The renin-angiotensin system (RAS) is a master regulator of blood pressure and fluid homeostasis. This system is a multi-enzymatic cascade in which angiotensinogen, the major substrate, is processed in a two-step reaction by renin and angiotensin-converting enzyme (ACE), resulting in the sequential generation of angiotensins I and II. In recent years, several new enzymes, peptides, and receptors in this system have been identified, manifesting a complexity that was previously unappreciated. Although appropriate activation of the RAS is vital for preventing circulatory collapse and maintaining intravascular fluid balance, dysregulation and/or persistent RAS activation can lead to inappropriate blood pressure elevation, target organ damage, and even reduced survival. Accordingly, pharmacological agents that inhibit the synthesis or activity of angiotensin II are effective and widely used anti-hypertensive agents that can ameliorate morbidity and mortality in cardiovascular diseases including congestive heart failure and slow the progression of a wide range of progressive kidney diseases including diabetic nephropathy. Angiotensin receptor blockers (ARBs), which block type 1 (AT1) receptors, are similarly effective for treating these disorders. Below, we provide a comprehensive overview of the physiology of the RAS with an emphasis on actions impacting the kidney.
Keywords
Renin, Angiotensins, Angiotensin, receptors, Angiotensinogen, Hypertension, Diabetic nephropathy
Highly conserved through phylogeny, the renin– angiotensin system (RAS) is an essential regulator of blood pressure and fluid balance. This biological system is a multi-enzymatic cascade in which angiotensinogen, its major substrate, is processed in a two-step reaction by renin- and angiotensin-converting enzyme (ACE), resulting in the sequential generation of angiotensin I and angiotensin II. Along with its importance in maintaining normal circulatory homeostasis, abnormal activation of the RAS can contribute to the development of hypertension and target organ damage.
The importance of the RAS in clinical medicine is highlighted by two sets of observations. First are associations between polymorphisms of genes encoding RAS components and cardiovascular disease. Second, and perhaps more compelling, is the impressive efficacy of pharmacological agents that inhibit the synthesis or activity of angiotensin II. For example, angiotensin converting enzyme (ACE) inhibitors are very effective and well-tolerated anti-hypertensive agents. Along with their ability to lower blood pressure, these agents also effectively prevent or ameliorate morbidity and mortality associated with cardiovascular diseases. In this regard, large clinical trials have demonstrated that ACE inhibitors improve survival in patients with congestive heart failure, and in patients with risk factors for coronary artery disease. They also slow the progression of a variety of kidney diseases, including diabetic nephropathy. Angiotensin receptor blockers (ARBs), which block AT 1 receptors, are similarly effective for treating these disorders. The purpose of this chapter is to provide an overview of the major physiological features of the RAS, focusing on its role in the kidney.
The Components of the renin–angiotensin System
Renin
The aspartyl protease renin was first isolated from the kidney by Tigerstedt more than a century ago. Renin is synthesized as a precursor protein, pro-renin, containing an additional 43 amino acids at the N-terminus that block the enzyme’s active site. Active renin is generated by removal of this N-terminal peptide fragment, presumably by proteases in the juxtaglomerular cells of the kidney. Whether intact pro-renin has a distinct physiological role remains to be determined; however, there is accumulating evidence suggesting specific contributions of the pro-renin molecule in some normal and disease states.
Active renin specifically cleaves the 10 amino acids from the N-terminus of angiotensinogen to form angiotensin I. A substantial excess of angiotensinogen is present in serum, and ACE is ubiquitous in the endothelium and plasma. Accordingly, in the bloodstream, the amount of renin is the rate-limiting step determining the level of angiotensin II, and thus the activity of the system. The primary source of renin in the circulation is the kidney, where its expression and secretion are tightly regulated at the juxtaglomerular apparatus by two distinct mechanisms: a renal baroreceptor and sodium chloride delivery to the macula densa. Through these sensing mechanisms, levels of renin in plasma can be incrementally titrated in response to changes in blood pressure and salt balance. These regulatory principles provide a basis for many of the physiological characteristics of the RAS, and regulation of renin release in the kidney will be discussed in detail below.
In addition to its protease activity, renin may also bind specifically to other proteins or putative receptors. This binding may induce physiologically significant intracellular signaling. It has been suggested that the mannose-6-phosphate receptor (M6P-R), also known as insulin-like growth factor II receptor, binds renin and pro-renin, leading to internalization and degradation. Nguyen et al. reported cloning a receptor from human kidney expression library that binds renin and pro-renin specifically and with high affinity, termed the (pro)renin receptor (PRR). Binding of PRR causes a conformational change of renin that leads to increased renin catalytic activity. Similarly, binding of PRR to pro-renin causes a conformational change, resulting in an ezymatically active pro-renin without the requirement for cleavage of the pro-segment. Furthermore, binding of renin to this receptor induces a rapid and sustained activation of ERK1/ERK2, without affecting concentrations of calcium or cAMP. It has been suggested that it may mediate angiotensin II-independent effects of renin, and might also indicate a functional role of pro-renin. Although the physiological significance of PRR remains unclear, recent studies suggest PRR may have a role in blood pressure regulation. Transgenic rats overexpressing human PRR selectively in vascular smooth muscle cells display elevated blood pressure and heart rate. In addition, human PRR gene polymorphism has been shown to be associated with ambulatory blood pressure in Japanese men. PRR may also play a role in development. Deletion of PRR in mice results in early embryonic lethality. In a family with X-linked mental retardation and epilepsy, linkage analysis identified an exonic splice enhancer in the PRR gene as the only mutation, and this resulted in the loss of the capacity of renin to phosphorylate ERK1/2.
The pro-renin receptor appears to have other functions independent of the renin–angiotensin system. For example, it is found as part of a complex required for the normal function of V-ATPase in several cell lineages, including cardiac myocytes. The pro-renin receptor acts as an adaptor between the Wnt receptor and V-ATPase in a Wnt/β-catenin signaling complex required for normal CNS development. Thus, deletion of the pro-renin receptor gene causes a lethal phenotype at a very early embryonic stage, which contrasts significantly with the phenotype of renin knockouts, indicating important functions of the receptor that are independent of its actions in the RAS. Two groups have recently described studies of mouse lines in which the pro-renin receptor was deleted specifically from podocytes. In both cases, there was a similar, dramatic phenotype characterized by disruption of the glomerular filtration barrier, with marked proteinuria and abnormal podocyte structure, perhaps due to dysregulated autophagy. Thus, while this molecule appears to play a critical role in the kidney, much remains to be learned about its functions in normal kidney physiology and disease, including the extent to which these functions are influenced by renin or pro-renin binding.
Angiotensinogen
Angiotensinogen, the substrate for renin, is the source of all angiotensin peptides. Angiotensinogen in the circulation is derived primarily from synthesis in the liver. In humans, plasma concentrations of angiotensinogen are typically near the Km for renin, so that changes in plasma concentration may influence the rate of angiotensin I generation at any given level of renin. In human hypertensive siblings, Jeunemaitre et al. showed that a specific variant of the human angiotensinogen gene, M235T, was linked to hypertension, and was also associated with a modestly elevated plasma angiotensinogen concentration, about 120% of normal. They proposed that this variant of the AGT gene leads to an increase in plasma angiotensinogen levels and thereby eventually to increased blood pressure. However, because amino acid 235 is in a non-conserved portion of the angiotensinogen protein and variation of this amino acid does not affect protein stability, a mechanism to explain the physiological consequences of the mutation was not clear. An apparent explanation came later, when the M235T variant was found to be in linkage disequilibrium with another variant in the 5′ untranslated region of the AGT gene. This second variant, a single nucleotide substitution in the promoter of the AGT gene, was associated with increased transcriptional activity of the gene. Higher levels of AGT mRNA were found in patients carrying the variant allele. The causal capacity of alterations in plasma angiotensinogen level to affect blood pressure was demonstrated in studies of mice engineered to carry from 0 to 4 copies of the AGT gene. In these animals, there was a positive correlation between the number of AGT gene copies, plasma levels of angiotensinogen, and blood pressure.
In addition to its synthesis by the liver, angiotensinogen is also produced by other tissues including the brain, the immune system, and the kidney. In the kidney, synthesis of angiotensinogen in proximal tubules has been well-documented, and proximal tubule synthesis may be regulated in part by the end-product, angiotensin II. Along with angiotensinogen, the kidney expresses all of the other components of the RAS. Accordingly, it has been suggested that regulation and functioning of autonomous “tissue” renin–angiotensin systems in the kidney, as well as other organs, may contribute to the physiological functions of the system, especially in disease states. This hypothesis has been used to explain additional complexity of the system, whereby the apparent activity of the RAS is not reflected by measured plasma levels of it major components. For example, in the broad population of patients with hypertension, diabetes, and cardiovascular disease, pharmacological antagonists of the RAS lower blood pressure and prevent end-organ damage, even in the absence of overt elevation of plasma renin levels. However, the precise nature and physiological contributions of these tissue systems has been difficult to define experimentally.
Angiotensin Converting Enzyme
Angiotensin converting enzyme (ACE) is a carboxypeptidase that generates the vasoactive peptide angiotensin II by cleaving two amino acids from the c-terminus of the inactive precursor angiotensin I. There are two distinct forms of ACE, somatic and testicular, both generated by alternative splicing of a single gene. Somatic ACE is expressed as an ectoenzyme on the surface of endothelial cells throughout the body, and is particularly abundant in lung, intestine, choroid plexus, placenta, and on brush border membranes in the kidney. A soluble form of ACE that circulates in plasma is formed by enzymatic cleavage of tissue-bound ACE at its transmembrane domain. As with other components of the RAS, molecular variants of ACE have been proposed as candidate genes in hypertension, cardiovascular, and kidney diseases. Insertion (I) and deletion (D) polymorphisms of the human ACE gene are common, and have been associated with altered levels of ACE in plasma. In some cohorts, but not others, these ACE gene variants have been linked to differing susceptibilities to hypertension, cardiovascular, and renal diseases.
In addition to angiotensin I, other biologically active peptides are substrates for ACE. Perhaps the most important of these is bradykinin. ACE degrades bradykinin into an inactive peptide, representing a significant biological pathway for bradykinin metabolism in vivo ; in older literature, ACE was referred to as kininase II. Since bradykinin has vasodilator and natriuretic properties, it has been suggested that one mechanism of blood pressure reduction with ACE inhibition is blockade of this kininase activity. This was clearly demonstrated by Brown and associates, who showed that the anti-hypertensive efficacy of ACE inhibitors is attenuated by simultaneous administration of a bradykinin receptor antagonist.
Using genome-based strategies, homologs of ACE have been identified. One of these, ACE2, exhibits more than 40% identity at the protein level with the catalytic domain of ACE. Similar to ACE, ACE2 is expressed on the surface of certain endothelial cell populations. However, compared to the ubiquitous distribution of ACE, the expression pattern of ACE2 is more limited, with most abundant expression in kidney followed by heart and testis. Their substrate specificities also differ; ACE2 hydrolyzes angiotensin II with high efficiency, but has much lower activity against angiotensin I. Hydrolysis of angiotensin II by ACE2 generates another peptide with putative biological actions: angiotensin 1-7. Accumulating evidence indicates that this peptide causes vasodilation, natriuresis, and may promote reduced blood pressures via the Mas receptor. It has been further suggested that ACE2 may be a major pathway for synthesis of angiotensin 1-7. Thus, the functions of ACE2 may be determined by its distinct actions to metabolize angiotensin II and to generate angiotensin 1-7.
Although the precise physiological role of ACE2 is not clear, it was originally identified and cloned from a cDNA library prepared from ventricular tissue of a patient with heart failure. Initial studies in ACE2-deficient mice have suggested a role for ACE2 in cardiac function and in blood pressure regulation. More recent work by many groups has demonstrated roles for ACE2 in renal diseases, such as diabetic and non-diabetic kidney disease, and hypertension in both experimental models and human cohorts.
A third member of the ACE gene family, collectrin, was identified as a gene that is upregulated in the sub-total nephrectomy model of chronic kidney disease. Collectrin is highly homologous to the transmembrane portion of ACE2, but lacks the carboxypeptidase domain. Its physiological functions are emerging and appear to regulate amino acid transport by the kidney.
Angiotensin Receptors
The biological actions of angiotensin II are mediated by cell surface receptors that belong to the large family of 7 transmembrane receptors. The angiotensin receptors can be divided into two pharmacological classes, type 1 (AT 1 ) and type 2 (AT 2 ), based on their different affinities for various non-peptide antagonists ( Figure 15.1 ). Studies using these antagonists suggested that most of the classically recognized functions of the RAS are mediated by AT 1 receptors. Gene targeting studies have confirmed these conclusions.

AT 1 receptors from a number of species have been cloned and two subtypes, designated AT 1A and AT 1B , have been identified in rat and mouse. In the classical view, AT 1 receptors signal through G αq -linked signaling pathways involving phospholipase C, IP3, and increases in intracellular calcium. However, the AT 1 receptor has also been linked to JAK/STAT activation, as well as β-arrestin-dependent pathways linked to ERK activation. In addition, recent studies have shown that the AT1 receptor has the capacity to transactivate the EGF receptor, which may be independent of ligand. This pathway may contribute to chronic kidney injury.
The murine AT 1 receptors are products of separate genes and share substantial sequence homology. AT 1A receptors predominate in most organs, except the adrenal gland and regions of the CNS, where AT 1B expression may be more prominent. A single report has suggested that AT 1B receptors might also exist in man, but this has not been confirmed in the unpublished work of several independent groups, and the consensus view is that there is no human counterpart to the murine AT 1B receptor. Thus, the AT 1A receptor is considered the closest murine homolog to the single human AT 1 receptor.
The binding signatures of the AT 1A and AT 1B receptors are virtually identical, and it was difficult to discriminate their in vivo functions pharmacologically. Experiments using gene targeting have provided insights into the discrete functions of the two AT 1 receptor genes. Although the AT 1B receptor has a unique role to mediate thirst responses in the CNS, AT 1A receptors have the predominant role in determining the level of blood pressure, and in mediating vasoconstrictor responses. The phenotype of markedly reduced blood pressures and profound sodium sensitivity in mice lacking the AT 1A receptor underscores its importance in blood pressure control.
Pharmacological and genetic studies have confirmed that virtually all of the classically recognized functions of the RAS are mediated by AT 1 receptors. Until recently, little was known about the physiological role of AT 2 receptors. AT 2 receptors are found in abundance during fetal development, but their expression generally falls after birth. However, persistent AT 2 receptor expression can be detected in several adult tissues including the kidney, adrenal gland and the brain, and absolute levels of AT 2 receptor expression may be modulated by angiotensin II and certain growth factors. AT 2 receptors appear to signal by coupling to G αi2 and G αi3 proteins. Using site-directed mutagenesis, the intermediate portion of the third intracellular loop of the AT 2 receptor was found to be necessary for normal receptor signaling. Moreover, it has been suggested that activation of AT 2 receptors stimulates bradykinin, nitric oxide, and guanosine cyclic 3′,5′-monophosphate (cGMP), and these pathways may mediate actions of the receptor to promote natriuresis and blood pressure lowering. Finally, there is also evidence to support HETEs as second messengers for AT 2 receptors in the kidney, leading to ERK1/2 phosphorylation.
Targeted disruption of the mouse Agtr2 gene did not cause a dramatically abnormal phenotype. These animals clearly manifest increased sensitivity to the pressor actions of angiotensin II. One of the AT 2 deficient lines manifested increased baseline blood pressure and heart rate. Interestingly, behavioral changes were also observed in AT 2 -deficient mice. They had decreased spontaneous movements and rearing activity, and impaired drinking response to water deprivation. Transgenic mice that overexpress the AT 2 receptor gene under control of a cardiac-specific promoter have decreased sensitivity to AT 1 -mediated pressor and chronotropic actions. Moreover, the pressor actions of angiotensin II are significantly attenuated in these transgenic mice. This attenuation was completely reversed following pretreatment with a specific AT 2 receptor antagonist. Taken together, these data suggest that a primary function of the AT 2 receptor may be to negatively modulate the actions of the AT 1 receptor. Along similar lines, the recently described non-peptide agonist, compound 21, has been used to uncover additional functions of the AT 2 receptor, which appear quite diverse. Studies with this agonist regarding blood pressure have been variable, but it appears to have minimal effect on blood pressure in normal situations; the potential to affect blood pressure in disease states may be different.
Aldosterone
Aldosterone is a steroid hormone synthesized in the zona glomerulosa (ZG) of the adrenal gland. The two dominant regulators of aldosterone synthesis and release are angiotensin II and the level of serum potassium. The RAS-dependent component of aldosterone regulation is triggered by binding of angiotensin II to AT 1 receptors in the ZG. Stimulation of aldosterone release by angiotensin II contributes to enhanced sodium reabsorption and anti-natriuresis. Independently of angiotensin II, hyperkalemia can control the release of aldosterone through a process that involves the membrane depolarization of ZG cells. In addition, adrenocorticotropic hormone (ACTH) can stimulate aldosterone via its G-protein coupled receptor. Elevations in ACTH influence aldosterone production only during short-term stress, as this response is attenuated with persistent exposure to ACTH. In contrast, angiotensin II and potassium can both exert a chronic, sustained stimulation of aldosterone generation by the zona glomerulosa.
The classically recognized effects of aldosterone to influence sodium handling in the distal nephron are mediated by aldosterone binding to the mineralocorticoid receptor (MR). The MR is a 107 kD protein that acts as a transcription factor to regulate gene expression in target tissues. The molecular mechanisms used by the MR to drive epithelial sodium channel (ENaC) function in the collecting tubule have been reviewed recently. Cortisol actually exhibits a higher affinity for the mineralocorticoid receptor than aldosterone, but locally expressed 11ß-hydroxysteroid dehydrogenase type 2 “protects” the MR by converting cortisol to cortisone, which does not activate the MR. The binding of aldosterone to the MR in the principal cell of the collecting tubule epithelium induces transcription of the α-subunit, the multimeric coupling of the α-, β-, and γ-subunits of the EnaC, and the translocation of the ENaC complex to the luminal surface of the tubule. Aldosterone-induced expression of the αENaC-subunit, in particular, follows a diurnal variation pattern that depends on the circadian transcription factor Period1.
Aldosterone stimulates ENaC transcription and activity largely through the upregulation of serum- and glucocorticoid-regulated kinase 1 (sgk1). At the transcriptional level, Sgk1 phosphorylates ALL1-fused gene from chromosome 9 (Af9), which in turn blocks the repressor effects of the histone H3 Lys79 methyltransferase disruptor of telomeric silencing alternative splice variant a (Dot1a) on αENaC gene transcription. At the post-translational level, Sgk1 phosphorylates Nedd4-2, causing ENaC proteins to remain in the apical membrane of the principal cell. Once inserted into the luminal membrane of the principal cell, ENaC permits cellular uptake of intraluminal sodium, generating an electronegative potential in the distal tubular lumen which favors secretion of potassium from the principal cell into the urinary filtrate via the renal outer medullary potassium channel (ROMK). Sgk1 may also phosphorylate ROMK, similarly increasing its apical density, further facilitating the kaliuresis induced by aldosterone. In addition, aldosterone appears to directly increase ROMK expression. Finally, aldosterone modulates sodium transport in the distal nephron independently of ENaC by enhancing expression and activity of the thiazide-sensitive Na-Cl co-transporter (NCC). Sgk-1 mediates this effect by phosphorylating serine/threonine kinase with-no-lysine 4 (WNK4), thereby diminishing the inhibitory effects of WNK4 on NCC activity. Through these pathways, the MR regulates sodium and potassium transport within the mineralocorticoid-responsive segments of the distal nephron.
Recent human phenotyping studies and animal studies using gene-targeting strategies have confirmed the contribution of the MR to tubular function and salt balance. For example, in humans with a mutation leading to a constitutively active MR, early onset hypertension develops, whereas heterozygosity for an inactivating mutation of the MR leads to salt-wasting, hypotension, metabolic acidosis, and hyperkalemia. Mice genetically deficient for the MR similarly develop severe salt-wasting that leads to neonatal death, whereas mice with genetic deletion of the MR restricted to the principal cell waste salt and lose body weight only when exposed to a low-sodium diet, suggesting that at baseline the late distal convoluted tubule and early connecting tubule may be able to compensate for a lack of ENaC activity in the distal nephron. Alternatively, the discrepancy in phenotypes between the global and conditional knockout mice may be due to discrete functions of aldosterone to modulate solute transport in the proximal tubule and/or medullary thick ascending limb. Mutations that activate the ENaC may cause hypertension, whereas global inactivation of the subunits of the ENaC in mice causes sodium-wasting, potassium retention, and early mortality, and in humans pseudohypoaldosteronism type 1 with severe salt-wasting. In contrast, inactivation of the α-ENaC gene only in the collecting duct does not impair sodium and potassium balance, again indicating that the regulation by aldosterone of ENaC in the latter regions of the distal convoluted tubule and/or the connecting tubule may also contribute to sodium and fluid homeostasis.
In addition to its physiologic effects on renal solute handling, aldosterone has the capacity to mediate direct cellular injury in the kidney. In this regard, pathologic functions of aldosterone in non-tubular renal compartments become increasingly relevant. For example, aldosterone impairs vascular reactivity by diminishing expression of glucose-6-phosphate in the endothelium and mediates direct vascular injury via a placental growth factor-dependent pathway. In mesangial cells, aldosterone activates sgk-1, NF-kB, and MAP kinases, leading to cellular proliferation, generation of oxidative stress, and connective tissue growth factor expression. Emerging evidence suggest aldosterone may also promote oxidative stress and apoptosis directly within podocytes. Consistent with these pathologic effects of aldosterone in several cell lineages of the kidney glomerulus, human studies have now demonstrated a role for aldosterone blockade in ameliorating the progression of proteinuric kidney disease.
Integrated Actions of the RAS in the Kidney
The important role of the kidney in regulation of blood pressure has been long recognized, and the relationship between alterations in systemic blood pressure and changes in renal sodium excretion is well-documented. For example, an elevation in perfusion pressure in the renal artery results in a rapid increase in sodium and water excretion by the kidney, so-called “pressure natriuresis”. Based on such observations, Guyton and co-workers suggested that whenever arterial pressure is elevated, activation of this pressure-natriuresis mechanism will cause sufficient excretion of sodium and water to return systemic pressures to normal. They further hypothesized that the substantial capacity for sodium excretion by the kidney provides a compensatory system of virtually infinite gain to oppose processes, including increases in peripheral vascular resistance, which would tend to increase blood pressure. It follows that defects in renal excretory function would therefore be a pre-requisite for sustaining a chronic increase in intra-arterial pressure.
The RAS has potent actions to modulate pressure-natriuresis relationships in the kidney and these actions shape the characteristics of RAS-dependent blood pressure regulation in normal physiology and in disease states. For example, as depicted in Figure 15.2 , chronic infusion of angiotensin II causes a shift of the pressure natriuresis curve to the right, suggesting that when the RAS is activated, higher pressures are required to excrete an equivalent sodium load ( Figure 15.2 ). Conversely, administration of ACE inhibitors or ARBs shifts the curve to the left, meaning that natriuresis is facilitated at lower levels of blood pressure ( Figure 15.2 ). The basic features of endogenous control of the RAS are consistent with these homeostatic functions. As shown in Figure 15.2 , the system is activated at low levels of salt intake, stimulating renal sodium reabsorption and conservation of body fluid volumes and blood pressure. In contrast, with high sodium intake, the system is suppressed, facilitating natriuresis.
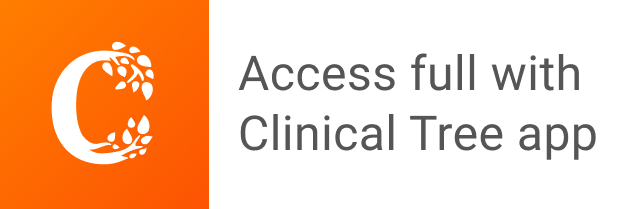