Chapter Outline
MAJOR ARTERIES AND VEINS, 83
Hydraulic Pressure Profile of the Renal Circulation, 85
TOTAL RENAL BLOOD FLOW , 85
INTRARENAL BLOOD FLOW DISTRIBUTION, 87
Vascular-Tubule Relations, 87
Cortical Blood Flow, 87
Peritubular Capillary Dynamics, 89
Medullary Blood Flow, 90
Medullary Microcirculation, 90
Structure of the Glomerular Microcirculation, 92
DETERMINANTS OF GLOMERULAR ULTRAFILTRATION, 94
Hydraulic Pressures in the Glomerular Capillaries and Bowman’s Space, 94
Glomerular Capillary Hydraulic and Colloid Osmotic Pressure Profiles, 94
Determination of the Ultrafiltration Coefficient, 96
Selective Alterations in the Primary Determinants of Glomerular Ultrafiltration, 97
REGULATION OF RENAL HEMODYNAMICS AND GLOMERULAR FILTRATION, 98
Vasomotor Properties of the Renal Microcirculations, 98
Role of the Renin-Angiotensin System in the Control of Renal Blood Flow and Glomerular Filtration Rate, 99
Endothelial Factors in the Control of Renal Hemodynamics and Glomerular Filtration, 101
Renal Autoregulation, 104
NEURAL REGULATION OF GLOMERULAR FILTRATION RATE, 110
Under resting conditions, blood flow to the kidneys represents approximately 20% of cardiac output in humans even though these organs constitute less than 1% of body mass. This rate of blood flow, approximately 400 mL per 100 g of tissue per minute, is significantly greater than that observed in other vascular beds considered to be well perfused, such as heart, liver, and brain. From this enormous blood flow (1.0 to 1.2 L/minute), only a small quantity of urine is formed (1 mL/minute). Although the metabolic energy requirement of urine production is relatively high (approximately 10% of basal O 2 consumption), the renal arteriovenous O 2 difference reveals that blood flow far exceeds metabolic demands. In fact, the high rate of blood flow is essential to the process of urine formation as described later.
The kidney contains several distinct microvascular networks, including the glomerular microcirculation, the cortical peritubular microcirculation, and the unique microcirculations that nourish and drain the inner and outer medulla. In Chapter 2 the gross anatomy of the kidney and arrangement of tubular segments were described. In this chapter we consider the intrarenal organization of the discrete microcirculatory networks and regional renal blood flows as well as how this anatomy contributes to the physiologic factors that regulate renal blood flow (RBF) and glomerular filtration rate (GFR).
Major Arteries and Veins
Blood supply for each kidney is provided by a renal artery that branches directly from the abdominal aorta. The human renal artery typically branches into multiple segmental vessels at a point just before entry into the renal parenchyma ( Figure 3.1 ). Therefore, complete obstruction of an arterial segmental vessel results in ischemia and infarction of the tissue in its area of distribution. In fact, ligation of individual segmental arteries has frequently been performed in the rat to reduce renal mass and produce the remnant kidney model of chronic renal failure. Morphologic studies in this model reveal the presence of ischemic zones adjacent to the totally infarcted areas. These regions contain viable glomeruli that appear shrunken and crowded together, demonstrating that some portions of the renal cortex may have partial dual perfusion.

The anatomic distribution just described is most common; however, other patterns may occur. Not infrequently, secondary renal arteries may result from division of the renal artery at the aorta. These vessels, which most often supply the lower pole, may be the sole arterial supply of some part of the kidney. Such additional arteries are found in 20% to 30% of normal individuals.
Within the renal sinus of the human kidney, division of the segmental arteries gives rise to the interlobar arteries. These vessels, in turn, give rise to the arcuate arteries, whose several divisions lie at the border between the cortex and medulla (see Figure 3.1 ). From the arcuate arteries, the interlobular arteries branch more or less sharply, most often as a common trunk that divides two to five times as it extends toward the kidney surface ( Figure 3.2 ). Afferent arterioles leading to glomeruli arise from the smaller branches of the interlobular arteries ( Figure 3.3 ). Glomeruli are classified according to their position within the cortex as superficial (i.e., near the kidney surface), midcortical, or juxtamedullary (near the corticomedullary border). The capillary network of each glomerulus is connected to the postglomerular (peritubular) capillary circulation by way of the efferent arterioles. Both the nomenclatures and the patterns of the renal arterial system are similar in most of the mammals commonly used experimentally. For example, the main arterial branches that lie beside the medullary pyramid are called interlobar, even in animals such as rodents that have but a single lobe.


Hydraulic Pressure Profile of the Renal Circulation
The pressure drop between the systemic vasculature and the end of the interlobular artery in both the superficial and the juxtamedullary microvasculature can be as much as 25 mm Hg at normal perfusion pressures, with the majority of that pressure drop occurring along the interlobular arteries ( Figure 3.4 ). However, on the basis of studies of the vasculature of a unique set of juxtamedullary nephrons, most of the preglomerular pressure drop between the arcuate artery and the glomerulus occurs along the afferent arteriole. Approximately 70% of the postglomerular hydraulic pressure drop takes place along the efferent arterioles, with approximately 40% of the total postglomerular resistance accounted for by the early efferent arteriole (see Figure 3.4 ). Of note, studies of juxtamedullary nephrons perfused via the arcuate artery indicate that the very late portion of the afferent arteriole (last 50-150 µm) and the very early portion of the efferent arteriole (first 50-150 µm) provide a large portion of the total preganglionic and postglomerular resistance. Indeed, elegant work by Peti-Peterdi and associates using multiphoton imaging indicate the presence of an intraglomerular precapillary sphincter ( Figure 3.5 ).


Total Renal Blood Flow
Total RBF in humans typically exceeds 20% of the cardiac output, or about 1 to 1.2 L/min for a man. The classic method of determining total RBF is first to determine renal plasma flow using the “clearance” of an indicator substance from blood passing through the kidney and its subsequent appearance in the urine. The simple formula for the clearance of any substance is as follows:
C x = U x V/P x
If the substance is neither metabolized nor synthesized in the kidney then its rate of appearance in the urine equals its rate of extraction from the blood. The blood extraction rate is equal to the renal plasma flow rate multiplied by the difference between the arterial and renal venous plasma concentrations. This can be expressed mathematically as follows:
U x V = ( Art x − Vein x ) × RPF
RPF = U x V/ ( Art x − Vein x )
RBF can then be calculated by dividing RPF by the plasma fraction of whole blood (from the hematocrit, Hct):
RBF = RPF/ ( 1 − Hct )
Historically, RBF has been estimated from determinations of renal plasma flow using p -aminohippuric acid (PAH) as the indicator. This substance is both filtered at the glomerulus and actively secreted by the tubules, resulting in the renal extraction of 70% to 90% of PAH from the blood. Not all the PAH is removed from the kidney circulation because of flow through regions of the kidney (e.g., medulla) that do not perfuse proximal tubule segments where secretion occurs, incomplete removal of PAH in (some) cortical regions, and the presence of periglomerular shunts ( Figure 3.6 ). If the extraction is assumed to be equal to 100% (renal venous concentration equals zero) then the clearance of PAH, using equation 1 ((U PAH × V)/Art PAH ), provides a simple, noninvasive approximation of RPF. This approximation is often termed “effective” renal plasma flow (ERPF) and provides an estimate of RPF without the need for a renal venous blood sample. However, this estimate of renal plasma flow is much less accurate in renal disease because extraction is further reduced by damage to proximal tubule segments involved in PAH secretion. Figure 3.7 shows some typical values for ERPF and GFR in adult humans from a number of studies.


Micropuncture studies performed in vivo in experimental animals provide more accurate and detailed information about cortical blood flow but the medulla is less accessible to micropuncture, and thus the medullary blood flow has been less studied. For detailed discussion of historical methods of RBF measurements, the reader is referred to Dworkin and Brenner. Improved methods of RBF measurement have been introduced with laser Doppler flowmetry, video microscopy, and imaging techniques such as positron emission tomography (PET), high-speed computed tomography (CT), and magnetic resonance imaging (MRI). These methods have been especially useful in determining regional blood flow as discussed later.
Intrarenal Blood Flow Distribution
The cortex accounts for filtration and the majority of reabsorption, whereas the medulla’s primary function is maintenance of a hypertonic gradient and urine concentration. Therefore, RBF to these regions is differentially regulated in response to the differing demands of these two kidney regions. There are structural differences in vascular components of the cortex and the medulla that may account for differences in RBF, namely, the organization of the afferent and efferent arterioles of the cortical and juxtamedullary glomeruli. Studies conducted in rabbits demonstrated that cortical afferent arterioles have larger internal diameters than the efferent arterioles, whereas juxtamedullary afferent and efferent arterioles are significantly larger and the efferent arteriole is more muscular compared to the cortical arterioles. In addition, the cortical peritubular capillaries, derived from efferent arterioles of cortical glomeruli, are about half the size of the medullary vasa recta derived from efferent arterioles of the juxtamedullary glomeruli ( Figure 3.8 ). These features may partially explain the differential control of medullary and cortical blood flows. Additional factors include sympathetic nerve activity and hormonal influences.

Vascular-Tubule Relations
Cortical vascular-tubule relations have been described most completely in the canine kidney. These studies show that, except for convoluted tubule segments in the outermost region of the cortex, the efferent peritubular capillary network and the nephron arising from each glomerulus are often dissociated. In addition, even though the blood supply of many superficial proximal and distal convoluted tubules is derived from peritubular capillaries arising from the parent glomerulus of the same nephron ( Figure 3.9 ), the loops of Henle of such nephrons, descending in the medullary ray, are surrounded by blood vessels emerging from many midcortical glomeruli through efferent arterioles that extend directly into the ray. Nephrons originating from midcortical glomeruli have proximal and distal convoluted tubule segments lying close to the interlobular axis in the region above the glomerulus of origin. This region is perfused by capillary networks arising from the efferent arterioles of more superficial glomeruli. It is in the inner cortex, however, that this dissociation between individual tubules and the corresponding postglomerular capillary network is most apparent. The convoluted tubule segments of these nephrons lie above the glomeruli surrounded either by the dense network close to the interlobular vessels or by capillary networks arising from other inner cortical glomeruli.

Efferent vessel patterns and vascular-tubule relationships in the human kidney are similar to those in the dog kidney. In general, a close association between the initial portions of peritubular capillaries and early and late proximal tubule segments of the same glomerulus has been shown. However, this close association does not mean that each vessel adjacent to a given tubule necessarily arises from the same glomerulus. In fact, Briggs and Wright found that although superficial nephron segments and vessels arising from the same glomerulus are closely associated, each vessel may serve segments of more than one nephron.
Cortical Blood Flow
The majority of RBF perfuses the cortex. Vasoconstrictors such as angiotensin II, endothelin, and noradrenaline have much greater effects on cortical blood flow than on medullary blood flow, whereas vasodilators such as bradykinin and nitric oxide tend to selectively increase medullary blood flow. There can be extensive redistribution of blood flow in the kidney under various conditions that may be important in physiologic and pathophysiologic conditions. Studies by Trueta of RBF distribution after hemorrhage were among the first performed. These studies indicated that during shock, RBF appeared to be shunted through the medulla. This phenomenon, observed more than 60 years ago in qualitative studies of the distribution of India ink and radiographic contrast media, was subsequently termed “cortical ischemia with maintained blood flow through the medulla.”
Peritubular Capillary Dynamics
The same Starling forces that control fluid movement across all capillary beds govern the rate of fluid movement across peritubular capillary walls. Owing to the relatively high resistance along the afferent and efferent arterioles, a large drop in hydraulic pressure occurs prior to the peritubular capillaries. In addition, as protein-free fluid is filtered out of the glomerular capillaries and into Bowman’s space, the oncotic pressure of blood flowing into the peritubular capillaries increases because of “trapped” plasma proteins. The sum of these forces favors fluid movement into the peritubular capillaries. Alterations in the net driving force for reabsorption (i.e., the balance between the transcapillary oncotic and hydraulic pressure gradients) have significant effects on net proximal reabsorption. The absolute amount of movement resulting from this driving force also depends on the peritubular capillary surface area available for fluid uptake and the hydraulic conductivity of the capillary wall. Values for the hydraulic conductivity of the peritubular capillaries are not as great as those for the glomerular capillaries, but this difference is offset by the much larger total surface area of the peritubular capillary network.
In the rat, it has been estimated that approximately 50% of the peritubular capillary surface is composed of fenestrated areas. Unlike the glomerular capillaries, peritubular capillary fenestrations are bridged by a thin diaphragm that is negatively charged. Beneath the fenestrae of the endothelial cells lies a basement membrane that completely surrounds the capillary. For the most part, peritubular capillaries are closely apposed to cortical tubules ( Figure 3.10 ), so that the extracellular space between the tubules and capillaries constitutes only about 5% of the cortical volume. The tubular epithelial cells are surrounded by the tubular basement membrane, which is distinct from and wider than the capillary basement membrane (see Figure 3.10 ). Numerous microfibrils connect the tubular and capillary basement membranes, a feature that may help limit expansion of the interstitium and maintain close contact between tubular epithelial cells and the peritubular capillaries during periods of high fluid flux. Thus, the pathway for fluid reabsorption from the tubular lumen to the peritubular capillary is composed, in series, of the epithelial cell, tubular basement membrane, a narrow interstitial region containing microfibrils, the capillary basement membrane, and the thin membrane bridging the endothelial fenestrae.

Like the endothelial cells, the basement membrane of the peritubular capillaries possesses anionic sites. The electronegative charge density of the peritubular capillary basement membrane is significantly greater than that observed in the unfenestrated capillaries of skeletal muscle and similar to that observed in the glomerular capillary bed. Although the function of the anionic sites in the peritubular capillaries is uncertain, it is likely, by analogy to the glomerulus, that they are an adaptation to compensate for the greater permeability of fenestrated capillaries, allowing free exchange of water and small molecules while restricting anionic plasma proteins to the circulation. In fact, some workers have reported that the renal peritubular capillaries are more permeable to both small and large molecules than are other beds. This conclusion is based on tracer studies in which the renal artery was clamped or the kidney removed before fixation. However, because normal plasma flow conditions appear necessary for the maintenance of the glomerular permeability barrier, it is possible that these high stop-flow peritubular permeabilities occur as an artifact of the unfavorable experimental conditions employed. Indeed, studies by Deen and associates indicate that, at least under free-flow conditions, the permeability of these vessels to dextrans and albumin is extremely low.
Because the peritubular capillaries that surround a given nephron are derived from many efferent vessels, regulatory processes related to capillary factors should not be viewed only as a mechanism for balancing filtration and reabsorption in a single nephron. Instead, assuming that capillary dynamics throughout the cortex are the same, we may consider that all tubule segments are surrounded by capillary vessels that are operating in a similar reabsorptive mode. Thus, the function of the cortex as a whole may reflect the average reabsorptive capacity of all cortical peritubular vessels.
Medullary Blood Flow
Medullary blood flow constitutes about 10% to 15% of total RBF and is derived from efferent arterioles of the juxtamedullary nephrons. Although these medullary flows are less than one fourth as high as cortical flows, medullary flow is still substantial. Thus, per gram of tissue, outer medullary flow exceeds that of liver, and inner medullary flow is comparable to that of resting muscle or brain. The fact that such large flows are compatible with the existence and maintenance of the inner medullary solute concentration gradient attests to the efficiency of countercurrent mechanisms in this region. The descending vasa recta have a continuous endothelium in which water moves across water channels and urea moves through endothelial carriers. The ascending vasa recta are fenestrated, with a high hydraulic conductivity and water movement likely governed by transcapillary hydraulic and oncotic pressure gradients. Medullary blood flow is highest under conditions of water diuresis and declines during antidiuresis. This decrease depends, at least in part, on a direct vasoconstrictive action of vasopressin on the medullary microcirculation. Vasodilatory factors act to preserve medullary blood flow and prevent ischemia. Acetylcholine, vasodilator prostaglandins, kinins, adenosine, atrial peptides, bradykinin, and nitric oxide increase medullary RBF. In contrast to their vasoconstrictor effects in the renal cortex, angiotensin II and endothelin increase medullary blood flow, effects mediated in part by vasodilatory prostaglandins, whereas vasopressin decreases medullary blood flow. Alterations in medullary blood flow may be a key determinant of medullary tonicity and, thereby, solute transport in the loops of Henle. In addition the medullary circulation may play an important role in the control of sodium excretion and blood pressure.
Medullary Microcirculation
Vascular Patterns
The precise location of the boundary between the renal cortex and medulla is difficult to discern because the medullary rays of the cortex merge imperceptibly with the medulla. In general, the arcuate arteries or the sites at which the interlobular arteries branch into arcuate arteries mark this boundary. When considering the medullary circulation, most studies focus on its relation to the countercurrent mechanism as facilitated by the parallel array of descending and ascending vasa recta. However, although this configuration is characteristic of the inner medulla, the medulla also contains an outer zone, which consists of two morphologically distinct regions, the outer and inner stripes of the outer medulla (see Figure 3.9 ). The boundary between the outer medulla and inner medulla is defined by the beginning of the thick ascending limbs of Henle (see Figure 3.9 ). In addition to the thick ascending limbs, the outer medulla contains descending straight segments of proximal tubules ( pars recta ), descending thin limbs, and collecting ducts. The nephron segments of the inner stripe of the outer medulla include thick ascending limbs, thin descending limbs, and collecting ducts. Each of these morphologically distinct medullary regions is supplied and drained by an independent, specific vascular system.
The blood supply of the medulla is derived entirely from the efferent arterioles of the juxtamedullary glomeruli (see Figure 3.9 ). Depending on the species and the method of evaluation, it has been estimated that from 7% to 18% of glomeruli give rise to efferents that ultimately supply the medulla. Efferent arterioles of juxtamedullary nephrons are larger in diameter and possess thicker endothelium and more prominent smooth muscle layers than arterioles originating from superficial glomeruli.
The vasculature of the outer medulla displays both vertical and lateral heterogeneity, but in general, both the outer and inner stripes contain two distinct circulatory regions: the vascular bundles, formed by the coalescence of the descending and ascending vasa recta, and the interbundle capillary plexus. Vascular bundles of descending and ascending vasa recta arise from the efferent arterioles of juxtamedullary glomeruli and descend through the outer stripe of the outer medulla to supply the inner stripe of the outer medulla and the inner medulla ( Figure 3.11 ). Within the outer stripe, the descending vasa recta also give rise, via small side branches, to a complex capillary plexus. Early studies suggested that this capillary network was limited and, therefore, not the main blood supply to this region. Instead, it was thought that nutrient flow was provided by the ascending vasa recta rising from the inner stripe. This notion was further suggested by the large area of contact between ascending vasa recta and the descending proximal straight tubules within this zone.

The outer medulla includes the metabolically active thick ascending limbs. Nutrients and O 2 to this energy-demanding tissue to the inner stripe are delivered by a dense capillary plexus arising from a few descending vasa recta at the periphery of the bundles. Approximately 10% to 15% of total RBF is directed to the medulla, and of this amount, probably the largest portion perfuses this inner stripe capillary plexus. The smooth muscle cells of the descending vasa recta are replaced by pericytes surrounding the endothelium with subsequent loss of the pericytes and transformation into medullary capillaries accompanied by endothelial fenestrations.
The rich capillary network of the inner stripe drains into numerous veins, which for the most part do not join the vascular bundles but ascend directly to the outer stripe. These veins subsequently rise to the cortical-medullary junction, and the majority join with cortical veins at the level of the inner cortex. A minority of the veins may extend within the medullary rays to regions near the kidney surface. Thus, the capillary network of the inner stripe makes no contact with the vessels draining the inner medulla.
The inner medulla contains thin descending and thin ascending limbs of Henle, together with collecting ducts (see Figure 3.9 ). Within this region, the straight, unbranching vasa recta descend in bundles, with individual vessels leaving at every level to divide into a simple capillary network characterized by elongated links (see Figures 3.6 and 3.9 ). These capillaries converge to form the venous vasa recta. Within the inner medulla, the descending and ascending vascular pathways remain in close apposition, although distinct vascular regions can no longer be clearly discerned. The venous vasa recta rise toward the outer medulla in parallel with the supply vessels to join the vascular bundles. Thus, the outer medullary vascular bundles include both supplying and draining vessels of the inner medulla. Within the outer stripe of the outer medulla, the vascular bundles spread out and traverse the outer stripe as wide, tortuous channels that lie in close apposition to the tubules, eventually emptying into arcuate or deep interlobular veins. The venous pathways within the bundles are both larger and more numerous than the arterial vessels, suggesting lower flow velocities in the ascending (venous) than in the descending (arterial) direction. The close apposition of the arterial and venous pathways within the vascular bundles is important for maintaining the hypertonicity of the inner medulla.
There are important differences in the structures of the ascending and descending vasa recta. The descending vasa recta possess a contractile layer composed of smooth muscle cells in the early segments that evolve into pericytes by the more distal portions of the vessels. Immunohistochemical studies demonstrate that these pericytes contain smooth muscle α-actin, suggesting that they may serve as contractile elements and participate in the regulation of medullary blood flow as well as vascular-tubular crosstalk. Each of these vessels also displays a continuous endothelium that persists until the hairpin turn is reached and the vessels divide to form the medullary capillaries. In contrast, ascending vasa recta, like true capillaries, lack a contractile layer and are characterized by a highly fenestrated endothelium.
Vascular-Tubule Relations
The mechanism of urine concentration requires coordinated function of the vascular and tubule components of the medulla. In species capable of marked concentrating ability, medullary vascular-tubule relations show a high degree of organization favoring particular exchange processes by the juxtaposition of specific tubule segments and blood vessels. In addition to anatomic proximity, the absolute magnitude of these exchanges is greatly influenced by the permeability characteristics of the structures involved, which may vary significantly among species.
Most of our detailed knowledge of vascular-tubule relations within the medulla is based on histologic studies of rodent species. As already discussed, the inner stripe of the outer medulla contains two distinct territories, the vascular bundles and the interbundle regions (see Figures 3.6 and 3.11 ). In most mammals, the vascular bundles contain only closely juxtaposed descending and ascending vasa recta running in parallel. The tubule structures of the inner stripe, including thin descending limbs, thick ascending limbs, and collecting ducts, are found in the interbundle regions and are supplied by the dense capillary bed described earlier. Commonly, the interbundle territory is organized with the long loops of the juxtamedullary nephrons lying closest to the vascular bundles. The shorter loops arising from superficial glomeruli are more peripheral and therefore closer to the collecting ducts. The vascular bundles themselves contain no tubule structures.
Medullary Capillary Dynamics
The functional role of the medullary peritubular vasculature is basically the same as that of cortical peritubular vessels. These capillaries supply the metabolic needs of nearby tissues and are responsible for the uptake and removal of water extracted from collecting ducts during the process of urine concentration. However, because the urinary concentration process is based on the maintenance of a hypertonic interstitium, the countercurrent arrangement of medullary blood flow plays a vital role in maintaining the medullary solute gradient through passive countercurrent exchange.
Structure of the Glomerular Microcirculation
The glomerulus and glomerular filtration are discussed in detail later in this chapter. Figure 3.12 shows a scanning electron micrograph of a resin-filled cast of a glomerulus with the afferent arteriole branching from the interlobular artery, the many loops of the glomerular capillaries, and the efferent arteriole emerging from the glomerular tuft. Elger and coworkers published a detailed ultrastructural analysis of the vascular pole of the renal glomerulus. They described significant differences in the structure and branching patterns of the afferent and efferent arterioles as these vessels enter and exit the tuft. Afferent arterioles lose their internal elastic layer and smooth muscle cell layer prior to entering the glomerular tuft. Smooth muscle cells are replaced by renin-positive, myosin-negative granular cells that are in close contact with the extraglomerular mesangium. As described by Eljer and coworkers, upon entering Bowman’s space, afferent arterioles branch immediately and are distributed along the surface of the glomerular tuft. These primary branches have wide lumens and immediately acquire features of glomerular capillaries, including a fenestrated endothelium, characteristic glomerular basement membrane, and epithelial foot processes. In contrast, the efferent arteriole arises deep within the tuft, from the convergence of capillaries arising from multiple lobules. Additional tributaries join the arteriole as it travels toward the vascular pole. The structure of the capillary wall begins to change even before the vessels coalesce to form the efferent arteriole, losing fenestrae progressively until a smooth epithelial lining is formed. At the arteriole’s terminal portion within the tuft, endothelial cells may bulge into the lumen, reducing its internal diameter.

Typically, the diameter of the efferent arteriole within the tuft is significantly less than that of the afferent arteriole in the outer cortex. Efferent arterioles of the juxtamedullary nephrons, however, may be larger in diameter than the afferent arterioles and have thicker walls. Moreover, both the efferent and afferent arterioles of the juxtamedullary nephrons appear to be larger in diameter than those of more superficial nephrons (see Figure 3.8 ). Efferent arterioles acquire a smooth muscle cell layer, which is observed distal to the entry point of the final capillary. The efferent arteriole is also in close contact with the glomerular mesangium as it forms inside the tuft and with the extraglomerular mesangium as it exits the tuft. This precise and close anatomic relationship between the afferent and efferent arterioles and mesangium is consistent with the presence of the intraglomerular signaling system that participates in the regulation of blood flow and filtration rate.
The appearance of the vascular pathways within the glomerulus may change under different physiologic conditions. The glomerular mesangium has been shown to contain contractile elements ( Figure 3.13 ) and to exhibit contractile activity when exposed to angiotensin II. Mesangial cells, which possess specific receptors for angiotensin II, undergo contraction when exposed to this peptide in vitro. Three-dimensional reconstruction of the entire mesangium in the rat suggests that approximately 15% of capillary loops may be entirely enclosed within armlike extensions of mesangial cells that are anchored to the extracellular matrix. Contraction of these cells might alter local blood flow and filtration rate as well as the intraglomerular distribution of blood flow and total filtration surface area. Many hormones and other vasoactive substances capable of altering glomerular filtration may bring about this adjustment, in part, by altering the state of contraction of mesangial cells.

In the outermost, or subcapsular, region of the cortex, the efferent arterioles give rise to a dense capillary network that surrounds the convoluted tubule segments arising from the superficial glomeruli (see Figure 3.9 ). There is evidence suggesting that this arrangement is of great importance for reabsorption of water and electrolytes in proximal tubule segments of superficial nephrons. In contrast, the efferent arterioles originating from the comparatively fewer juxtamedullary glomeruli extend into the medulla and give rise to the medullary microcirculatory patterns: an intricate capillary network in the outer medulla and long, unbranched capillary loops, the vasa recta, in the inner medulla. The arrangement of the medullary microcirculation plays an important role in the process of concentration of urine.
Venous drainage of the most superficial cortex is by way of superficial cortical veins. In middle and inner cortex, venous drainage is achieved mainly by the interlobular veins. The dense peritubular capillary network surrounding the interlobular vessels drains directly into the interlobular veins through multiple connections, whereas the less dense, long-meshed network of the medullary rays appears to anastomose with the interlobular network and thus drain laterally. The medullary circulation also shows two different types of drainage: The outer medullary networks typically extend into the medullary rays before joining interlobular veins, whereas the long vascular bundles of the inner medulla (vasa recta) converge abruptly and join the arcuate veins. (See previous section on medullary circulation.)
Determinants of Glomerular Ultrafiltration
Urine formation begins with filtration of a nearly protein-free fluid from the glomerular capillaries into Bowman’s space. The barrier to filtration includes the fenestrated endothelial surface of the glomerular capillaries; the three layers of the glomerular basement membrane; the filtration slits between adjacent pedicels or foot processes of the visceral epithelial cells (podocytes) that surround the capillaries; and the filtration slit diaphragm that extends along the filtration slits and connects adjacent foot processes to form the ultimate barrier to filtration (see Chapter 2 and Figure 3.13 ). Water, electrolytes, amino acids, glucose, and other endogenous or exogenous compounds with molecular radii smaller than 20 Å are freely filtered from the blood into Bowman’s space, whereas molecules larger than about 50 Å are virtually excluded from filtration. At any given point of a glomerular capillary wall, the process of ultrafiltration of fluid is governed by the net balance among the transcapillary hydraulic pressure gradient (ΔP), the transcapillary colloid osmotic pressure gradient (Δπ), and the hydraulic conductivity of the filtration barrier (k) on the basis of the Starling equation, as follows:
J v = k ( Δ P − Δ π ) = k [ ( P GC − P BS ) − ( π GC − π BS ) ]
SNGFR = kS ( Δ P ¯ − Δ π ¯ ) = K f P ¯ UF
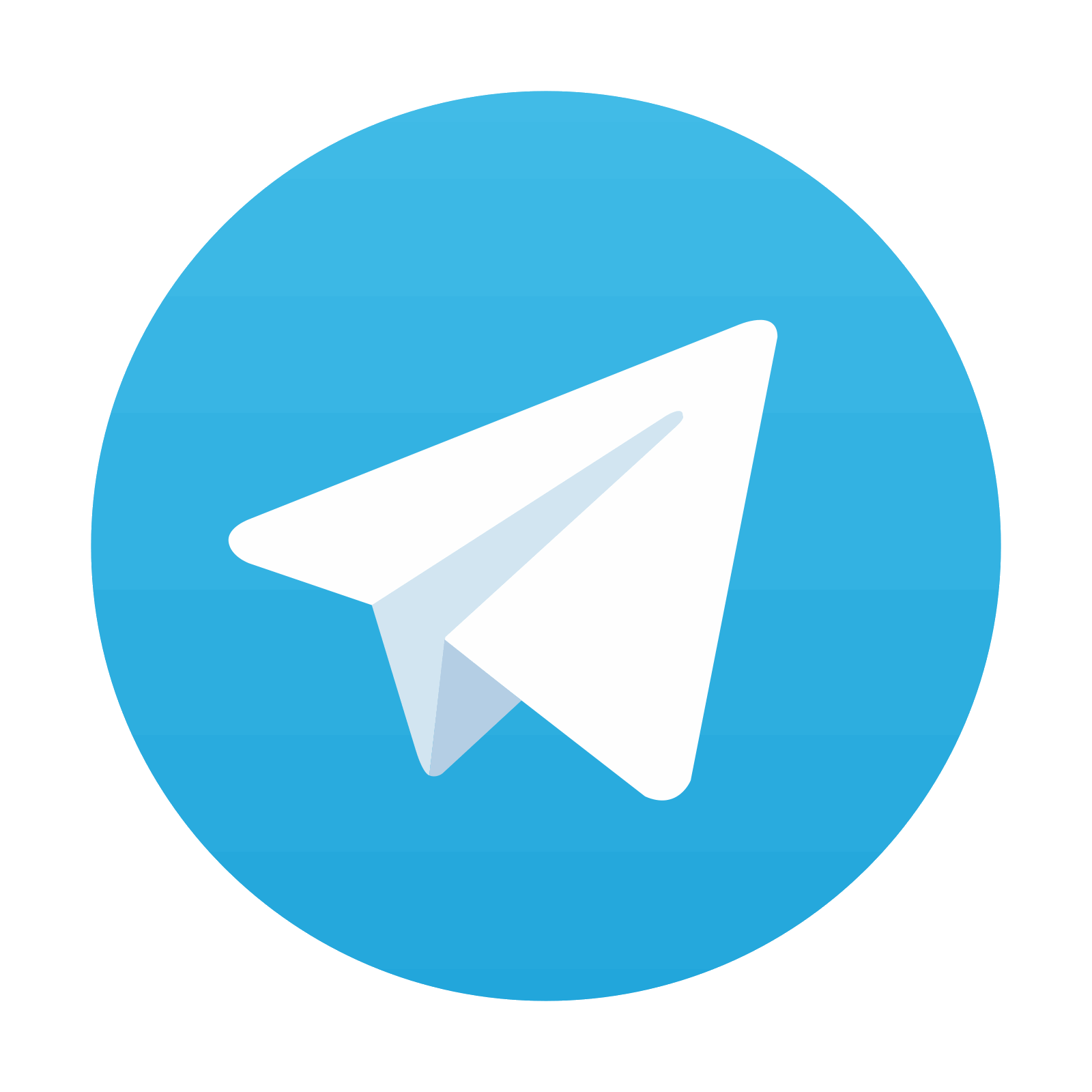
Stay updated, free articles. Join our Telegram channel
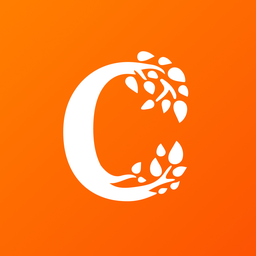
Full access? Get Clinical Tree
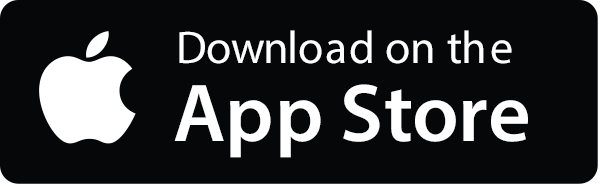
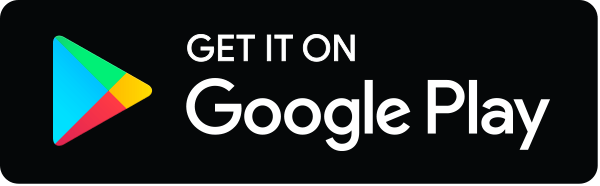