Outline
Introduction 389
Basic Components of Tissue Engineering 390
Cells 390
Native Cells 390
Embryonic Stem Cells 390
Therapeutic Cloning (Somatic Cell Nuclear Transfer) 391
Reprogrammed Somatic Cells 391
Placental and Amniotic Fluid Stem Cells 392
Biomaterials 392
Tissue Engineering of Specific Genitourinary Organs 394
Conclusion 398
Acknowledgment 398
There is a critical shortage of organs available for donation to patients suffering from degenerative diseases of various organ systems, including the genitourinary system. This shortage becomes more severe yearly, as the aging population grows and such diseases become more common. The fields of regenerative medicine and tissue engineering now offer hope for these patients with new advances in material science, cell transplantation and bioengineering. Novel methods and materials to construct biological substitutes for diseased and injured tissues are being developed, and the goal is to restore and maintain normal function to the patient. The field of stem cell research is rapidly advancing, offering unforeseen options for treatment. For example, therapeutic cloning, where an enucleated oocyte receives a donor cell nucleus, yielding pluripotent stem cells, offers a potentially limitless source of cells for tissue engineering applications. However, recent discoveries in this field indicate that the use of non-controversial cells and tissues also has enormous potential for tissue engineering purposes. This article gives an overview of recent advances in regenerative medicine of the genitourinary tract, and describes their applications in tissue and organ replacement technology, as well as how these technologies offer new therapies to a person facing end-stage organ failure. There is a growing optimism that the successes in this field will achieve the goal of significantly extending the life of the patient.
Introduction
Patients suffering from diseased or injured genitourinary organs are often treated with reconstructive surgery or transplants, but there is a severe shortage of donor tissue and organs. The aging population grows, and the need for organs grows with it. Physicians and scientists have begun to look to the fields of regenerative medicine and tissue engineering to provide new options for these patients. These fields apply the principles of cell transplantation, material science and bioengineering to construct biological substitutes that can significantly improve the quality of life of the urological patient by eliminating the need for intensive grafting procedures or transplant surgery.
Tissue engineering, one of the major components of regenerative medicine, follows the principles of cell transplantation, materials science and engineering to develop biological substitutes that can restore and maintain normal organ function. Tissue engineering strategies generally fall into two categories: the use of acellular matrices designed to direct the body’s natural ability to use its own cells to regenerate damaged tissue, and the use of matrices seeded with cells in the laboratory to produce novel tissues and organs. Acellular tissue matrices are usually prepared by manufacturing artificial scaffolds, or by removing cellular components from donor tissues via mechanical and chemical manipulation to produce collagen-rich matrices ( ; and see Chapter 16 ). These matrices slowly degrade after implantation and are replaced by the extracellular matrix (ECM) proteins secreted by the ingrowing cells. Cells themselves can also be used for therapy via injection, either with carriers such as hydrogels or alone.
The most common way to use cells in tissue engineering is to obtain a small piece of donor tissue and dissociate it into individual cells in the laboratory. These cells are either implanted directly into the host or expanded in culture and attached to a support matrix. The cell–matrix construct is then reimplanted into the host. The source of the donor tissue can be heterologous (such as bovine), allogeneic (same species, different individual) or autologous. Ideally, autologous cells are used, because in this case both structural and functional tissue replacement will usually occur with minimal complications. To accomplish this, a biopsy of tissue is obtained from a host, the cells are dissociated and expanded in culture, and the expanded cells are implanted back into the same host . The use of autologous cells, although it may cause an inflammatory response, avoids rejection and thus, the deleterious side-effects of lifelong immunosuppression can be avoided.
However, for many patients with extensive end-stage organ failure, a tissue biopsy may not yield enough normal cells for expansion and transplantation. In other instances, primary autologous cells cannot be expanded from a particular organ, such as the pancreas. In these situations, stem cells are envisioned as an alternative source of cells from which the desired tissue can be derived. Stem cells can be derived from discarded human embryos (human embryonic stem cells), from fetal tissue or from adult sources (bone marrow, fat, skin). However, there are ethical issues involved in the use of embryonic stem cells (ESCs) and most human applications are currently banned in the USA. Despite this, the field of stem cell biology is advancing rapidly, and cutting-edge techniques such as therapeutic cloning and somatic cell reprogramming circumvent some of the ethical questions and offer a potentially limitless source of these cells for tissue engineering applications.
This chapter will review the major components of most tissue engineering techniques, and will describe how these techniques are being applied to the reconstruction and regeneration of the genitourinary system.
Basic Components of Tissue Engineering
Cells
Native Cells
In the past, one of the limitations of applying cell-based regenerative medicine techniques to organ replacement was the inherent difficulty of growing certain cell types in large quantities. Even when some organs, such as the liver, have a high regenerative capacity in vivo, cell growth and expansion in vitro can be difficult. By studying the privileged sites for committed precursor cells in these organs, as well as by exploring the conditions that promote differentiation and/or self-renewal of these cells, it has been possible to overcome some of the obstacles that limit cell expansion in vitro. One example is the urothelial cell. Urothelial cells could be grown in the laboratory setting in the past, but only with limited success. Several protocols were developed over the past two decades that identify the undifferentiated cells in a mixed culture of cells isolated from the urinary tract, and keep them undifferentiated during their growth phase . Using these methods of cell culture, it is now possible to expand a urothelial culture that initially covered a surface area of 1 cm 2 to one covering a surface area of 4202 m 2 (the equivalent of one football field) within 8 weeks . These studies indicated that it should be possible to collect autologous bladder cells from human patients, expand them in culture and return them to the donor in sufficient quantities for reconstructive purposes . Major advances in cell culture techniques have been made within the past decade, and these techniques make the use of autologous cells possible for clinical application.
Embryonic Stem Cells
In 1981, pluripotent cells were found in the inner cell mass of the human embryo, and the term “human embryonic stem cell” (hESC) was coined . These cells are able to differentiate into all cells of the human body, excluding placental cells (only cells from the morula are totipotent; that is, able to develop into all cells of the human body). These cells have great therapeutic potential, but their use is limited by both biological and ethical factors.
The political controversy surrounding stem cells began in 1998 with the creation of hESCs derived from discarded embryos. hESCs were isolated from the inner cell mass of a blastocyst (an embryo 5 days postfertilization) using an immunosurgical technique. Given that some cells cannot be expanded ex vivo, ESCs could be the ideal resource for tissue engineering because of their fundamental properties: the ability to self-renew indefinitely and the ability to differentiate into cells from all three embryonic germ layers. Skin and neurons have been formed, indicating ectodermal differentiation . Blood, cardiac cells, cartilage, endothelial cells and muscle have been formed, indicating mesodermal differentiation . Pancreatic cells have been formed, indicating endodermal differentiation . In addition, as further evidence of their pluripotency, ESCs can form embryoid bodies, which are cell aggregations that contain all three embryonic germ layers while in culture, and can form teratomas in vivo . These cells have demonstrated longevity in culture and can maintain their undifferentiated state for at least 80 passages when grown using current published protocols .
However, in addition to the ethical dilemma surrounding the use of ESCs, their clinical application is limited because they represent an allogenic resource and thus have the potential to evoke an immune response. New stem cell technologies (such as somatic cell nuclear transfer and reprogramming) promise to overcome this limitation.
Therapeutic Cloning (Somatic Cell Nuclear Transfer)
Somatic cell nuclear transfer (SCNT), or therapeutic cloning, entails the removal of an oocyte nucleus in culture, followed by its replacement with a nucleus derived from a somatic cell obtained from a patient. Activation with chemicals or electricity stimulates cell division up to the blastocyst stage.
At this point, it is extremely important to differentiate between the two types of cloning that exist: reproductive cloning and therapeutic cloning. Both involve the insertion of donor DNA into an enucleated oocyte to generate an embryo that has identical genetic material to its DNA source. However, the similarities end there. In reproductive cloning, the embryo is then implanted into the uterus of a pseudopregnant female to produce an infant that is a clone of the donor. A world-famous example of this type of cloning resulted in the birth of a sheep named Dolly in 1997 . However, there are many ethical concerns surrounding such practices, and as a result, reproductive cloning has been banned in most countries (see also Chapter 25 ).
While therapeutic cloning also produces an embryo that is genetically identical to the donor, this process is used to generate blastocysts that are explanted and grown in culture, rather than in utero. ESC lines can then be derived from these blastocysts, which are only allowed to grow up to a 100-cell stage. At this time the inner cell mass is isolated and cultured, resulting in ESCs that are genetically identical to the patient. This process is detailed in Fig. 24.1 . It has been shown that nuclear transferred ESCs derived from fibroblasts, lymphocytes and olfactory neurons are pluripotent and can generate live pups after injection into blastocysts. This shows that cells generated by SCNT have the same developmental potential as blastocysts that are fertilized and produced naturally . In addition, the ESCs generated by SCNT are perfectly matched to the patient’s immune system and no immunosuppressants would be required to prevent rejection should these cells be used in tissue engineering applications.

Although ESCs derived from SCNT contain the nuclear genome of the donor cells, mitochondrial DNA (mtDNA) contained in the oocyte could lead to immunogenicity after transplantation. To assess the histocompatibility of tissue generated using SCNT, Lanza et al. microinjected the nucleus of a bovine skin fibroblast into an enucleated oocyte . Although the blastocyst was implanted (reproductive cloning), the purpose was to generate renal, cardiac and skeletal muscle cells, which were then harvested, expanded in vitro and seeded onto biodegradable scaffolds. These scaffolds were then implanted into the donor steer from which the cells were cloned to determine whether cells were histocompatible. Analysis revealed that cloned renal cells showed no evidence of T-cell response, suggesting that rejection will not necessarily occur in the presence of oocyte-derived mtDNA. This finding represents a step forward in overcoming the histocompatibility problem of stem cell therapy.
Although promising, SCNT has certain limitations that require further improvement before its clinical application, in addition to the ethical considerations regarding the potential of the resulting embryos to develop into cloned embryos if implanted into a uterus. In addition, this technique has not been shown to work in humans. The initial failures and fraudulent reports of nuclear transfer in humans reduced enthusiasm for human applications , although it was recently reported that non-human primate embryonic stem cell lines were generated by SCNT of nuclei from adult skin fibroblasts .
Before SCNT-derived ESCs can be used as clinical therapy, careful assessment of quality of the lines must be determined. For example, some cell lines generated by SCNT have contained chromosomal translocations and it is not known whether these abnormalities originated from aneuploid embryos or if they occurred during ESC isolation and culture. In addition, the low efficiency of SCNT (0.7%) and the inadequate supply of human oocytes further hinder the therapeutic potential of this technique. Still, these studies renew the hope that embryonic stem cell lines could one day be generated from human cells to produce patient-specific stem cells with the potential to cure many human diseases that are currently untreatable.
Reprogrammed Somatic Cells
Recently, exciting reports of the successful transformation of adult cells into pluripotent stem cells through a type of genetic reprogramming have been published. Reprogramming is a technique that involves dedifferentiation of adult somatic cells to produce patient-specific pluripotent stem cells, eliminating the need to create embryos (see also Chapter 13 ). Cells generated by reprogramming would be genetically identical to the somatic cells (and thus, the patient who donated these cells) and would not be rejected. Yamanaka was the first to discover that mouse embryonic fibroblasts (MEFs) and adult mouse fibroblasts could be reprogrammed into an induced pluripotent state . These induced pluripotent stem (iPS) cells possessed the immortal growth characteristics of self-renewing ESCs, expressed genes specific for ESCs, and generated embryoid bodies in vitro and teratomas in vivo. When iPS cells were injected into mouse blastocysts, they contributed to a variety of cell types. However, although iPS cells selected in this way were pluripotent, they were not identical to ESCs. Unlike ESCs, chimeras made from iPS cells did not result in full-term pregnancies. Gene expression profiles of the iPS cells showed that they possessed a distinct gene expression signature that was different from that of ESCs. In addition, the epigenetic state of the iPS cells was somewhere between that found in somatic cells and that found in ESCs, suggesting that the reprogramming was incomplete.
These results were improved significantly by Wernig et al. in 2007 . In this study, DNA methylation, gene expression profiles and the chromatin state of the reprogrammed cells were similar to those of ESCs. Teratomas induced by these cells contained differentiated cell types representing all three embryonic germ layers. Most importantly, the reprogrammed cells from this experiment were able to form viable chimeras and contribute to the germ line like ESCs, suggesting that these iPS cells were completely reprogrammed.
It has recently been shown that reprogramming of human cells is possible . Yamanaka and co-workers generated human iPS cells that are similar to hESCs in terms of morphology, proliferation, gene expression, surface markers and teratoma formation . Thompson’s group showed that retroviral transduction of the stem cell markers OCT4 , SOX2 , NANOG and LIN28 could generate pluripotent stem cells . However, in both studies, the human iPS cells were similar but not identical to hESCs. Although reprogramming is an exciting phenomenon, our limited understanding of the mechanism underlying it currently limits the clinical applicability of the technique, but the future potential of reprogramming is quite exciting.
Placental and Amniotic Fluid Stem Cells
Recently, it has been shown that pluripotent cells may be derived from the amniotic fluid and placenta. Both amniotic fluid and placenta are known to contain multiple partially differentiated cell types derived from the developing fetus. Stem cell populations have been isolated from these sources. Called amniotic fluid and placental stem cells (AFPSCs), they express embryonic and adult stem cell markers . The undifferentiated stem cells expand extensively without a feeder cell layer and double every 36 h. Unlike hESCs, AFPSCs do not form tumors in vivo. Lines maintained for over 250 population doublings retained long telomeres and a normal complement of chromosomes. AFPSCs are broadly multipotent, and human lines can be induced to differentiate into cell types representing each embryonic germ layer, including cells of adipogenic, osteogenic, myogenic, endothelial, neuronal and hepatic lineages. Examples of differentiated cells derived from AFPSCs and displaying specialized functions include neuronal lineage secreting the neurotransmitter l -glutamate or expressing G-protein-gated inwardly rectifying potassium (GIRK) channels, hepatic lineage cells producing urea and osteogenic lineage cells forming tissue engineered bone. In this respect, they meet a commonly accepted criterion for pluripotent stem cells, without implying that they can generate every adult tissue. The cells could be obtained either from amniocentesis or chorionic villous sampling in the developing fetus, or from the placenta at the time of birth. They could be preserved for self-use and used without rejection, or they could be banked. A bank of 100,000 specimens could potentially supply 99% of the US population with a perfect genetic match for transplantation. Such a bank may be easier to create than with other cell sources, since there are approximately 4.5 million births per year in the USA .
Biomaterials
In the most common tissue engineering procedures, isolated cells are seeded onto a scaffold composed of an appropriate biomaterial. These biomaterials replicate the biological and mechanical function of the native ECM found in tissues in the body by serving as an artificial ECM. Biomaterials provide a three-dimensional space for the cells to develop into new tissues with appropriate structure and function (see also Chapter 16 ). They can also allow delivery of appropriate bioactive factors (e.g. cell adhesion peptides, growth factors) to the developing tissue to help regulate cellular function. As the majority of mammalian cell types is anchorage dependent and will die if no cell adhesion substrate is available, biomaterials provide this substrate that can deliver cells to specific sites in the body with high loading efficiency. Biomaterials can also provide mechanical support against in vivo forces so that the predefined three-dimensional structure of the engineered implant is maintained during tissue development.
The ideal biomaterial should be biodegradable and bioresorbable, and support the replacement of normal tissue without inducing inflammation. Incompatible materials are destined for an inflammatory or foreign-body response that eventually leads to rejection and/or necrosis. Degradation products, if produced, should be removed from the body via metabolic pathways at an adequate rate so that the concentration of these degradation products in the tissues remains at a tolerable level . The biomaterial should also provide an environment in which appropriate regulation of cell behavior (adhesion, proliferation, migration and differentiation) can occur. Cell behavior in the newly formed tissue has been shown to be regulated by multiple interactions of the cells with their microenvironment, including interactions with cell-adhesion ligands and with soluble growth factors. Since biomaterials provide temporary mechanical support while the cells undergo spatial reorganization into tissue, the properly chosen biomaterial should allow the engineered tissue to maintain sufficient mechanical integrity to support itself in early development, while in late development, it should begin to degrade so that it does not hinder further tissue growth .
In general, three classes of biomaterials have been used for engineering tissues: naturally derived materials (e.g. collagen and alginate), acellular tissue matrices (e.g. bladder submucosa and small intestinal submucosa) and synthetic polymers [e.g. polyglycolic acid (PGA), polylactic acid (PLA), and poly(lactic-co-glycolic acid) (PLGA)]. These classes of biomaterials have been tested with respect to their biocompatibility . Naturally derived materials and acellular tissue matrices have the potential advantage of biological recognition. However, synthetic polymers can be produced reproducibly on a large scale with controlled properties such as strength, degradation rate and microstructure, which would aid in the preparation of easily used, off-the-shelf scaffold material.
Naturally Derived Materials
Collagen is the most abundant and ubiquitous structural protein in the body, and may be readily purified from both animal and human tissues with an enzyme treatment and salt/acid extraction . Collagen implants, under normal conditions, degrade through a process involving phagocytosis of collagen fibrils in fibroblasts . This is followed by sequential attack by lysosomal enzymes including cathepsins B1 and D. Under inflammatory conditions, the implants can be rapidly degraded, largely by matrix metalloproteins (MMPs) and collagenases . However, the in vivo resorption rate of a collagen implant can be regulated by controlling the density of the implant and the extent of intermolecular cross-linking: the lower the density, the greater the space between collagen fibers and the larger the pores for cell infiltration, leading to a higher rate of implant degradation. Collagen contains cell adhesion domain sequences (e.g. RGD) that may assist in retaining the phenotype and activity of many types of cells, including fibroblasts and chondrocytes .
Alginate, a polysaccharide isolated from seaweed, has been used as an injectable cell delivery vehicle and a cell immobilization matrix owing to its gentle gelling properties in the presence of divalent ions such as calcium. Alginate is relatively biocompatible and is approved by the Food and Drug Administration (FDA) for human use as wound dressing material. Alginate is a family of copolymers of d -mannuronate and l -guluronate. The physical and mechanical properties of alginate gel are strongly correlated with the proportion and length of polygluronic block in the alginate chains .
Acellular Tissue Matrices
Acellular tissue matrices are collagen-rich matrices prepared by removing cellular components from tissues. The matrices are often prepared by mechanical and chemical manipulation of a segment of tissue . These matrices slowly degrade upon implantation, and are replaced and remodeled by ECM proteins synthesized and secreted by transplanted cells or on in-growing cells.
Synthetic Polymers
Polyesters of naturally occurring α-hydroxy acids, including PGA, PLA and PLGA, are widely used in tissue engineering. These polymers are FDA approved for a variety of applications, including sutures . The ester bonds in these polymers are hydrolytically labile, and they degrade by non-enzymatic hydrolysis. The degradation products of PGA, PLA and PLGA are non-toxic natural metabolites and are eventually eliminated from the body in the form of carbon dioxide and water . The degradation rate of these polymers can be tailored to the application by altering crystallinity, initial molecular weight and the copolymer ratio of lactic to glycolic acid. In general, the optimal degradation time ranges from several weeks to several years. Since these polymers are thermoplastics, they can be easily formed into a three-dimensional scaffold with a desired microstructure, gross shape and dimension by various techniques, including molding, extrusion, solvent casting , phase separation techniques and gas foaming techniques . Many applications in tissue engineering often require a scaffold with high porosity and ratio of surface area to volume. Other biodegradable synthetic polymers, including poly(anhydrides) and poly(ortho-esters), can also be used to fabricate scaffolds for tissue engineering with controlled properties .
Tissue Engineering of Specific Genitourinary Organs
Investigators around the world, including the author’s laboratory, have been working towards the development of several cell types, tissues and organs for clinical application. The following subsections will describe this research in detail.
Urethra
Various biomaterials without cells, such as PGA and acellular collagen-based matrices derived from decellularized small intestine and bladder, have been used in animal models for the regeneration of urethral tissue . Some of these biomaterials, like acellular collagen matrices derived from bladder submucosa, have also been seeded with autologous cells for urethral reconstruction. The author’s laboratory has been able to replace tubularized urethral segments with cell-seeded collagen matrices .
Acellular collagen matrices derived from bladder submucosa by this laboratory have been used experimentally and clinically. In animal studies, segments of the urethra were resected and replaced with acellular matrix grafts in an onlay fashion. Histological examination showed complete epithelialization and progressive vessel and muscle infiltration, and the animals were able to void through the neourethras . These results were confirmed in a clinical study of patients with hypospadias and urethral stricture disease . Decellularized cadaveric bladder submucosa was used as an onlay matrix for urethral repair in these patients. Patent, functional neourethras were noted in these patients with up to a 7-year follow-up ( Fig. 24.2 ). The use of an off-the-shelf matrix appears to be beneficial for patients with abnormal urethral conditions and obviates the need for obtaining autologous grafts, thus decreasing operative time and eliminating donor site morbidity.
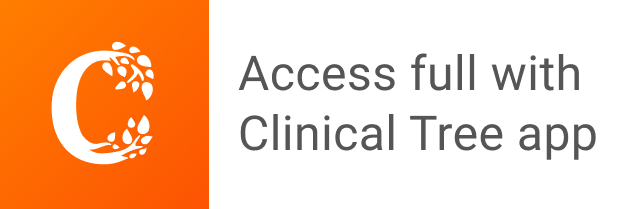