Chapter Outline
CALCIUM TRANSPORT IN THE KIDNEY, 185
Role of Calcium in Cellular Processes, 185
Calcium Present in Serum in Bound and Free Forms, 186
Regulation of Calcium Homeostasis by the Parathyroid Hormone–Vitamin D Endocrine System, 187
Reabsorption of Calcium along the Tubule, 187
Regulation of Ca 2+ Transport in the Kidney, 189
Regulation of Renal Calcium Transport by Novel Proteins, 191
Structures of Proteins Involved in the Transport of Calcium, 191
MAGNESIUM TRANSPORT IN THE KIDNEY, 193
Role of Magnesium in Cellular Processes, 193
Magnesium Present in Serum in Bound and Free Forms, 193
Regulation of Magnesium Homeostasis, 193
Reabsorption of Magnesium along the Tubule, 194
Regulation of Magnesium Transport in the Kidney, 195
Structures of Proteins Involved in the Transport of Magnesium, 195
PHOSPHORUS TRANSPORT IN THE KIDNEY, 196
Role of Phosphorus in Cellular Processes, 196
Phosphorus Present In Blood in Multiple Forms, 196
Regulation of Phosphate Homeostasis: An Integrated View, 197
Reabsorption of Phosphate along the Nephron, 198
Regulation of Phosphate Transport in the Kidney, 199
Structures of Proteins Involved in the Transport of Phosphorus, 202
In this chapter, we will discuss how the kidney regulates calcium, phosphorus, and magnesium balance and the manner in which various hormones and factors alter the efficiency with which these substances are reabsorbed by the kidney. The molecular processes responsible for the reabsorption of these substances by the kidney and the localization of the cognate molecular machinery along the nephron are unique for calcium, phosphorus, and magnesium. In the case of calcium and phosphorus, similar hormones regulate the efficiency of renal reabsorption, although specific factors for each substance also function to regulate reabsorption. With magnesium, the molecular mediators of reabsorption are poorly regulated, and the precise hormonal factors involved in the regulation of magnesium reabsorption by the nephron are less well defined.
Calcium Transport in the Kidney
Role of Calcium in Cellular Processes
Calcium is an abundant cation in the body ( Table 7.1 ). Several biochemical and physiologic processes, including nerve conduction and function, coagulation, enzyme activity, exocytosis, and bone mineralization, are critically dependent on normal calcium concentrations in extracellular fluid. Not unexpectedly, intricate mechanisms exist to maintain extracellular fluid calcium concentrations within a narrow range and to maintain calcium balance. Significant decreases in serum calcium concentrations are associated with Chvostek’s and Trousseau’s signs, tetany and, when profound, generalized seizures. A deficiency in calcium absorption in the intestine, such as occurs in vitamin D deficiency, is associated with secondary hyperparathyroidism, hypophosphatemia, and rickets or osteomalacia. Hypercalcemia, with attendant hypercalciuria, is associated with a reduced capacity to concentrate urine, volume depletion, and nephrocalcinosis and renal stones. As shown in Figure 7.1 , the intestine and kidney are important in the absorption and reabsorption and excretion of calcium. Following absorption in the intestine, calcium in the extracellular fluid space is deposited in bone (the major repository of calcium in the body) and is filtered in the kidney. The concentration of calcium in serum varies with age and gender, with higher values being present in children and adolescent subjects than in adults.
Body Weight (kg) | Water † (g) | Fat † (g) | Water (g) | N (g) | Na (mEq) | K (mEq) | Cl (mEq) | Mg (g) | Ca (g) | P (g) | Fe (mg) | Cu (mg) | Zn (mg) | B (mg) | Co (mg) |
---|---|---|---|---|---|---|---|---|---|---|---|---|---|---|---|
70 | 605 | 160 | 720 | 34 | 80 | 69 | 50 | 0.47 | 22.4 | 12.0 | 74 | 1.7 | 28 | 0.37 | 0.02 |
* As determined by chemical analysis (values per kilogram fat-free tissue, unless otherwise indicated).

Calcium Present in Serum in Bound and Free Forms
Calcium is present in plasma in filterable (60% of total calcium) and bound (40% of total calcium) forms. Filterable calcium is comprised of calcium complexed to anions such as citrate, sulfate, and phosphate (~10% of total calcium) and ionized calcium (~50% of total calcium; Figure 7.2 ). The percentage of calcium bound to proteins (predominantly albumin and, to a lesser extent, globulins), and, the amount of filterable calcium, is dependent on plasma pH. Alkalemia is associated with a reduction in free calcium, whereas acidemia is associated with an increase in free calcium. A 1-g/dL change in serum albumin is associated with a 0.8-mg/dL change in total serum calcium, and a 1-g/dL change in globulins is associated with a 0.16-mg/dL change in total serum calcium. An equation defining the amount of calcium (mmol/L) bound to albumin and globulins (g/L) as a function of pH is as follows :
[ CaProt ] = 0.019 [ Alb ] − [ ( 0.42 ) ( [ Alb ] / 47.3 ) ( 7.42 − pH ) ] + 0.004 [ Glob ] − [ ( 0.42 ) ( [ Glob ] / 25.0 ) ( 7.42 − pH ) ]

If one assumes that all calcium is bound to albumin, the following equation applies:
[ CaProt ] = 0.0211 [ Alb ] − [ ( 0.42 ) ( [ Alb ] / 47.3 ) ( 7.42 − pH ) ]
A nomogram describing this relationship is shown in Figure 7.3 .

Regulation of Calcium Homeostasis by the Parathyroid Hormone–Vitamin D Endocrine System
In states of neutral calcium balance, the amount of calcium absorbed by the intestine is equivalent to the amount excreted by the kidney. The central role of the parathyroid hormone–vitamin D endocrine system in the regulation of calcium homeostasis is well recognized and is summarized in Figure 7.4 . In response to reductions in calcium intake and subsequent decreases in the serum calcium level, parathyroid hormone release from the parathyroid glands is increased. The change in serum calcium concentration is detected by the parathyroid gland calcium-sensing receptor, a G protein–coupled receptor, which alters parathyroid hormone release from the parathyroid cell. Parathyroid hormone enhances the efficiency of calcium transport in the distal tubule of the kidney and increases the activity of the renal 25-hydroxyvitamin D 1α-hydroxylase, which enhances the formation of 1α,25-dihydroxyvitamin D (1α,25(OH) 2 D), the active metabolite of vitamin D. The reader is referred to reviews by Kumar and colleagues concerning details of the regulation of the synthesis of 1α,25(OH) 2 D. 1α,25(OH) 2 D increases the efficiency of calcium transport in the intestine and in the kidney. The effects of parathyroid hormone and 1α,25(OH) 2 D restore calcium balance by increasing the amount of calcium accreted. At the same time, parathyroid hormone and 1α,25(OH) 2 D increase bone calcium mobilization and help maintain serum calcium concentrations. The converse series of events occurs in hypercalcemic circumstances.

Reabsorption of Calcium Along the Tubule
The kidney reabsorbs filtered calcium in amounts that are subject to regulation by calciotropic hormones, parathyroid hormone (PTH), and 1α,25(OH) 2 D. The glomerulus filters 9000 to 10,000 mg of complexed and ionized calcium in a 24-hour period. The amount of calcium appearing in the urine is approximately 250 mg/day, and it is therefore evident that a large percentage of filtered calcium is reabsorbed. As a result of reabsorption processes that occur in both the proximal and distal tubules, only 1% to 2% of calcium filtered at the glomerulus appears in the urine. Figure 7.5 shows the percentages of calcium reabsorbed along different segments of the nephron.

Ca 2+ Reabsorption in the Proximal Tubule
This reabsorption is predominantly passive. As noted earlier, about 60% to 70% of total plasma calcium is free (not protein-bound) and is filtered at the glomerulus. A large percentage (~70%) of filtered calcium (Ca 2+ ) is reabsorbed in the proximal tubule (PT), mainly by paracellular processes that are linked with Na + reabsorption. In this nephron segment, the reabsorption of Na + and Ca 2+ is proportional under a variety of conditions and is not dissociated following the administration of several factors known to alter renal Ca 2+ reabsorption, such as PTH, cyclic adenosine monophosphate (cAMP), chlorothiazide, furosemide, acetazolamide, or changes in the hydrogen ion content. The precise cellular and molecular mechanisms responsible for the movement of Ca 2+ from the lumen of the proximal tubule into the interstitial space are not clearly defined. Most Ca 2+ is believed to move in between cells (paracellular movement), with a smaller, but significant, transcellular component ( Figure 7.6 ). The components of the paracellular pathway include claudin-2. Ca 2+ permeates through claudin-2 and simultaneously competitively inhibits Na + conductance. A transcellular component of Ca 2+ reabsorption may also be present in the proximal tubule. Undefined Ca 2+ channels and intracellular Ca 2+ binding proteins influence the movement of Ca 2+ into and across the cell. The Na + -K + -ATPase has been implicated in transcellular Ca 2+ transport in the proximal tubule, and both the Na + -Ca 2+ exchanger and isoforms 1 and 4 of the plasma membrane Ca 2+ pump are expressed in the proximal tubule and could be important in the movement of Ca 2+ out of the proximal tubule cell. Although the proximal tubule reabsorbs large amounts of Ca 2+ , primarily by paracellular processes, the rate of Ca 2+ reabsorption is not influenced by factors or hormones that regulate calcium balance. However, in conditions such as volume depletion, in which proximal tubule Na + reabsorption is increased, one also observes enhanced Ca 2+ reabsorption, which can contribute to the hypercalcemia that is sometimes seen in such situations. The salutary effects of isotonic saline administration in patients with hypercalcemia are attributable to a reduction in Ca 2+ reabsorption as a result of reduced Na + reabsorption.

Ca 2+ Reabsorption in the Loop of Henle
The thin descending and thin ascending limbs of the loop of Henle do not transport significant amounts of Ca 2+ . Of filtered Ca 2+ , 20% to 25% is reabsorbed in the thick ascending limb of Henle primarily by the paracellular route involving claudins 16 and 19. Thick ascending limb cells express the furosemide-sensitive Na + -K + -2Cl − cotransporter, NKCC2, which mediates the reabsorption of Na + and thereby contributes to the driving force for paracellular Ca 2+ transport. A lumen-positive transepithelial potential is generated in the thick ascending limb of the loop of Henle through the activity of the NKCC2 (Na + -K + -2Cl − cotransporter) by two mechanisms, secondary apical recycling of K + via the renal outer medullary potassium (ROMK) channel and a NaCl diffusion potential generated by reabsorbed NaCl establishing a concentration gradient across the Na-selective paracellular pathway. This transepithelial voltage provides the driving force for passive Ca 2+ reabsorption through the paracellular pathway.
The specific role played by claudins in the tight junction of the thick ascending limb of Henle in Ca 2+ reabsorption (and Mg 2+ reabsorption, as discussed in the next section) is controversial. Together with claudin-19, claudin-16 forms a paracellular pore and a heteromeric claudin-16, and claudin-19 interaction is required to assemble and traffic to the tight junction and to generate cation-selective paracellular channels. It has been postulated that these channels are themselves responsible for permeating divalent cations, Ca 2+ and Mg 2+ , via the paracellular route. An alternative prevailing hypothesis is that claudins 16 and 19 form Na + channels and act primarily to establish the transepithelial NaCl diffusion potential, thus contributing to the driving force for divalent cation reabsorption. Regardless of the mechanism, loss of function mutations in the genes encoding claudin-16 and claudin-19 result in familial hypomagnesemia with hypercalciuria and nephrocalcinosis, which is characterized by renal Ca 2+ and Mg 2+ wasting because of defective thick ascending limb divalent cation reabsorption. Similarly, mutations of NKCC2 are associated with the common form of Bartter’s syndrome, which, like the other Bartter’s forms, can be associated with hypercalciuria.
There is considerable species heterogeneity with respect to responses to calcium-regulating hormones by the thick ascending limb; in the mouse, PTH and calcitonin (CT) stimulate Ca 2+ transport in the cortical thick ascending limb, whereas in the rabbit CT stimulates calcium reabsorption in the medullary thick ascending limb but not in the cortical thick ascending limb. Extracellular fluid calcium also regulates calcium reabsorption in this segment through the Ca 2+ -sensing receptor (see below).
Ca 2+ Reabsorption in the Distal Tubule
This reabsorption is hormonally regulated, transcellular, and active and is mediated by specific channels, proteins, and pumps. In the distal convoluted tubule (primarily DCT2) and connecting tubule (together abbreviated as DT), 5% to 10% of filtered Ca 2+ is reabsorbed by active transport processes against electrical and concentration gradients. Ca 2+ reabsorption in this segment of the nephron is regulated by PTH, calcitonin, and 1α,25(OH) 2 D 3 , hormones that increase the efficiency of Ca 2+ reabsorption in this nephron segment. Ca 2+ reabsorption in the DT occurs via a transcellular pathway. Mediators of Ca 2+ transport in the renal DT include apically situated, transient receptor potential cation channels, subfamily V, types 5 and 6 channels (TRPV5, TRPV6), which mediate the increase in Ca 2+ uptake from the lumen into the cell ; micropuncture studies in knockout mice have indicated that TRPV5 is the gatekeeper of Ca 2+ reabsorption in the accessible DT in mice ( Figure 7.7 A ). Intracellular Ca 2+ binding proteins such as calbindin D9K and D28K facilitate the movement of Ca 2+ across the cell, and the basolateral plasma membrane calcium (PMCA) pump, Na + -Ca 2+ exchanger (NaCX), and Na + -Ca 2+ -K + exchanger (NaCKX) increase the rate of extrusion of Ca 2+ across the basolateral membrane (see Figure 7.7 B and C ). The Na + gradient for the activity of the NaCX and the NaCKX is provided by the Na + -K + -ATPase situated at the basolateral cell membrane (not shown).

Regulation of Ca 2+ Transport in the Kidney
Calcium-Regulating Hormones
Calcium-regulating hormones alter the expression of calcium channels, calcium-binding proteins, calcium pumps, and exchangers in the kidney by varied mechanisms. PTH increases the activity of TRPV5 channels in the kidney by activating cAMP-PKA (protein kinase A) signaling and phosphorylating a threonine residue within the channel, resulting in an increase in the open probability of the channel. PTH also activates the PKC pathway and increases the numbers of TRPV5 channels on the surface of tubular cells by inhibiting endocytosis of the caveolae in which the channels are located. 1α,25(OH) 2 D 3 enhances the expression of TRPV5 and TRPV6 channels present in the distal and connecting tubules and cortical collecting duct by increasing respective messenger RNA (mRNA) concentrations through increased binding of the vitamin D receptor to response elements in the gene promoters. 1α,25(OH) 2 D 3 increases the expression of calbindin D9K and D28K and the PMCA pump in the kidney and cultured renal cells. The effect of PTH and 1α,25(OH) 2 D 3 is to increase the expression of Ca 2+ channels, binding proteins, pumps, and exchangers, thereby increasing the retention of calcium by the kidney.
Extracellular Calcium
The level of extracellular calcium regulates renal Ca 2+ reabsorption by signaling through the Ca-sensing receptor (CaSR). In the kidney, the CaSR is primarily expressed on the basolateral membrane of the thick ascending limb of the loop of Henle (TALH). Activation of the CaSR reduces renal tubular Ca 2+ reabsorption and induces calciuresis in response to a Ca load. One mechanism is by inhibition of NKCC2 expression or activity. More recently, it has been suggested that CaSR acts primarily by regulating paracellular permeability. Loupy and colleagues have shown that a CaSR antagonist increases Ca 2+ permeability in isolated perfused TALH, with no change in transepithelial voltage or Na flux. This appears to be mediated by regulation of the expression of claudin-14. Activation of the CaSR causes robust upregulation of claudin-14, which, through physical interaction, inhibits paracellular cation channels formed by claudin-16 and claudin-19. The signaling mechanism seems to involve CaSR inhibiting calcineurin, a phosphatase that normally activates the nuclear factor of activated T cells (NFAT) to increase transcription of two micro-RNAs, miR-9 and miR-374, thereby downregulating claudin-14 expression. The central role of claudin-14 is further supported by the finding that claudin-14 knockout mice are unable to increase their fractional excretion of calcium in response to a high-Ca 2+ diet and exhibit complete loss of regulation of urinary Ca 2+ excretion in response to a CaSR agonist or antagonist.
Diuretics
Loop diuretics such as furosemide increase urinary calcium losses. The mechanism whereby furosemide causes hypercalciuria is linked to its ability to bind to and inhibit the furosemide-sensitive Na + -K + -2Cl − cotransporter type 2, NKCC2, present in the TALH. NaCl absorption is diminished, as is potassium recycling, resulting in a reduction in lumen positivity that drives Ca 2+ reabsorption. Subjects with the common form of Bartter’s syndrome have inactivating mutations of the NKCC2, which are associated with calciuria. Compensatory increases occur in the expression of distal tubule transport channels and proteins, such as the TRPV5 and TRPV6 channels and calbindin D28K following the administration of furosemide, but fail to compensate for the increase in excretion that occurs in the TALH.
Thiazide diuretics, on the other hand, cause hypocalciuria, and the effect appears to be independent of PTH in humans and rodents. Thiazides bind to and inhibit the Na-Cl cotransporter in the distal tubule. Chronic thiazide use is associated with a reduction in extracellular fluid volume, which secondarily enhances Na + and Ca 2+ reabsorption in the proximal tubule of the kidney. Distal tubule Ca 2+ transport is clearly unaffected by chronic thiazide use, in contrast to older reports that thiazide acutely increases Ca 2+ reabsorption in an isolated perfused DCT. The development of hypocalciuria parallels a compensatory increase in Na + reabsorption secondary to an initial natriuresis following thiazide administration. These observations are supported by the upregulation of the Na + -H + exchanger, responsible for most of the Na + and associated Ca 2+ reabsorption in the proximal tubule, whereas the expression of proteins involved in active Ca 2+ transport in the distal tubule was unaltered. Indeed, thiazide administration was associated with hypocalciuria in Trpv5 knockout mice. Humans with Gitelman’s syndrome and inactivating mutations of the thiazide-sensitive Na-Cl transporter have hypocalciuria, hypomagnesemia, and volume depletion, findings that are recapitulated in Na-Cl cotransporter knockout mice.
Estrogens
Estrogens influence calcium transport in the kidney because postmenopausal women have higher urinary Ca 2+ excretion than premenopausal women. In the early postmenopausal period, the administration of estrogen is associated with a decrease in urine Ca 2+ excretion and an increase in serum PTH and 1α,25(OH) 2 D levels. Estradiol increases the expression of the TRPV5 channel in the kidney in a manner independent of 1α,25(OH) 2 D 3 . These observations are supported by reduced duodenal TRPV5 channel expression in mice lacking the estrogen receptor alpha.
Metabolic Acidosis and Alkalosis
Metabolic acidosis is associated with hypercalciuria and, when prolonged, often results in bone loss and osteoporosis. Metabolic acidosis and metabolic alkalosis decrease or increase the reabsorption of Ca 2+ in the distal tubule, expression of TRPV5 in the distal tubule, and activity of TRPV5 channels.
Regulation of Renal Calcium Transport by Novel Proteins
Klotho
Klotho is a co-receptor for the phosphaturic peptide, fibroblast growth factor 23 (FGF-23), with β-glucuronidase activity. It is a kidney- and parathyroid gland–specific protein, which influences epithelial Ca 2+ transport by deglycosylating TRPV5, thereby trapping the channel in the plasma membrane and sustaining the activity of the channel. Further evaluation of serum Klotho concentrations and their association with changes in renal calcium excretion are required to establish a role for this factor in the regulation of renal calcium transport.
Sclerostin
Sclerostin is an osteocyte-derived glycoprotein that influences bone mass. Patients with sclerosteosis and its milder variant, van Buchem’s disease, have exceptionally dense bones and skeletal overgrowth that often constricts cranial nerve foramina and the foramen magnum, resulting in premature death. Sclerosteosis is caused by inactivating mutations of the sclerostin ( SOST ) gene, and the milder van Buchem’s disease is caused by a 52-kb deletion of a downstream enhancer element of the sclerostin gene. Mouse models of sclerosteosis have increases in skeletal mass similar to those found in patients with the disease and, by using a Sost gene knockout model generated in our laboratory, we have demonstrated that sclerostin, directly or indirectly, through an alteration in the synthesis of 1α,25(OH) 2 D, influences renal calcium reabsorption in the kidney. Urinary calcium excretion and renal fractional excretion of calcium are decreased in Sost −/− mice. Serum 1α,25(OH) 2 D concentrations are increased without attendant hypercalcemia; renal 25(OH)D-1α hydroxylase ( Cyp27b1 ) mRNA and protein expression are also increased in Sost −/− mice, strongly suggesting that the increase in serum 1α,25(OH) 2 D concentrations was the result of increased 1α,25(OH) 2 D synthesis. When recombinant sclerostin is added to cultures of proximal tubular cells, the expression of the messenger RNA for Cyp27b1, the 1α-hydroxylase cytochrome P450, is diminished. Serum 24,25(OH) 2 D concentrations were diminished in Sost −/− mice, and PTH concentrations were similar in knockout and wild-type mice. The lack of change in PTH is consistent with previous studies in humans. The data suggest that in addition to the hormones traditionally thought to alter calcium reabsorption in the kidney (PTH and 1α,25(OH) 2 D), sclerostin plays a significant role in altering renal calcium excretion. Although PTH and 1α,25(OH) 2 D decrease fractional excretion of calcium by increasing the efficiency of calcium reabsorption in the DT, sclerostin increases fractional excretion of calcium (the absence of sclerostin expression is associated with a reduced fractional excretion of calcium). Thus, the adaptation to a reduction in calcium intake and resultant downstream alterations in hormones (see Figure 7.2 for current understanding) may need to be amended to include changes in sclerostin expression (see Figures 7.4 and 7.7 C ). In the modified scheme, reduced sclerostin expression, which can occur as a result of increases in PTH, would enhance renal Ca 2+ reabsorption directly or through changes in 1α,25(OH) 2 D synthesis (see Figure 7.4 ). The change in 1α,25(OH) 2 D synthesis might be direct or mediated through changes in FGF-23 concentrations. Clearly, further work needs to be performed to dissect the proximate drivers of increased sclerostin-mediated renal Ca + reabsorption.
Structures of Proteins Involved in the Transport of Calcium
The structural analysis and molecular modeling of Ca 2+ transporters and Ca 2+ -binding proteins have revealed surprisingly diverse mechanisms whereby these proteins bind the metal ligand. Such information is important because it suggests how drugs might be designed to inhibit or enhance the activity of these Ca 2+ transporters. As noted earlier, the TRPV5 channel, calbindin D28K, and plasma membrane calcium pump are important in the transport of Ca 2+ across the cell. Figure 7.8 A shows a homology model for the human TRPV5. The TRPV5 channel forms a tetramer. Each TRPV5 protomer, shown by a blue, green, red, and yellow trace of α carbon backbone atom positions, putatively contains six transmembrane helices that may traverse the phospholipid bilayer as drawn. Most of the tetramer, including the N- and C-termini, is intracellular. Residues of transmembrane helices 5 and 6 form a central pore (black), where regulated calcium influx occurs. Residue D542 was reported as essential for Ca 2+ selectivity and likely creates the first of two calcium binding sites (red spheres) inside a funnel-like cavity within the pore’s extracellular entry. Residue T539 may form another calcium binding site closer to the pore. N572 and I575 seemingly perform gatelike functions because the pore’s diameter appears restricted by these residues. Ca 2+ influx through TRPV5 is inhibited by a feedback mechanism when Ca 2+ -bound calmodulin binds to amino acids 691 to 711 ( arrow ) between two TRPV5 protomers; the key contacts are the conserved R699, W701, L704, R705, and L709. PTH-mediated PKA kinase activation leads to phosphorylation of T708 at this site, which stimulates TRPV5 by inhibiting calmodulin binding.

The structure of Ca-loaded calbindin D28K bound to Ca 2+ has been solved (see Figure 7.8 B ). Calbindin D28K contains six EF-hand motifs, which are canonical helix-loop-helix structures that coordinate Ca 2+ . As shown, only four of the six EF hands in calbindin D28K bind Ca 2+ ions with significant binding affinity—EF hand 1 (red Ca 2+ ), EF hand 3 (blue Ca 2+ ), EF hand 4 (purple Ca 2+ ), and EF hand 5 (cyan Ca 2+ ). The yellow surface patches indicate residues that display substantial chemical shifts when calbindin D28K is titrated with three peptides derived from other proteins known to interact. Unlike the conformation of many EF-hand proteins, such as calmodulin, Ca 2+ -bound calbindin D28K forms one ordered domain. The apo calbindin D28K structure is also mostly ordered. However, EF hands 1 and 4, a segment prior to EF hand 1 and polypeptide between EF hands 4 and 5 and 5 and 6, are altered in conformation from the fully Ca 2+ -bound form. When only one, two, or three Ca 2+ are bound, calbindin D28K is considerably more disordered.
A model of the human plasma membrane calcium pump A1 (PMCA1) is shown in Figure 7.8 C . The protein acts as a high-affinity, Ca 2+ -H + P-type ATPase (cotransporter) that removes Ca 2+ from cells at a slow rate against their electrochemical gradient. The structural features of the PMCA1 are 10 transmembrane helices, with most of the polypeptide inside the cytoplasm (bottommost), organized into three domains, termed P, N, and A. The P, or phosphorylation domain, has the highly conserved catalytic core, with a canonical Rossmann fold common among ATPases. The N, or nucleotide-binding domain, is a region of β-sheet that oscillates among conformations to deliver bound ATP to the P domain’s phosphorylation site. The A, or actuator domain, is also very mobile and actually consists of two subdomains, which may exist to protect the phosphoryl group from hydrolysis and sometimes block ion access or egress. The mechanism whereby the PMCA1 transports Ca 2+ begins with Ca 2+ binding to a site(s) in the transmembrane domain (key residues are N859, N891, and D895) made cytoplasmically accessible because helices 4 and 6 are structurally altered when the P domain is unphosphorylated. Two calciums, shown as red spheres, are modeled here based on a crystal structure of the homologous sarcoplasmic reticulum Ca 2+ -ATPase. ATP-Mg 2+ binding to a site ( arrow ) that perturbs an important salt bridge between R646 and D248 brings the N domain into closer proximity with the P domain’s residue D475. ATP hydrolysis leads to phosphorylation of D475 and large-scale conformational changes result, such as a 90-degree rotation of the A domain and rearrangement of transmembrane helices 4 and 6 to cause extracellular release of Ca 2+ . There remains some debate about whether the ratio of calcium flux is one calcium per hydrolyzed ATP molecule. PMCA1 is autoinhibited by its C terminus; this regulation is relieved when calmodulin binds to a calmodulin-binding domain, also within the C terminus. PMCA1 is also activated by acidic phospholipids, although a full understanding is lacking.
Magnesium Transport in the Kidney
Role of Magnesium in Cellular Processes
Magnesium is an abundant cation in the human body ( Table 7.1 ). It is required for a variety of biochemical functions. The activities of magnesium-dependent enzymes are modulated by the metal as a result of binding to the substrate or of direct binding to the enzyme. Enzymes of the glycolytic and citric acid pathways, exonuclease, topoisomerase, RNA and DNA polymerases, and adenylate cyclase are among the many enzymes regulated by magnesium. Also, magnesium regulates channel activity.
Given its role in such diverse biologic processes, it is not surprising that a deficiency or increase in serum magnesium concentration is associated with important clinical symptoms. For example, a low serum magnesium concentration is associated with muscular weakness, fasciculations, Chvostek’s and Trousseau’s signs, and sometimes frank tetany. The tetany of hypomagnesemia is independent of changes in the serum calcium level. On occasion, personality changes, anxiety, delirium, and psychoses may manifest. Hypocalcemia, reduced PTH secretion, and hypokalemia are sometimes present in patients with hypomagnesemia. Cardiac arrhythmias and prolongation of the corrected QT interval are sometimes observed. Conversely, hypermagnesemia seen in association with the administration of excessive amounts of magnesium in diseases such as eclampsia and in patients with renal failure is manifest as weakness of the voluntary muscles.
Magnesium Present in Serum in Bound and Free Forms
Most magnesium within the body is present in bone or within the cells ( Table 7.2 ). Approximately 60% of magnesium is stored in bone. The serum magnesium concentration varies slightly with age; in adults, it is 1.6-2.3 mg/dL (0.66 to 0.94 mmol/L). In plasma, about 70% of Mg is ultrafiltrable, 55% is free, and 14% of Mg is in the form of soluble complexes with citrate and phosphate. Because Mg is present largely within cells and bone, there is some interest as to whether serum Mg concentration reflects tissue stores, especially when Mg is depleted or deficient. When rats and humans are placed on Mg-deficient diets, the serum Mg level decreases within 1 day in rats and in 5 to 6 days in humans. Bone Mg and blood mononuclear cell Mg concentrations correlate well with total body Mg and serum Mg levels. The correlations between total body Mg stores and muscle or cardiac Mg, however, are not precise.
Site | Whole-Body Mg (%) | Concentration and Content |
---|---|---|
Bone | 53 | 0.5% of bone ash |
Muscle | 27 | 9 mmol/kg wet weight |
Soft tissue | 19 | 9 mmol/kg wet weight |
Adipose tissue | 0.012 | 0.8 mmol/kg wet weight |
Erythrocytes | 0.5 | 1.65-2.73 mmol/L |
Serum | 0.3 | 0.69-0.94 mmol/L |
Regulation of Magnesium Homeostasis
The intestine and the kidney regulate magnesium balance ( Figure 7.9 ). A normal diet adequate in magnesium normally contains 200 to 300 mg of magnesium. Of ingested dietary magnesium, 75 to 150 mg is absorbed in the jejunum and ileum, primarily by paracellular passive processes. The Trpm6 protein (a mutant form of this protein is present in patients with familial hypomagnesemia) is localized to the apical membrane of intestinal epithelial cells and mediates transcellular magnesium absorption. About 30 mg of magnesium is secreted into the intestine via pancreatic and intestinal secretions, giving a net magnesium absorption of approximately 130 mg/24 hr. Magnesium that is not absorbed in the intestine and is secreted into the intestinal lumen eventually appears in the feces (125 to 150 mg). Absorbed magnesium enters the extracellular fluid pool and moves in and out of bone and soft tissues. Approximately 130 mg of magnesium (equivalent to the net amount absorbed in the intestine) is excreted in the urine.

In experimental animals and humans, feeding a diet low in magnesium results in a rapid decrease in urinary and fecal magnesium and the development of a negative magnesium balance. Conversely, the administration of magnesium is associated with an increase in the renal excretion of magnesium. Unlike calcium and phosphorus, however, no hormones or molecules have been identified that alter magnesium transport in the intestine or alter the renal excretion of magnesium in response to changes in magnesium balance.
Reabsorption of Magnesium Along the Tubule
Approximately 10% of total body magnesium is filtered in glomeruli (≈3000 mg/24 hr). About 75% of total plasma magnesium is filterable. Because urinary magnesium excretion is about 150 mg/24 hr, a substantial fraction of filtered magnesium is reabsorbed along the tubule (≈95%). Fifteen percent to 20% of filtered magnesium is reabsorbed in the proximal tubule ( Figure 7.10 ). The cellular and molecular mechanisms whereby magnesium is reabsorbed in the proximal nephron are unknown. However, it is speculated that reabsorption of magnesium in the proximal nephron occurs by paracellular mechanisms. The bulk of the filtered magnesium is reabsorbed in the TALH, again by a paracellular mechanism of which paracellin-1 or claudin-16 is a critical component. As discussed earlier for Ca 2+ transport, claudin-16 and claudin-19 form cation-selective paracellular channels that directly mediate paracellular Mg 2+ reabsorption or facilitate the generation of a NaCl diffusion potential that provides the driving force for paracellular Mg 2+ reabsorption. Mutations of the CLDN16 and CLDN19 genes and the SLC12A1, KCNJ1 and CLCNKB genes, which encode proteins required for normal thick ascending limb function, result in excessive magnesium losses in the urine and hypomagnesemia. Figure 7.11 shows the mechanism whereby magnesium is transported in the TALH.
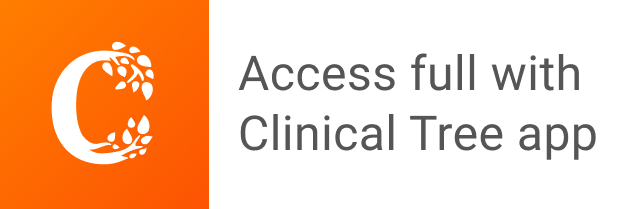