, both by Kriz and coworkers and in later studies by Pannabecker and Dantzler, found that the IMCDs in the inner medullary base (initial IMCDs) form clusters that coalesce along the corticomedullary axis. The thin descending limbs are predominantly present at the periphery of these clusters and appear to form an asymmetric ring around each collecting duct cluster, whereas the thin ascending limbs are distributed relatively uniformly among the collecting ducts and thin descending limbs.
Pannabecker and Dantzler identified three population groups of loops of Henle in Munich-Wistar rats, distinguished by the position of the thin ascending limb at the base of the inner medulla and by differing loop length ( Figure 10.5 ). Group 1 loops have thin ascending limbs that are interposed between collecting ducts and reach a mean length of 700 µm into the inner medulla. Group 2 loops have thin ascending limbs that are adjacent to just one collecting duct and reach a mean length of 1500 µm. Group 3 loops have thin ascending limbs that lie more than 0.5 tubule diameters from a collecting duct and reach a mean length of 2200 µm. As the collecting ducts coalesce and the shorter loops of Henle disappear, the originating portions of the longer thin ascending limbs run alongside the collecting ducts for a substantial distance.

Vasculature
For detailed descriptions of the renal vasculature, see Chapters 2 and 3 . The major blood vessels that carry blood into and out of the renal medulla are named the vasa recta . Blood enters the descending vasa recta from the efferent arterioles of juxtamedullary nephrons and supplies it to the capillary plexuses at each level of the medulla. The outer medullary capillary plexus is denser and better perfused than the plexus in the inner medulla. Blood from the inner medullary capillary plexus feeds into ascending vasa recta (ascending vasa recta are never formed directly from descending vasa recta in a looplike structure). Inner medullary ascending vasa recta traverse the inner stripe of the outer medulla in close physical association with the descending vasa recta in vascular bundles. In many animal species, thin descending limbs of short-looped nephrons surround the vascular bundles, as shown in Figure 10.3 . Here the thin descending limb segments are labeled with an antibody to the UT-A2 urea transporter, suggesting a route for urea recycling from the vasa recta to the thin descending limbs of short-looped nephrons. The outer medullary capillary plexus is drained by vasa recta that ascend through the outer stripe of the outer medulla, separate from the descending vasa recta. Computer-assisted digital tracing of mouse kidney combined with AQP1 immunohistochemistry has added to our knowledge of the arrangement of tubules and vessels in the vascular bundles being implicit in providing a lateral osmolality heterogeneity for urine concentration.
The counterflow arrangement of the vasa recta in the medulla promotes countercurrent exchange of solutes and water, which is abetted by the presence of AQP1 and UT-B urea transporters in the endothelial cells of the descending portion of the vasa recta. Countercurrent exchange provides a means of reducing the effective blood flow to the medulla while maintaining a high absolute perfusion rate. The low effective blood flow that results from countercurrent exchange is thought to be important for the preservation of solute concentration gradients in the medullary tissue (see later).
In contrast to the medulla, the cortical labyrinth has a high effective blood flow. The rapid vascular perfusion to this region promotes the rapid return of solutes and water reabsorbed from the nephron to the general circulation. The rapid perfusion is thought to maintain the interstitial concentrations of most solutes at levels close to those in the peripheral plasma. The medullary rays of the cortex have a capillary plexus that is considerably sparser than that of the cortical labyrinth. Consequently, the effective blood flow to the medullary rays has been postulated to be lower than that of the cortical labyrinth.
Medullary Interstitium
The renal medullary interstitium connects the tubules and vasculature. It is a complex space that includes the medullary interstitial cells, microfibrils, extracellular matrix, and fluid. The interstitium is relatively small in volume in the outer medulla and the outer portion of the inner medulla, which may be important in limiting the diffusion of solutes upward along the medullary axis. In contrast, the interstitial space is much larger in the inner half of the inner medulla. Within this region, it consists of a gelatinous matrix containing large amounts of highly polymerized hyaluronic acid, consisting of alternating N -acetyl-D-glucosamine and D-glucuronate moieties. Theories have been proposed in which the hyaluronic acid interstitial matrix plays a direct role in the generation of an inner medullary osmotic gradient through its ability to store and transduce energy from the smooth muscle contractions of the renal pelvis (see later).
Renal Pelvis
Urine exits the collecting duct system through the ducts of Bellini at the papillary tip and enters the renal pelvis ( Figure 10.6 ). The renal pelvis (or calyx in multipapillate kidneys) is a complex intrarenal urinary space that surrounds the papilla. The renal pelvis (or calyx) has portions that extend into the outer medulla, which are called fornices and secondary pouches. Although a transitional epithelium lines most of the pelvic space, the renal parenchyma is separated from the pelvic space by a simple cuboidal epithelium. It has been proposed that water and solute transport could occur across this epithelium, thereby modifying the composition of the renal medullary interstitial fluid. There are two smooth muscle layers within the renal pelvic (calyceal) wall. Contractions of these smooth muscle layers generate powerful peristaltic waves that appear to displace the renal papilla downward with a “milking” action. These peristaltic waves may intermittently propel urine along the collecting ducts. The contractions compress all structures within the renal inner medulla, including the interstitium, loops of Henle, vasa recta, and collecting ducts. Theories have been proposed whereby these contractions furnish part of the energy for concentrating solutes, and hence concentrating urine, within the inner medulla, as discussed later.

Urine Concentration and Dilution Processes Along the Mammalian Nephron
Sites of Urine Concentration and Dilution
Micropuncture studies of the mammalian nephron have determined the major sites of tubule fluid concentration and dilution ( Figure 10.7 ). Proximal tubule fluid is always isosmotic with plasma, regardless of whether the kidney is diluting or concentrating the urine. In contrast, the fluid in the early DCT is always hypotonic, regardless of the final osmolality of the urine. Indeed, during both antidiuresis and water diuresis, the earliest nephron segment where significant differences in tubule fluid osmolality can be detected is the late distal tubule. During water diuresis, the fluid in the distal tubule remains hypotonic. During antidiuresis, the fluid in the distal tubule becomes isosmotic with plasma, and the osmolality between the end of the late distal tubule and the IMCD rises to a level greater than that of plasma. Thus the conclusion from micropuncture studies is that the loop of Henle is the major site of dilution of tubule fluid, and that dilution processes in the loop occur regardless of whether the final urine is dilute or concentrated. Further dilution of the tubule fluid can occur in the collecting ducts during water diuresis. In contrast, the chief site of urine concentration is beyond the distal tubule (i.e., in the collecting duct system). The mechanisms of urinary dilution and of urinary concentration are discussed in the following sections.

Mechanism of Tubule Fluid Dilution
Micropuncture studies in rats have shown that the fluid in the early distal tubule is hypotonic, due mainly to a reduction in luminal NaCl concentration relative to that in the proximal tubule. The low luminal NaCl concentration could result either from active NaCl reabsorption from the loop of Henle or from water secretion into the loop of Henle. Micropuncture measurements in rats, performed using inulin as a volume marker, demonstrated net water reabsorption from the superficial loops of Henle during antidiuresis, thereby ruling out water secretion as a potential mechanism of tubule fluid dilution. Thus one can conclude that luminal dilution occurs because of NaCl reabsorption from the loops of Henle, in excess of water reabsorption. Classic studies of isolated perfused rabbit thick ascending limbs established the mechanism of tubule fluid dilution. NaCl is rapidly reabsorbed by active transport, which lowers the luminal osmolality and NaCl concentration to levels below those in the peritubular fluid. The osmotic water permeability of the thick ascending limb is very low, which prevents dissipation of the transepithelial osmolality gradient by water flux.
The tubule fluid remains hypotonic throughout the distal tubule and collecting duct system during water diuresis, aided by the low osmotic water permeability of the collecting ducts when circulating levels of vasopressin are low. Even though the tubule fluid remains hypotonic in the collecting duct system, the solute composition of the tubule fluid is modified within the collecting duct, mainly by Na + absorption and K + secretion. Active NaCl reabsorption from the collecting duct results in a further dilution of the collecting duct fluid, beyond that achieved in the thick ascending limbs.
Mechanism of Tubule Fluid Concentration
When circulating vasopressin levels are high, net water absorption occurs between the late distal tubule and the collecting ducts. The reader is referred to Chapter 11 for a detailed discussion of the cell biology of vasopressin action. Because water is absorbed in excess of solutes, with a resulting rise in osmolality along the collecting ducts toward the papillary tip, it can be concluded that collecting duct fluid is concentrated chiefly by water absorption, rather than by solute addition.
An axial osmolality gradient in the renal medullary tissue, with the highest degree of hypertonicity at the papillary tip, provides the osmotic driving force for water absorption along the collecting ducts. This osmolality gradient was initially reported by Wirz and colleagues. In a classic study they demonstrated, in antidiuretic rats, the existence of a continuously increasing osmolality gradient along the outer and inner medulla, with the highest osmolality in the deepest part of the inner medulla, the papillary tip. In addition, within the medulla the osmolality of the collecting ducts was as high as in the loops of Henle, and the osmolality of vasa recta blood, sampled from near the papillary tip, was virtually equal to that of the final urine. Taken together these results demonstrated that the high tissue osmolality was not simply a manifestation of a high osmolality in a single structure, namely, the collecting duct. Micropuncture studies by Gottschalk and Mylle based on the superficial and thus accessible tubules and vessels confirmed that the osmolality of the fluid in the loops of Henle, the vasa recta, and the collecting ducts is approximately the same (see Figure 10.7 ), supporting the hypothesis that the collecting duct fluid is concentrated by osmotic equilibration with a hypertonic medullary interstitium. Furthermore, in vitro studies demonstrated that collecting ducts have a high water permeability in the presence of vasopressin, as is required for osmotic equilibration. The mechanism by which the corticomedullary osmolality gradient is generated is considered in the next section.
Although the final axial osmolality gradient within the renal medulla is due to the combined gradients of several individual solutes, as initially demonstrated using tissue slice analysis by Jarausch and Ullrich, the principal solutes responsible for the osmolality gradient are NaCl and urea ( Figure 10.8 ). The increase in the NaCl concentration gradient along the corticomedullary axis occurs predominantly in the outer medulla, with only a small increase in the inner medulla. In contrast, the increase in urea concentration occurs predominantly in the inner medulla, with little or no increase in the initial outer medulla. The mechanisms for generating the NaCl gradient in the outer medulla and urea accumulation in the inner medulla are discussed in the next section.

Generation of the Axial Sodium Chloride Gradient in the Renal Outer Medulla
A sustained osmolality gradient is maintained along the corticomedullary axis of the outer medulla (see Figure 10.8 ); that gradient, which is present in both diuresis and antidiuresis, arises mostly from an accumulation of NaCl. The gradient is generated by the concentrating mechanism of the outer medulla. General considerations circumscribe the nature of that mechanism. Because the axial osmolality gradient is present in both diuresis and antidiuresis (in which the outer medullary collecting duct is water permeable to varying degrees), the accumulation of NaCl in the outer medulla cannot depend on a sustained osmolality difference across the collecting duct epithelium. Thus the concentrating mechanism must depend on the loops of Henle, on the vasculature, and on their interactions within the outer medulla. Moreover, mass balance of water and NaCl must be maintained; thus, for example, concentrated fluid that flows into the inner medulla must be balanced by dilute fluid that, in the presence of vasopressin, is absorbed from the cortical collecting duct, dilutes the cortical interstitial fluid, enters the cortical vasculature, and thus participates in maintaining an appropriate systemic level of blood plasma osmolality.
It has long been believed that the osmolality gradient of the outer medulla is generated by means of countercurrent multiplication of a single effect (“Vervielfältigung des Einzeleffektes”). In this paradigm, proposed by Kuhn and Ryffel in 1942, osmotic pressure is raised along parallel but opposing flows in nearby tubes that are made contiguous by a hairpin turn ( Figure 10.9 ): a transfer of solute from one tubule to another (i.e., a single effect) would augment (multiply), or reinforce, the osmotic pressure in the parallel flows. Thus, by means of the countercurrent configuration, a small transverse osmotic difference would be multiplied into a relatively large difference along the axes of flow. In support of this paradigm, Kuhn and Ryffel provided both a mathematical model and an apparatus that exemplified countercurrent multiplication.

As anatomic and physiologic understanding of the renal medulla increased, the countercurrent multiplication paradigm was reinterpreted and modified. In 1951 Hargitay and Kuhn put the paradigm in the context of specific renal tubules. The loop of Henle was identified with the parallel tubes joined by a hairpin turn as proposed by Kuhn and Ryffel. Thus the loops of Henle were proposed as the source of the outer medullary gradient, and that gradient was hypothesized to draw water out of water-permeable collecting ducts. In 1959 Kuhn and Ramel used a mathematical model to show that active transport of NaCl from thick ascending limbs could serve as the single effect. Subsequent physiologic experiments confirmed the active NaCl transport and the osmotic absorption of water from collecting ducts. Experiments indicating high water permeability in hamster descending limbs of short loops and in descending limbs of long loops suggested that the accumulation of NaCl from thick limbs concentrated descending limb tubule fluid by osmotic water withdrawal, rather than by NaCl addition (see Figure 10.9 ).
Doubts have arisen about whether the paradigm of countercurrent multiplication provides an accurate representation of the means by which the gradient is generated in the mammalian outer medulla. Several weaknesses have been noted:
- 1.
The descending limbs of short loops are anatomically separated from ascending limbs, with inner stripe portions of short loops near (or within) the vascular bundles and thick limbs near the collecting ducts. This configuration is not consistent with direct interactions between counterflowing limbs.
- 2.
In short-looped rat nephrons, Wade and associates found that AQP1 is not expressed in portions of descending limb segments in the distal inner stripe. Zhai and colleagues found that AQP1 is not expressed in descending limbs of short loops in the inner stripes of mice, rats, and humans. The absence of AQP1 suggests that the assumption of high water permeability in descending limbs of short loops merits further experimental study. (Dantzler and coworkers have found that the AQP1-null segments of descending limbs in the inner medulla are essentially impermeable to water. )
- 3.
Using transport rates based on measured sodium-potassium adenosine triphosphatase (Na + -K + -ATPase) activities, mathematical models predict substantial transepithelial NaCl gradients along the medullary portions of thick limbs ; this seems contrary to the notion of a small single effect.
- 4.
It may well be that the concentrating mechanism of the outer medulla is placed under increased load, not less, if it has to concentrate water flowing in, and absorbed from, the descending limbs of short loops.
- 5.
The absorption of water from the outer medullary portions of long loops has sometimes been considered to participate in a generalized form of countercurrent multiplication, and this may be the case for long loops that extend for sufficiently short distances into the inner medulla. However, in sufficiently long loops, tubule fluid is likely to be much altered by urea secretion and passive NaCl absorption within the inner medulla.
From these considerations, it seems reasonable therefore to hypothesize that the outer medullary osmolality gradient arises principally from vigorous active transport of NaCl, without accompanying water, from the thick ascending limbs of short- and long-looped nephrons. The tubule fluid of the thick limbs that enters the cortex is diluted well below plasma osmolality, and thus, the requirement of mass balance is met. In rats and mice, the thick limbs are localized near the collecting ducts ; mathematical models suggest that at a given level of the outer medulla, the interstitial osmolality will be higher near the collecting ducts than near the vascular bundles. This higher osmolality will facilitate water withdrawal from the descending limbs of long loops and from collecting ducts. Descending vasa recta are thought to be found only in the vascular bundles. Thus the ascending vasa recta will act as the collectors of any NaCl that is absorbed from loops of Henle and water that is absorbed from the descending limbs of long loops and from collecting ducts.
The countercurrent configuration of the ascending vasa recta, relative to the descending limbs and collecting ducts, is likely to participate in sustaining the axial gradient: as ascending vasa recta fluid ascends toward the cortex, its osmolality will exceed that in the descending limbs of long loops and in the collecting ducts. Thus ascending vasa recta fluid will be progressively diluted as that fluid contributes to the concentrating of fluid in descending limbs of long loops and in collecting ducts, by giving up NaCl to, and absorbing water from, the interstitium ( Figure 10.10 ).

The previous summary appears to account for the elevation of osmolality in the outer medulla without invoking a role for countercurrent multiplication. However, a question remains: why does the osmolality gradient increase along the outer medulla as a function of increasing medullary depth? The answer likely lies in the local balance of NaCl absorption from thick limbs and water absorption from descending limbs of long loops and from collecting ducts. At deeper medullary levels, the rate of NaCl absorption from thick limbs may be higher than at shallow levels, owing to a higher Na + -K + -ATPase activity at deeper levels and to a saturation of transport proteins by the higher NaCl concentration in thick limb tubule fluid before dilution. Moreover, because of the water already absorbed in the upper outer medulla, the load of water presented to the thick limbs deep in the outer medulla by descending limbs of long loops and by the collecting ducts is much reduced.
A caveat is in order. Our understanding of the outer medulla is mostly based on information obtained from heavily studied laboratory animals, especially rats and mice. Outer medullary function and structure are likely to vary substantially in other species. For example, the human kidney has limited concentrating capability (relative to many other mammals), and only approximately one seventh of the loops of Henle are long ; the mountain beaver (Aplodontia rufa) has mostly cortical loops of Henle and essentially no inner medulla. It seems likely that the outer medullary structure in these species differs substantially from that in rats and mice. Finally, it should be acknowledged that the paradigm formulated earlier is similar to that proposed by Berliner and coworkers in 1958.
Accumulation of Urea in the Renal Inner Medulla
Urea accumulation within the inner medulla is partly dependent on variable urea permeabilities along the collecting duct system ( Figure 10.11 ). Within the collecting duct system, only the terminal portion of the IMCD possesses high urea permeability, which can be further increased by vasopressin. The effects of vasopressin are mediated (at least in part) by the secondary messenger cyclic adenosine monophosphate (cAMP). Urea-specific transporters are localized to the apical and basolateral plasma membranes of the IMCD cells and are responsible for the high urea permeability of the terminal portion of the IMCD.

The mechanisms of urea accumulation in the renal medulla are depicted in Figure 10.12 . Accumulation of urea is predominantly a result of passive urea reabsorption from the IMCD. Tubule fluid entering the collecting duct system in the renal cortex has a relatively low urea concentration. However, during antidiuresis, water is osmotically reabsorbed from the urea-impermeable parts of the collecting duct system in the cortex and outer medulla, causing a progressive increase in the luminal urea concentration along the connecting tubules, cortical collecting ducts, and outer medullary collecting ducts. Thus, when the tubule fluid reaches the highly urea-permeable terminal IMCD (due to the presence of urea transporters), urea rapidly exits from the lumen to the inner medullary interstitium, where it is “trapped” by countercurrent urea exchange between descending and ascending flows in both vasa recta and loops of Henle. Under steady-state conditions, and in the continued presence of vasopressin, urea nearly equilibrates across the IMCD epithelium and thus osmotically balances the urea in the collecting duct lumen, preventing possible instances of osmotic diuresis ( Figure 10.13 ).


The descending and ascending vasa recta are in close association with each other in the inner medulla, facilitating countercurrent exchange of urea between the two structures. In the ascending vasa recta, aided by the extremely high (>40 × 10 −5 cm/sec) permeability to urea, the concentration of urea exiting the inner medulla is similar to the concentration of urea in the descending vasa recta. This minimizes the washout of urea from the inner medulla. However, countercurrent exchange cannot completely eliminate loss of urea from the inner medullary interstitium, because the volume flow rate of blood in the ascending vasa recta exceeds that in the descending vasa recta. During antidiuresis, water is added to the vasa recta from both IMCDs and descending limbs, resulting in a higher volume flow rate and an increased mass flow rate of urea. This ensures that the inner medullary vasculature continually removes urea from the inner medulla. Quantitatively, the most important loss of urea from the inner medullary interstitium is thought to occur via the vasa recta, but urea recycling pathways play a major role in limiting the loss of urea from the inner medulla. These pathways have been further elucidated via the use of knockout mouse models (see later). Three major urea recycling pathways are described in the following list, and an overview of these is shown in Figure 10.14 .
- 1.
Recycling of urea through the ascending limbs, distal tubules, and collecting ducts. Urea that escapes the inner medulla in the ascending limbs of the long loops of Henle is carried back through the thick ascending limbs, DCTs, and early portions of the collecting duct system by the flow of tubule fluid. When it reaches the urea-permeable part of the IMCDs, it passively exits into the inner medullary interstitium and starts the cycle again.
- 2.
Recycling of urea through the vasa recta, short loops of Henle, and collecting ducts. The delivery of urea to the superficial distal tubule exceeds the delivery out of the superficial proximal tubule. This implies that net urea addition occurs somewhere along the short loops of Henle. One possible mechanism is that the urea leaving the inner medulla in the vasa recta is transferred to the descending limbs of the short loops of Henle and is subsequently carried through the superficial distal tubules back to the urea-permeable part of the IMCDs, where it passively exits, completing the recycling pathway. The close physical association between the vasa recta and the descending limbs of the short loops in the vascular bundles of the inner stripe of the outer medulla would facilitate this transfer of urea from the vasa recta to the short loops of Henle. Furthermore, the existence of a facilitative urea transporter, UT-A2, in the thin descending limb of the short loops of Henle provides further support for this mechanism. However, later studies on UT-A2 knockout mice and UT-A2/UT-B knockout mice have raised doubts about the importance of this pathway.
- 3.
Urea recycling between ascending and descending limbs of the loops of Henle. The urea permeability of thick ascending limbs from the inner stripe of the outer medulla is low. However, the urea permeability of thick ascending limbs from the outer stripe of the outer medulla and the medullary rays is relatively high. Based on this, a urea recycling pathway has been proposed in which urea is reabsorbed from thick ascending limbs and is secreted into neighboring proximal straight tubules, forming a recycling pathway between the ascending limbs and the descending limbs of the loop of Henle. Urea recycling from the thick ascending limbs and the proximal straight tubules is facilitated by the parallel relationship of these two structures in the outer stripe of the outer medulla and in the medullary rays. This transfer of urea is also likely to depend on a relatively attenuated effective blood flow in these regions. Urea secretion into the proximal straight tubules can occur by passive diffusion, active transport, or a combination of both. Urea presumably enters the proximal straight tubules of both short- and long-looped nephrons. The urea that enters the short-looped nephrons will be carried back to the inner medulla by the flow of tubule fluid through the superficial distal tubules and cortical collecting ducts, reentering the inner medullary interstitium by reabsorption from the terminal IMCD. The urea that enters proximal straight tubules of long-looped nephrons returns to the inner medulla directly through the descending limbs of the loops of Henle.
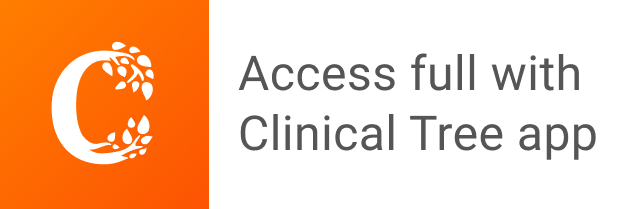