Structural-Functional Relationships in the Kidney
Wilhelm Kriz
Astrid Weins
Ruth Ellen Bulger
STRUCTURE-FUNCTION CORRELATIONS ALONG THE RENAL STRUCTURE
The kidney functions as it does, in large part, because of its architecture. In no instance is this more evident than in the urinary concentrating mechanism, where the complex nephron and vascular interrelationships permit the coordinated function of different nephron and vascular elements into countercurrent multiplication and exchange processes. A recent proliferation of detailed structural, biochemical, and functional information has led to an appreciation of other structural-functional relationships that are relevant to solute and water handling by the kidney. Moreover, it has become evident that an understanding of the pathologic developments in kidney diseases is only possible on the basis of a thorough knowledge of kidney structure.
The purpose of this chapter is to review some of the recent findings, with special emphasis on structural-functional relationships, to enhance our understanding of overall renal function; therefore, this chapter is divided into two parts. The first part considers the structural and functional interrelationships of each morphologic segment of the urinary tubule, stressing the unique characteristics of each segment. The second part discusses structure and function in terms of more general mechanisms used by several segments of the renal tubule to accomplish specific functions, such as ion or water transport.
FORM OF THE HUMAN KIDNEY
Human kidneys are paired, bean-shaped organs situated in a retroperitoneal position on the posterior aspect of the abdominal cavity, on either side of the vertebral column against the psoas major muscle. A fibrous capsule located within the perirenal adipose tissue and surrounded by perirenal fascia surrounds each kidney. The lateral border of each kidney is convex. The kidneys of an adult man weigh approximately 120 to 170 g each and measure roughly 11 × 6 × 2.5 cm; those of an adult woman weigh slightly less and are somewhat smaller. In both men and women, total kidney mass best correlates with body surface area.
The concave medial margin has a slitlike aperture, called the renal hilum. Branches of the renal artery, vein, nerves, lymphatics, and the expanded pelvis of the ureter pass through the hilum. The hilum communicates with a flattened space within the kidney called the renal sinus. Within this space, the renal pelvis branches into major and minor calyces.
Sections through the kidney reveal the cortex and medulla (Fig. 1.1). The human kidney is a multilobar organ containing 4 to 18 (average, 8) pyramids of medullary substance1 and is situated so that their bases are adjacent to the cortex. The darker red cortical substance covers the base of each medullary pyramid like the cap of an acorn. During fetal life, the kidney surface is demarcated by clefts that gradually disappear in the normal adult kidney. The apex of each medullary pyramid (called the papilla) extends into the renal sinus and is capped by a funnel-shaped, minor calyx. The minor calyces receive the urine that is released from the kidney into the extrarenal collecting system. A lobe of the kidney is composed of the conical medullary pyramid and the surrounding cortical substance. During fetal development, some lobes may fuse and calyces are remodeled so that the mature kidney has fewer calyces and papillae than the original number of papilla anlagen1; one calyx may drain a fused papilla developed from up to four anlagen, predominantly at the kidney poles. Striated elements called medullary rays extend peripherally at intervals from the bases of the medullary pyramids and penetrate into the cortex. These rays resemble the medulla in structure and although they extend deeply into the cortex, they are part of the cortex. The rest of the cortex is called the cortical labyrinth.
The medulla can be subdivided further either grossly or microscopically (Fig. 1.2). The medulla has an outer zone that is adjacent to the cortex and an inner zone that includes the papilla. The outer zone is subdivided into an inner and outer stripe. This zonation is important because it represents the location and orientation of the various segments of the renal tubules within the kidney.
The relative volumes occupied by the cortex, outer medulla, and inner medulla are 70%, 27%, and 3%, respectively,2 in humans. The relative thicknesses vary considerably among mammalian species.
RENAL (URINIFEROUS) TUBULES
Human renal morphology resulted from a long evolutionary process in which animals adapted to many changing environmental conditions. The three sequential types of kidneys that evolved were the pronephros, mesonephros, and metanephros. The urogenital system of each human embryo repeats this evolutionary process. The pronephros develops first, but degenerates before attaining any functional capacity.
The mesonephric kidney functions for a short period in utero, but it also degenerates, with the notable exception of the part of the mesonephric tubules that form a portion of the excurrent duct system of the male reproductive tract. The metanephric kidney forms last and eventually becomes the functional kidney of the human. The metanephric kidney is well suited to the human condition because of its efficient filtering device and its complex tubule, which allows for the production of not only dilute urine but also concentrated urine. This process occurs only in mammals and birds. Although it is well suited for maintaining homeostasis, the mammalian kidney is an inefficient organ for the elimination of salt and water. In humans, 180 L of fluid are filtered into the tubular lumen every 24 hours, of which approximately 178 L must be returned to the blood.
Each human kidney contains approximately 1 million functional units, called nephrons (with considerable interindividual variation).3 Each nephron is made up of a renal corpuscle (glomerulus) and a complex tubular portion, which drain into a unifying tubular system called the collecting duct system. Both kinds of tubules represent the renal (or uriniferous) tubules.
The nephrons are derived from the metanephric blastema, the collecting ducts from the urethral bud. A connecting tubule lies between the nephron and collecting ducts. At present, there is controversy as to whether the connecting tubule is derived from the metanephric blastema4,5,6 or the ureteric bud.7 As is discussed in the next section, the connecting tubule has marked morphologic similarities to the cortical collecting duct (CCD).
The segmentation of the renal tubule then includes the following regions.8
The Nephron
I. Renal corpuscle (most of which is called glomerulus)
Bowman’s capsule
Glomerular tuft
II. Proximal tubule
Convoluted part (pars convoluta) consists of P1 and the first part of P2 (PCT)
Straight part (pars recta) consists of the last part of P2 and all of P3 (PST)
III. Thin limbs of the loop of Henle (intermediate tubule)
Thin descending part of short-looped nephrons (SDTL)
Upper thin descending part of long-looped nephrons (LDTL up)
Lower thin descending part of long-looped nephrons (LDTL lp)
Ascending thin part of long-looped nephrons (ATL)
IV. Distal tubule
Straight part (pars recta)
Medullary thick ascending limb (MTAL), which includes regions located within the inner stripe and outer stripe of the medulla
Cortical thick ascending limb (CTAL), which includes the part ascending through the cortex, the macula densa (MD), and the post macula densa segment
Convoluted part (pars convoluta) (DCT)
The Collecting Duct System
I. Connecting tubule (CNT)
II. Cortical collecting duct (or tubule) (CCD)
III. Outer medullary-collecting duct (or tubule) (OMCD)
IV. Inner medullary-collecting duct (or tubule) including the papillary collecting ducts (also called the ducts of Bellini; IMCD)
Nephrons lie in characteristic positions (Fig. 1.2), with the renal corpuscles and proximal convoluted segments in the cortex (Figs. 1.3 and 1.4). The straight part of the proximal tubule, the thin limb segments, and the straight part of the distal tubule form the loop of Henle, which enters a medullary ray of the cortex and extends into the medulla, where it bends, returning to the cortex by means of the same medullary ray. The loops of juxtamedullary nephrons directly connect the outer stripe of the medulla without ever being contained in a medullary ray. As the straight part of the distal tubule returns to the cortex, it passes by the renal corpuscle from which the nephron originated, forming the macula densa; then, after a short postmacula densa segment, it continues as the distal convoluted tubule within the cortex.
The morphology of the nephron varies with the position of the renal corpuscle in the cortex. Each nephron is classified as superficial, midcortical, or juxtamedullary, according to the position of its renal corpuscle within the respective regions of the cortex (Fig. 1.2) and the pattern of efferent vessel formation.9,10,11 A given segment tends to occupy a specific region of the kidney, which gives rise to the gross zonation referred to in the preceding text. In the human kidney, superficial nephrons empty singly into a terminal collecting duct, whereas several juxtamedullary nephrons empty into an arched tubular portion (arcade) that courses peripherally in the cortex before it turns to enter a medullary ray. Most midcortical nephrons from humans empty individually as well.5,12 As known from the study of several species (rats and rabbits), an arcade is established by the connecting tubule epithelium (data from studies in humans are not available).
Nephrons also are classified as short or long looped according to the location of the position where their loops of Henle turn within the kidney. Short-looped nephrons arise from renal corpuscles located in superficial and midcortical regions and have loops of Henle that turn within the outer medulla. In humans, some superficial nephrons may have loops within the cortex itself. Short-looped nephrons have
short, thin limb segments that occur only along the descending limb. Long-looped nephrons have loops of Henle that turn within the inner medulla and have thin limb segments in both descending and ascending limbs. Although most species have both long- and short-looped nephrons, some species, such as dogs and cats, have only long ones,13 whereas other species, such as beavers, have only short ones.14,15 In human kidneys, the ratio of short- to long-looped nephrons is 6:1 to 7:1.
short, thin limb segments that occur only along the descending limb. Long-looped nephrons have loops of Henle that turn within the inner medulla and have thin limb segments in both descending and ascending limbs. Although most species have both long- and short-looped nephrons, some species, such as dogs and cats, have only long ones,13 whereas other species, such as beavers, have only short ones.14,15 In human kidneys, the ratio of short- to long-looped nephrons is 6:1 to 7:1.
THE RENAL CORPUSCLE
The renal corpuscle (first segment of the nephron) is the site at which an ultrafiltrate of the blood is produced (Fig. 1.5). The filtrate moves from the capillary lumen into Bowman’s space. This flow is influenced by the following factors: renal blood flow; the oncotic and hydrostatic pressures in the capillaries and in Bowman’s space; the size, shape, and charge of plasma molecules; and the various morphologic components of the wall separating the capillary lumen from Bowman’s space. The filtrate contains only barely detectable quantities of plasma proteins.16 The filtration barrier increasingly restricts the passage of larger molecules, with very little filtration of molecules larger than albumin (70 kDa).17
The renal corpuscle consists of Bowman’s capsule and the glomerular tuft. The latter is made up of capillaries, derived from the afferent arteriole, their supporting cells, and an envelope consisting of the glomerular basement membrane (GBM) and the visceral (podocyte) layer of Bowman’s capsule (Fig. 1.5). At the vascular pole, the visceral epithelium becomes the parietal epithelium, which then transforms into the proximal tubule epithelium at the urinary pole (Fig. 1.5). The space between both layers is the urinary space (Bowman’s space).
The human renal corpuscle is roughly ovoid and approximately 150 to 240 µm in diameter. The term glomerulus is widely used to refer to the entire renal corpuscle. The renal corpuscle without the parietal epithelial cells is referred to as the glomerular tuft. The afferent arteriole enters the renal corpuscle at the vascular pole, where it divides into several primary branches that each ramify to form a network of anastomosing capillaries, called a lobule. The lobule has a supporting region called the mesangium (Figs. 1.6 and 1.7). All lobules together establish the tuft; the converging mesangial regions are called the glomerular stalk, by which the tuft is connected to the extraglomerular mesangium (see Juxtaglomerular Apparatus). The capillaries coalesce toward the center of the capillary tuft to form
the efferent arteriole, which runs through the stalk and exits from the vascular pole. The efferent arteriole again breaks up to form a second capillary network, which surrounds the tubules and is called the peritubular capillary network.
the efferent arteriole, which runs through the stalk and exits from the vascular pole. The efferent arteriole again breaks up to form a second capillary network, which surrounds the tubules and is called the peritubular capillary network.
The renal corpuscle, therefore, consists of the following parts: (a) the parietal epithelium, (b) the visceral epithelium (podocytes), (c) the endothelial cells lining the capillaries, (d) the glomerular basement membrane (GBM), and (e) the intraglomerular mesangial cells and matrix. In the rat, the ratio of the number of endothelial cells to mesangial cells to visceral epithelial cells is 3:2:1.18
The Visceral Epithelium of Bowman’s Capsule
The visceral epithelial cells (nowadays generally called podocytes) are octopus-shaped cells that reside in Bowman’s space and attach to the GBM only by way of their processes (see below). This shape was well described by Zimmermann19 and is seen to advantage in scanning electron micrographs (SEM) (see later, Fig. 1.10). The exact details of cell shape differ depending
on the species being studied.20,21 As a consequence of the high degree of differentiation, podocytes, like neurons, are incapable of regenerative cell replication in the adult. Thus, podocytes that were lost, for any reason, cannot be replaced by new ones.22,23,24 On the other hand, in glomerular diseases based on dedifferentiation of podocytes (collapsing glomerulopathy), a vivid proliferation of the dedifferentiated cells accompanies the disease.25
on the species being studied.20,21 As a consequence of the high degree of differentiation, podocytes, like neurons, are incapable of regenerative cell replication in the adult. Thus, podocytes that were lost, for any reason, cannot be replaced by new ones.22,23,24 On the other hand, in glomerular diseases based on dedifferentiation of podocytes (collapsing glomerulopathy), a vivid proliferation of the dedifferentiated cells accompanies the disease.25
Recent observations in parietal epithelial cells question this view. First, it has been proposed that a niche of glomerular epithelial stem cells resides within the parietal epithelium at the transition to the proximal tubule.26,27 It is an intriguing hypothesis that proliferating stem cells from this locus may transform into podocytes and may reach the tuft via the transitions of the epithelia at the glomerular vascular pole. Migration of parietal cells onto the the vascular pole and subsequent transition into podocytes have been shown to occur in the newborn mouse.28 However, evidence that such a process may be of any relevance in the adult has so far not been presented.28 Moreover in the adult mouse, it has been shown that proliferating parietal epithelial cells may reach the glomerular tuft via tuft-capsule bridges; there they replace podocytes causing the collapse of the concerned tuft area obviously by bringing the local cross talk between podocytes and the endocapillary compartment to an end.29
The cell bodies give rise to large primary processes that may branch another time, finally splitting apart into terminal processes, called foot processes (as seen from SEM pictures, “finger processes” would be a better word) or pedicels (Figs. 1.6, 1.8,1.9,1.10,1.11, and see Fig. 1.13). The foot processes are anchored within the GBM to a depth of about 40 to 60 nm. The foot processes interdigitate in a complicated manner with those from adjacent cells to form an elaborate layer of small processes along the glomerular basement membrane. This interdigitation results in the formation of an extensive series of narrow slits between the foot processes, which provide a long extracellular path for filtration of water and solutes (Fig. 1.10). In transmission electron micrographs, these slits are bridged by a layer of extracellular material (4 to 6 nm thick) called the filtration-slit membrane (see Figs. 1.9 and 1.13). If tannic acid is added to the fixative solution, a highly ordered isoporous substructure is revealed in en face views of the filtration-slit membrane.30 Staggered rodlike units project from the podocyte plasmalemma and connect to a central linear bar. These rodlike units delineate rectangular pores 4 × 14 nm within the slit membrane (i.e., which approximate the size of an albumin molecule).
![]() FIGURE 1.9 Transmission electron micrograph showing the podocyte (P), pedicels (PC) near the basement membrane (BM), and the endothelial cells lining the capillary (C). (Magnification ×34,000.) |
![]() FIGURE 1.10 Scanning electron micrograph showing the elaborate cell shape of rat podocytes. (Magnification ×5,900.) |
Generally, the slit diaphragm is considered as an adherenslike intercellular junction.31 Intensive research in recent years has uncovered several transmembrane proteins that participate in the formation of the slit membrane (Fig. 1.11)—including P-cadherin,31 nephrin,32 Neph1,33 and FAT.34 Other molecules, such as ZO1,35 Podocin,36 CD2AP,37 and catenins mediate the connection to the actin cytoskeleton (see below). Nephrin is a member of the immunoglobin superfamily (IgCAM); its gene, NPHS1, has been identified as the gene whose mutations cause congenital nephrotic syndrome of the Finnish type.32 In addition to its role as a structural component, nephrin acts as a signaling molecule that can activate MAP kinase cascades.38 Neph1 is considered as a ligand for nephrin. Podocin belongs to the raft-associated stomatin family, whose gene, NPHS2, is mutated in a subgroup of patients with autosomal recessive steroid-resistent nephrotic syndrome.36 These patients show disease onset in early childhood and rapid progression to end-stage renal failure. Podocin interacts with nephrin and CD2AP39 FAT is a novel member of the cadherin superfamily with 34 tandem cadherinlike extracellular repeats and a molecular weight of 516 KDa.40 Because FAT has a huge extracellular domain, it is speculated that it dominates the molecular structure of the slit membrane34; the FAT mutant mouse fails to develop a slit membrane.41 P-cadherin31 is thought to mediate with its intracellular domain the linkage to β- and γ-catenin, a complex which then connects to the actin cytoskeleton via α-catenin and α-actinin. Taken together, many components of the slit membrane are known, but an integrative model of its substructure including all components is thus far lacking.
The cell body of podocytes contains a large nucleus that tends to be indented in the region of the large Golgi apparatus. Furthermore, it houses abundant rough-surface endoplasmic reticulum; individual cisternae, generally arranged in one complex per cell, are widened and filled with fine granular material of varying electrondensity—their relevance is unknown. In addition to the synthesis of membrane proteins necessary to supply the huge surface of their processes, podocytes in the adult synthesize and secrete all components of the GBM42 (see below). On the other hand, abundant multivesicular bodies (predominantly found in the large cell processes) demonstrate strong catabolic activity in podocytes.
Podocytes contain a well-developed cytoskeleton that accounts for the unique shape of the cells and the maintenance of the processes. In the cell body and the primary processes, microtubules and intermediate filaments, such as vimentin and desmin, dominate, whereas microfilaments are densely accumulated in the foot processes. Here, they are part of a complex contractile apparatus.43 The microfilaments form loop-shaped bundles, with their limbs running along the longitudinal axis of the foot processes. The bends of these loops are located centrally at the transition to the primary processes and are probably connected to the microtubules by the microtubule-associated protein τ.44 Peripherally, the actin bundles appear to be anchored in the dense cytoplasm associated with the cell membrane of the soles of foot processes, and are dynamically linked to the slit diaphragm complex (discussed earlier). The importance of the podocyte actin cytoskeleton is emphasized by the discovery of inherited forms of focal segmental glomerulosclerosis (FSGS) caused by
mutations in actin-binding proteins: α-actinin-4 is a widely expressed homodimeric protein that bundles and crosslinks actin, and is highly expressed within podocyte foot processes. It interacts with a variety of other adhesion and signalling molecules. Several mutations in the ACTN4 gene encoding this protein cause a late-onset, autosomal dominant form of kidney failure.45,46 More recently, nine independent missense mutations in INF2, which encodes a member of the formin family of actin-regulating proteins, were shown to segregate with FSGS in 11 unrelated families.47
mutations in actin-binding proteins: α-actinin-4 is a widely expressed homodimeric protein that bundles and crosslinks actin, and is highly expressed within podocyte foot processes. It interacts with a variety of other adhesion and signalling molecules. Several mutations in the ACTN4 gene encoding this protein cause a late-onset, autosomal dominant form of kidney failure.45,46 More recently, nine independent missense mutations in INF2, which encodes a member of the formin family of actin-regulating proteins, were shown to segregate with FSGS in 11 unrelated families.47
Specific transmembrane matrix receptors anchor the podocyte foot processes to the GBM. Two systems are so far known. The first is a specific integrin heterodimer consisting of α3β1 integrins. Within the GBM, these integrins bind to collagen type IV, fibronectin, and laminin 11.48,49 Second, a dystroglycan complex connects the intracellular molecule utrophin to laminin 11, agrin, and perlecan in the GBM.50,51 Both integrins and dystroglycans are coupled via adapter molecules (paxillin, vinculin, α-actinin, etc.) to the podocyte cytoskeleton, allowing outside-in and inside-out signaling as well as transmission of mechanical force in both directions.
In addition to the actin cytoskeleton within the foot processes, a subplasmalemmal actin system is found in podocytes.43 This actin network is connected to the transmembrane sialoprotein podocalyxin, which represents the major protein of the negatively charged surface coat of podocytes.52,53 The cell coat has the characteristics of a glycocalyx that contains sialic acid. A decrease in the content of sialic acid is associated with a podocyte foot process effacement and results in protein leakage through the filter, which has been shown under a great variety of circumstances.54,55,56,57,58
A huge body of data has been accumulated in recent years concerning the inventory of receptors and signaling processes starting from them in podocytes. cGMP signaling (stimulated by atrial natriuretic peptide [ANP], brain natriuretic peptide [BNP], and C-type natriuretic peptide [CNP], as well as by nitric oxide (NO]), cAMP signaling (stimulated by prostaglandin E2, dopamine, isoproterenol, parathyroid hormone [PTH]/PTH-related peptide (PTHrP]), and Ca2+ signaling (stimulated by a huge number of ligands, including angiotensin II, acetylcholine, prostaglandin F2 (PGF2), arginine vasopressin [AVP], adenosine triophosphate [ATP], endothelin, histamine, etc.) have been identified.59 An example of an ion channel of particular importance in podocytes is transient receptor potential canonical 6 (TRPC6), a nonselective cation channel, which is activated by diacylglycerol in a protein kinase C-dependent manner.60 Mutations in TRPC6 were found to cause autosomal dominant, late adult-onset proteinuria, with a similar clinical phenotype as seen in ACTN4-mediated FSGS.37 The major target of this signaling orchestra is the cytoskeleton, the concrete effects of which, however, are poorly understood. Other receptors, such as for C3b,61 Heymann’s antigen,62 transforming growth factor β (TGFβ),63,64 fibroblast growth factor 2 (FGF2),65 and various other cytokines and chemokines have been shown to be involved in the development of podocyte diseases.59
Endothelium
The endothelium consists of a simple squamous layer of fenestrated (porous) cells with the cell nuclei generally located near the axial region of the capillary loop (Fig. 1.6). The fenestrated regions (Fig. 1.12), which compose roughly 55% of the surface area,66 have a thin layer of cytoplasm (about 50 nm thick) penetrated by numerous fenestrae of round, oval, or irregular shape and varying sizes. The total area occupied by fenestrae accounts for 13% of the capillary surface. The fenestrated regions outline the pericapillary portions of the glomerular basement membrane, but also may be found adjacent to the mesangium (Fig. 1.8). The fenestrae have a diameter of 50 to 100 nm (thus they are larger than endothelial fenestrae elsewhere in the body) and are not bridged by diaphragms. Thus, this kind of a porous endothelium is unique for glomerular capillaries. Fenestrae with diaphragms are found only in the outflow segment of the efferent arteriole.67 Nonfenestrated regions are generally seen over nuclei and mesangial cell regions. Human glomerular endothelial cells also are fenestrated.68,69 A cell coat that is rich with polyanionic glycoproteins, including podocalyxin, covers the endothelial surface70,71,72 and appears to fill the fenstraelike “sieve plugs.”73 In addition, above this “classic” glycocalix a 200-nm thick endothelial surface layer has been revealed consisting of loosely attached plasma compounds.74
![]() FIGURE 1.12 Scanning electron micrograph of a sectioned capillary loop showing the pores (arrows) of the endothelium. (Magnification ×50,400.) |
As elsewhere in the body, glomerular endothelial cells are active participants in processes controlling coagulation, inflammation, and immune processes. Renal endothelial cells express surface antigens of the class 2 histocompatibility complex. Like platelets, glomerular endothelial cells contain components of the coagulation pathway and are capable of binding factors IXa and Xa and synthesize, release, and bind von Willebrand factor (factor VIII).69 Glomerular endothelial cells synthesize and release endothelin-1 and endotheliumderived relaxing factor (EDRF).75 Glomerular endothelial cells have receptors for vascular endothelial growth factor (VEGF) and angiopoetin I that are produced by podocytes.76,77 The signaling axis via VEGF appears to have a major relevance for the maintenance of the glomerular tuft. Glomerular endothelial cells, in turn, synthesize and secrete platelet-derived growth factor β (PDGFβ) that acts on adjacent mesangial cells.78
Glomerular Basement Membrane
The GBM covers the capillary loops except in the axial region, where it is reflected over the mesangium to the next capillary loop, accompanied by the layer of foot processes (Figs. 1.6,1.7,1.8). The endothelial cells do not have a separate basement membrane; thus, the endothelial cells directly abut the mesangium toward axial regions. In adult humans, the basement membrane has a mean diameter of 320 to 340 µm.79 It is thinner in young children and most laboratory animals.80
The basement membrane is composed of three layers: an outer, less dense subepithelial layer, the lamina rara externa; a central, electron-dense layer, the lamina densa ; and an inner subendothelial layer, the lamina rara interna, which is continuous with the mesangial matrix (see Figs. 1.9 and 1.13).
During glomerulogenesis, the GBM is generated as two separate layers produced by glomerular endothelial and epithelial cells. The two sheets are fused together to form the mature GBM.81,82,83,84 In the adult, the GBM is subject to a continuous turnover,85,86,87,88 but few details are known so far about these processes. Podocytes appear to be the dominant cell type to synthesize and probably to degrade the GBM. Podocytes are alone capable to synthesize all the components of the GBM42,89,90; glomerular endothelial cells and also mesangial cells may contribute to the formation of the GBM.91 It is less clear how the GBM degrades. In recent years, several extracellular matrix degrading enzymes have been described being produced by podocytes and mesangial cells.92,93,94 The relevance of these enzymes for the turnover of the GBM remains to be established.
The GBM is generally considered as a hydrated meshwork consisting of collagen type IV, laminin, entactin/nidogen, and sulfated proteoglycans including agrin and perlecan.42,95,96,97,98,99 Models of the ultramicroscopic structure of the basement membrane picture the GBM as a mat of collagen type IV. Monomers of type IV collagen consist of a 400-µm triple helix that, at its carboxy-terminal end, has a large noncollagenous globular domain called NC 1. At the aminoterminus the helix possesses a 60-µm triple helical rod, the 7S domain. Interactions between the 7S domain and the NC1 domain allow collagen type IV monomers to form tetramers that, by lateral association of triple helical strands, assemble into a three-dimensional network.100,101 Laminin forms a second network that is superimposed to the collagenous network. Laminin is a noncollagenous glycoprotein consisting of three polypeptide chains, two of which are glycosylated and cross-linked by disulfide bridges.95,102,103 Laminin binds to specific sites on the polymerized network of type IV collagen as well as the basal endothelial and epithelial integrins (see the preceding). The α-5-, β-2-, γ-1-laminin chains are assembled to form the GBM-specific heterotrimeric laminin 11.48 This combined network of collagen type IV and laminin provides mechanical stability to the basement membrane and serves as a basic structure on which other matrix components attach.
The proteoglycans of the basement membrane consist of core proteins and covalently bound glycosaminoglycans, which are concentrated in the laminae rarae internae and externae, where they have been referred to as anionic sites and can be localized with cationic probes.104 The major proteoglycans of the GBM are of the heparan sulfate type—the most prominent is agrin,96 but perlecan also has been shown to occur in the GBM.42 Digestion of these molecules with heparinase leads to a dramatic increase in the permeability of the basement membrane to anionic native ferritin used as a probe.70
Mesangium
The mesangium consists of mesangial cells that are embedded in a mesangial matrix. The term mesangium was introduced by Zimmermann in 192919 to describe the cells that form the stalk of the glomerulus and the axes of its lobules. Glomerular capillaries pursue a tortuous, highly anastomosing course around the mesangial axes. Together with the capillaries, the mesangium occupies the space inside the GBM, frequently termed the “endocapillary region.” Topographically, the mesangium can be subdivided into a juxtacapillary region, where it abuts the capillary endothelium, and more centrally located axial regions, which are bound by the perimesangial GBM (Figs. 1.5,1.6,1.7).105 The glomerular mesangium is continuous with the extraglomerular mesangium (Polkissen or lacis cells) along the glomerular stalk (Fig. 1.5). Both intraglomerular and extraglomerular mesangial cells have many similarities.
Mesangial cells are quite irregular in shape, with many cytoplasmic processes extending from the cell body toward the GBM. They have structural characteristics similar to those of smooth muscle cells in that they contain many bundles of microfilaments (especially within the cell processes) and peripheral dense bodies. Actin, myosin, and α-actin have been shown by immunocytochemistry to be contained in mesangial cells.106,107 The relevance of mesangial cell contractility is discussed in the following text.
The processes of mesangial cells extend toward the GBM, to which they are attached either directly or by the interposition of extracellular bundles of microfibrils (Figs. 1.6 and 1.9). The GBM has to be considered as the effector structure of mesangial contractility.105,108 Connections between mesangial cells and the GBM are especially prominent in the juxtacapillary region. At this site, tonguelike mesangial cell processes (packed with microfilament bundles) run underneath the capillary endothelium toward the turning points (mesangial angles) of the GBM, where they are anchored. Generally, two of these processes interconnect the GBM from two opposing mesangial angles (Fig. 1.8). In the axial mesangial region, contractile filament bundles are predominantly found within the numerous fingerlike projections of mesangial cells. These microprojections also run toward the GBM and are anchored to it. As in the juxtacapillary region, these microfilament bundles interconnect opposing parts of the GBM.105
The mesangial matrix fills the highly irregular spaces between mesangial cells and the perimesangial part of the GBM. In immunocytochemical studies, collagen types IV and V, heparan sulfate proteoglycan, fibronectin, laminin, and entactin have been localized within the mesangial matrix.99,109,110 Among these components, fibronectin is the most abundant and has been shown to be associated with microfibrils.109,111 Fibrillin 1 and other specific elastic fiber proteins have been detected in the glomerular mesangium and have been shown to be produced by mesangial cells.112,113
In specimens prepared for transmission electron microscopy according to routine methods, the mesangial matrix appears as basement membranelike material, albeit more fibrillar in character than the basement membrane proper.114 In specimens prepared by a technique that avoids osmium tetroxide and uses tannic acid for staining, the mesangial matrix is seen to contain a dense network of microfibrils.105,115 Microfibrils are noncollagenous, nonbranching, hollow structures of indeterminate length that are about 15 µm thick.116 Within the mesangium, microfibrils form a three-dimensional network that establishes a solid base of contact between mesangial cells and the GBM, fettering the GBM to mesangial cells. Distinct bundles of microfibrils may be regarded as “microtendons” that allow the transmission of contractile force of mesangial cells to specific sites of the GBM.105,115 The functional relevance of this system is discussed later.
The relevance of mesangial cells in phagocytosis is well documented. Mesangial cells are able to ingest particular tracers as well as macromolecules, such as thorotrast,117 ferritin,114 and aggregated proteins, as well as immune complexes.118 An increased uptake of such materials by mesangial cells has been noted in proteinuric states.114,119 It appears, however, that mesangial cells proper (i.e., mesangial cells that have contractile properties) are not primarily phagocytotic. A small subpopulation (3% to 7%) of cells in this region has been recognized as bone marrowderived—they represent macrophages that have taken up residence in the mesangium.120
Supportive Functions of the Mesangium and Podocytes
The glomerular tuft is constantly exposed to comparably high intraglomerular pressures within glomerular capillaries and mesangium. The high intraglomerular pressures challenge not only the glomerular capillaries themselves but also the folding pattern of the glomerular tuft. Increased pressures lead to the loss of the folding pattern and to dilation of the glomerular capillaries. Therefore, we have to ask: what are the specific structures and mechanisms that counteract the expansile forces in the glomerular tuft? To answer this question we have to distinguish between the structures and mechanisms maintaining (1) the folding pattern of the glomerular tuft and those maintaining (2) the width of glomerular capillaries.
The folding pattern of the glomerular tuft is primarily sustained by the mesangium.105,108,121 Mesangial cells are connected to the GBM by their contractile processes—they maintain the infoldings of the GBM by centripetal contractions, thereby allowing for the capillaries to arrange in the peripheral expansion of the GBM. This supporting role of mesangial cells is best illustrated under circumstances with loss of mesangial cells, such as Thy-1 nephritis.122 Under those circumstances the folding pattern of the GBM is progressively lost, finally resulting in mesangial aneurysms. Podocytes clearly contribute to maintenance of the folding pattern by specific cell processes that interconnect opposing parts of the GBM from outside within the niches of the infoldings. This function is also best illustrated under circumstances with loss of mesangial support: podocytes are capable of maintaining a high degree of the GBM folding pattern for 2 to 4 days after which they obviously fail, and mesangial aneurysms become prominent.122
The width of glomerular capillaries, in the long run, is probably controlled by growth processes accounting for differently sized capillaries. The width of a given capillary, in an acute situation being exposed to changes in blood pressure, appears to be stabilized by the GBM, which is a strong elastic structure123 and, together with the mesangial cell bridges (see previous text), capable of developing wall tension.121,124 In addition, the tensile strength of the GBM is reinforced by podocytes. Podocytes are a kind of pericyte; their foot processes represent a unique type of pericyte process which, like elsewhere in the body, counteract the dilation of the vessel. Podocyte processes are firmly attached to the underlying GBM (see previous text); their cytoskeletal tonus counteracts the elastic extension of the GBM. In this function, podocytes cannot be replaced by any other cell—failure in this function will lead to capillary dilation.
Glomerular Filtration Barrier
The essential components of the glomerular filtration barrier are the endothelium (Fig. 1.13), which is perforated by large open pores, the extracellular matrix feltwork of the GBM membrane, and the slit diaphragms between the podocyte
foot processes. When compared with the barrier established in capillaries elsewhere in the body, a glomerular filtration barrier is quite different in two respects: its permeability to water, small solutes, and ions is extremely high, whereas its permeability to plasma proteins the size of albumin and larger is very low.
foot processes. When compared with the barrier established in capillaries elsewhere in the body, a glomerular filtration barrier is quite different in two respects: its permeability to water, small solutes, and ions is extremely high, whereas its permeability to plasma proteins the size of albumin and larger is very low.
The high hydraulic permeability is rooted in the fact that filtration occurs along extracellular routes. All components of this route—the endothelial pores, the highly hydrated GBM, and the slit membrane—can be expected to be quite permeable to water and small solutes. Drummond and Deen125 have calculated the hydraulic conductance of the individual layers. According to this calculation, the hydraulic resistance of the endothelium is negligible. The GBM and the filtration slits each make up roughly one-half of the total hydraulic resistance of the filtration barrier.
Any decrease in the length of the filtration slit, and thus in slit area, as in experimental and clinical glomerulopathies along with footprocess effacement, correlates with the decrease in the ultrafiltration coefficient Kf.126,127 A model simulating those conditions showed, along with a decrease in slit area, its relevance in determining increases in flow resistance. The decrease in filtration slit area caused the average path length for the filtrate, through the basement membrane, to increase, thereby explaining the overall decreased hydraulic permeability.128
On the basis of evidence of contractility of mesangial cells exposed to vasoconstrictor stimuli in culture,129,130 of dimensional changes observed in intact glomeruli ex vivo,131,132 and of changes in ultrafiltration coefficient Kf found in vivo in response to vasoactive substances,133,134 some researchers have concluded that mesangial cells contract in situ and that this contraction alters glomerular filtration dynamics by decreasing filtration surface area.
Other considerations speak against this possibility. The geometric arrangement of the mesangial contractile apparatus (Fig. 1.6), however, does not seem to be compatible with the previously mentioned sequence of actions. Shortening of the mesangial cell processes connecting opposing angles would only bring the angles closer together, compressing the mesangial capillary interface, but leaving peripheral capillary wall area (filtration area) unaltered. In addition, with regard to the contractility of mesangial cells ex vivo (in culture as well as preparations of whole glomeruli), it should be remembered that mesangial contraction in these cases is not opposed by intercapillary hydrostatic pressure as it is in situ. These considerations, together with the absence of measurable changes in glomerular tuft dimensions in morphometric studies,135,136 as well as in response to vasoactive substances in vivo,137 have led to the suggestion121 that the mechanical action of the mesangial cell contraction is essentially static in nature, developing tension that serves to counteract expansile forces on the tuft without inducing significant changes in capillary dimension. If mesangial contractility acutely alters the glomerular ultrafiltration coefficient Kf, it is, therefore, probably not because of an acute change in filtration surface area.
The low permeability of the glomerular filtration barrier to plasma proteins is still poorly understood. Several points seem to be relevant. First, there is no vesicular transport of proteins through this barrier as in most other capillaries elsewhere in the body. The barrier function of the glomerular filter for macromolecules is quite specific and is selective for size, shape, and charge.138,139,140
In early extensive studies, Farquhar and associates,138,141,142 as well as Rennke and associates,143 used tracers, such as ferritin and dextrans of different sizes and charge, to elucidate the role of the various layers in determining the selectivity of this filtration barrier. When their results are summarized, it appears that the basement membrane may be the major barrier to anionic substances, whereas the most restrictive part for uncharged and cationic substances may be the slit diaphragm. Uncharged macromolecules up to an effective radius of 1.8 µm pass freely through the filter. Larger compounds are more and more restricted (indicated by their fractional clearances, which progressively decrease) and are totally restricted at effective radii of more than 4.0 µm. The effective radius is an empiric value, measured in artificial membranes, that takes into account the shape of micromolecules and also attributes a radius to nonspherical molecules. Plasma albumin has an effective radius of 3.6 µm; without the repulsion because
of the negative charge, plasma albumin would pass through the filter in considerable amounts.144
of the negative charge, plasma albumin would pass through the filter in considerable amounts.144
Thus, since these early studies, the glomerular barrier has been generally suggested to contain a size- and a chargerestrictive element. Despite more than 40 years of intensive research, the principles of the glomerular barrier function are still controversial and poorly understood—a situation that gives room even to hypotheses claiming that the glomerular barrier itself does not have any restrictive properties to macromolecules, such as albumin.145,146,147 A more realistic and elegant recent barrier hypothesis is based on the observation that filtration—the flow of filtrate through the barrier—creates a potential difference of 0.02 to 0.05 mV negative in Bowman’s space and that this potential difference is sufficient to hinder the negatively charged macromolecules like albumin from passing the filter.148
Summarizing the present status of knowledge, accumulating evidence suggest that the GBM has little relevance in size restriction, but the slit membrane is the decisive size-restrictive element within the glomerular barrier.149 The current strong engagement in elucidating the molecular architecture of the slit membrane promises to enlighten the porous pattern of the slit membrane and, hopefully, explain its size-restrictive property. Regarding the charge selectivity, it appears that the proximal portion of the barrier, above all the endothelium, are most important.73,150,151,152 Furthermore, prevention of albumin from entering the filter is also dependant on normal hemodynamic conditions in glomerular capillaries, as first shown by Ryan and Karnovsky.153
PROXIMAL TUBULE
At the urinary pole of the glomerulus, the flat parietal epithelium of Bowman’s capsule transforms abruptly into the high epithelium of the proximal tubule. In some species (rabbits),154 a neck segment is found interposed between the glomerulus and the proximal tubule—short neck segments also may be seen in humans.80 In contrast, in mice, the proximal tubule epithelium generally begins deep within the Bowman’s capsule.
The proximal tubule is composed of segments that have differing morphology, functional relevance, and vulnerability to toxins (Figs. 1.14,1.15,1.16,1.17,1.18). The two segments most frequently identified are the proximal convoluted portion (pars convoluta) occupying the cortical labyrinth (Figs. 1.14,1.15,1.16,1.17), and the straight portion (pars recta) in the medullary rays of the cortex and the outer stripe of the outer medulla (Fig. 1.18). Further subdivision on structural criteria results in the three segments of P1, P2, and P3 according to Jacobsen and Jorgensen,155 and S1, S2, and S3 according to Maunsbach.156,157 S1, or P1, corresponds to the first segment of the pars convoluta and lies exclusively in the cortical labyrinth; S2, or P2, corresponds to the remainder of the convoluted segment and the beginning of the pars recta, with the first part occupying the cortical labyrinth and the remainder occupying the medullary ray; and S3, or P3, corresponds to the remaining part of the pars recta located primarily in the outer stripe of the outer zone of the renal medulla. The transition from P1 to P2 is gradual, whereas the transition from P2 to P3 is generally abrupt, except in rabbits.158
To confuse matters further, the morphology of the subdivisions differs not only from each other but also among species, including mice,159 rats,156 rhesus monkeys,160 rabbits,158 and dogs.161 For a detailed description of a particular species, the reader should consult the original studies. Segmentation of human proximal tubules has not been studied as recently or thoroughly because of a lack of availability of well-fixed normal human kidney and, therefore, has been divided only into convoluted and straight regions.162
In general, cells of the P1 region are tall, have a welldeveloped apical microvillus border, an elaborate cell shape with well-developed lateral interdigitating processes containing abundant, large mitochondria, and a well-developed endocytic apparatus (Fig. 1.16). P2 cells decrease in cell height from those seen in P1, have a shorter microvillus border, and are less elaborately shaped cells with smaller mitochondria (Fig. 1.17). The P3 cells are more cuboidal in shape (less elaborate) and their microvillus border is generally less elaborate. The cells of rats are an exception and have a well-developed brush border of very long microvilli (Fig. 1.18).156 This cytologic segmentation of the proximal tubule is maintained in superficial, midcortical, and juxtamedullary nephrons.
![]() FIGURE 1.16 Transmission electron micrograph of a P1 segment from a rat kidney. (Magnification ×8,000.) |
![]() FIGURE 1.17 Transmission electron micrograph of a P2 segment from a rat kidney. Note the large dense lysosomes (L) and peroxisomes (P). (Magnification × 10,000.) |
![]() FIGURE 1.18 Transmission electron micrograph of a P3 segment from a rat kidney showing the extensive microvillus border (BB) and more simple cell shape of this segment. (Magnification ×8,000.) |
Proximal Convoluted Tubule (P1 and Part of P2)
The proximal convoluted tubule is the longest and largest segment of the mammalian nephron. The tubule is lined by cells that have an elaborate cell shape based on extensive basolateral interdigitations (Figs. 1.19,1.20,1.21), a well-developed microvillus border, a prominent intracellular digestive tract (endocytic apparatus and lysosomes (Fig. 1.15), and numerous large peroxisomes (microbodies). The single ovoid nucleus lies in the middle to basal region of the cytoplasm.
Cell Shape and Mitochondria
The cells are characterized by an extensive system of lateral cell processes that interdigitate with lateral processes from adjacent cells (Figs. 1.19,1.20,1.21). These lateral processes can extend the entire height of the epithelium, especially in the P1 segment, but become more elaborate toward the basal regions of the lateral surface (Fig. 1.16). The complex shape of these cells serves to increase the lateral cell surface area manyfold, which provides a greater area for transporters, above all of the Na+-K+-ATPase. These lateral extensions usually contain one or two layers of mitochondria (Fig. 1.20). The mitochondria are long, narrow rods that branch and double back on themselves. They are oriented perpendicular to the basement membrane and lie adjacent to the cell membrane, to which they presumably supply energy for transport processes.
The lateral extensions and their mitochondria cause the pattern of basal striations that are typical for numerous transporting cells. The lateral processes of proximal convoluted tubule cells in humans are not as elaborate as those seen in rats (compare Figs. 1.15 and 1.16). In rabbits, the lateral cell surface is increased 20 times over that of the basal cell surface.163 The lateral extensions also establish a complex and extensive surface labyrinth of lateral intercellular spaces (“basal labyrinth”).
![]() FIGURE 1.19 Scanning electron micrograph of a rat proximal tubule epithelial cell showing the apical brush border (BB) and the lateral processes (LP). (Magnification × 14,700.) |
Cell Junctions
The proximal convoluted tubular cells have an apical junctional complex that consists of a shallow, beltlike, tight junction next to the tubular lumen; a deeper, beltlike intermediate junction (the zonula adherens); and only small and infrequently seen desmosomes. The proximal tubular tight junctions are shallow and consist of only one or two lines of fusion of the outer leaflet of the cell membrane (Fig. 1.22). Freeze-fracture studies of normal proximal tight junctions, however, reveal focal discontinuities. During volume expansion, striking increases in the length of discontinuities were found.164 Transmission micrographs also show multiple areas of nonfusion of tight junctions associated with renal venous constriction and increased ureteral pressure.165 These sites of nonfusion may explain the increased permeability seen in these situations. Proximal tubular cells are electrically coupled by gap junctions (Fig. 1.22).166
Microvilli
A layer of slender (approximately 80 to 90 µm), fingershaped processes extends into the tubular lumen, forming the microvillus, or brush border. The length and number of processes vary with the segment and species. The luminal surface of the pars convoluta is increased 40 times in rats.157 The luminal surface is increased 36 times in the pars convoluta and 15 times in the pars recta in rabbits.163 Each microvillus has a core of microfilaments that extend into the apical cytoplasm connecting them to the cytoskeleton.
Cytoplasm
The cells contain a very prominent vacuolar apparatus (see later text), a Golgi apparatus, free ribosomes, and cisternae of rough and smooth surface endoplasmic reticulum. The latter forms specialized cisternae, called the perimembranous cisternal system, near the lateral cell membranes.80 In addition, the cells contain many peroxisomes that frequently have eyecatching shapes.167 Peroxisomes are limited by a single membrane and contain a dense matrix, frequently exhibiting crystalloid nucleoids (Fig. 1.17); platelike inclusions at the peroxisomal edge, which are called marginal plates, are also frequently encountered. Peroxisomes are invariably wrapped by elements of the smooth-surfaced endoplasmic reticulum. In the kidney, large peroxisomes are exclusively found in the proximal tubule, most frequently in the P3 segments167 —all other nephron portions contain only microperoxisomes. Peroxisomes contain enzymes from a primitive respiratory chain in which oxidases produce hydrogen peroxide, which is, in turn, destroyed by the catalase, hence the name peroxisome.168 Peroxisomes participate in the breakdown of very-long-chain fatty acids by lipid β-oxidation; in addition, they may play a protective role by destroying hydrogen peroxide produced by free radicals.
Straight Part of the Proximal Tubule (Last Part of P2 and All of P3)
The straight part of the proximal tubule (pars recta) begins in the medullary ray and penetrates into the outer stripe of the outer zone of the medulla (Fig. 1.18). The proximal pars recta converts into the thin limb near the junction of the inner and outer stripe. In rats, the pars recta includes the final region of both P2 and P3,169 and the transition
between the two segments occurs at various levels in the medullary ray. This region is marked by a sudden increase in microvillar length, a decrease in endocytic apparatus, and a decrease in interdigitation between adjacent cells. The microvilli of the pars recta cells decrease in height and number in rabbits158 and humans162 but remain high in rats (Fig. 1.18).
between the two segments occurs at various levels in the medullary ray. This region is marked by a sudden increase in microvillar length, a decrease in endocytic apparatus, and a decrease in interdigitation between adjacent cells. The microvilli of the pars recta cells decrease in height and number in rabbits158 and humans162 but remain high in rats (Fig. 1.18).
In general, the P3 pars recta cells have been described as lower in height with a less elaborate shape. In humans, the pars recta cells have a convex apical surface, and some lipid droplets are found in the basal cytoplasm. The mitochondria are fewer and no longer closely applied to the cell membrane. The lysosomes are smaller and the Golgi and endocytic apparatuses are less well developed. Peroxisomes are more numerous in the pars recta.80,162 The tight junctions of P3 can be more complex in shape, consisting of several junctional strands in rats, dogs, and cats.170
Structure-Function Correlations
The functional relevance of the proximal tubule is manifold and quantitatively enormous. It reabsorbs about 70% of filtered Na+, Cl+, and water; 95% of bicarbonate; 60% of Ca2+; and sodium phosphate. Reabsorption of the filtered glucose is complete, whereas reabsorption of amino acids is almost complete. This occurs either by transcellular transport via specific channels and transporters in both membranes (water, sodium, phosphate, glucose, amino acids, and bicarbonate in a specific enzyme-mediated mode) or predominantly by paracellular transport through the leaky tight junctions (Ca2+, Cl+). Furthermore, filtered macromolecules undergo reuptake by a prominently developed endocytotic apparatus. In addition, the proximal tubule (predominantly S3 segments) secretes organic cations and anions, which constitute an extraordinarily diverse array of compounds of physiologic, pharmacologic, and toxicologic importance. The transepithelial transport involves separate entry steps at the basolateral membrane and exit steps at the luminal membrane with specific transporters at both sites.171,172,173,174 Other potentially toxic xenobiotic compounds are metabolized within the well-developed smooth endoplasmic reticulum of S3 segments.175
Unique to the proximal tubule is the reabsorption and degradation of filtered protein and peptides (Figs. 1.15 and 1.16). As discussed previously, all proteins smaller than albumin leak through the glomerular filter; even albumin, in small amounts, is always contained in the filtrate. All these diverse macromolecules are taken up by proximal tubule cells via receptor-mediated endocytosis and degraded in lysosomes. In proteinuric states, this function is greatly enhanced. The uptake and digestion include the following steps.176,177
1. Binding of the filtered protein to two multiligand receptors, megalin and cubulin, which are densely accumulated within clathrin-coated pits in the intermicrovillar areas of the apical membrane.
Megalin is a 600-kDa transmembrane protein belonging to the LDL-receptor family (see reviews).176,177 The extracellular domain contains four clusters of cysteine-rich, complement-type repeats, constituting the ligand-binding regions. Cubulin is a 416-kDa peripheral membrane protein identical to the intrinsic factor vitamin B12 receptor, known from the small intestine. It contains 27 CUB domains, which are responsible for the ability to interact with a great variety of ligands.
2. Small apical vesicals pinch off from the tubular invagination at the intermicrovillar areas to ferry the protein to the next component.
3. Large apical vacuoles located in the apical cytoplasm are formed by fusion of small vesicles representing the early endocytotic compartment.
4. Condensing vacuoles form in which the protein is condensed. These vacuoles move basally in the cell and acquire hydrolytic enzymes by fusion either with primary or secondary lysosomes. The proteins sequestered within lysosomes appear to be broken down into amino acids that are reused by the cell.
5. Already sequestered in the early endosomal compartment, the receptors are concentrated in dense, apical tubules by which they are returned to the apical plasma membrane.
This process of megalin-mediated internalization appears to be of even much wider relevance. As mentioned previously, the proximal tubule reabsorbs phosphate, representing a key element in phosphate homeostasis. Depending on the amount of sodium phosphate cotransporters present in the brush border cell membrane, the reabsorption varies. Internalization of the phosphate transporter type II (e.g., PTH induced to downregulate reabsorption) occurs via megalin-mediated endocytosis.178,179 The proximal tubule has also endocrine relevance. In response to stimulation by PTH, it produces, by adding a second hydroxyl group, the active vitamin D3, calcitriol.
Thin Limbs of the Loop of Henle (Intermediate Tubule)
Thin limbs can be short, occurring only along the descending limb, or they can be long, reaching varying distances into the inner medulla. In the long-looped variety, thin limb segments compose part of both the descending and ascending limbs. Four types of thin limb segments are routinely identified: (1) descending thin limbs of short-looped nephrons (SDTL) (Figs. 1.23,1.24,1.25), (2) upper portions of descending thin limbs of long-looped nephrons (LDTL up), (3) lower portions of descending thin limbs of long-looped nephrons (LDTL lp), and (4) ascending thin limbs of long-looped nephrons (ATL) (Figs. 1.24 and 1.25). This pattern has been observed in rats,180,181 mice,182 syric hamsters,183 the desert rodent Psammomys obesus,184,185 and the rabbit.158 Thin limbs have not been studied as carefully in humans as
in several other animal species.13,186,187 An additional subsegment of thin limbs has been identified in chinchillas.188
in several other animal species.13,186,187 An additional subsegment of thin limbs has been identified in chinchillas.188
Surprisingly, as seen by light microscopy, these simple looking epithelia are strikingly different from each other— not only the ascending from the descending limbs but, most remarkably, the descending limbs of short from those of long loops. Furthermore, within the descending segments, the proximal portion, although structurally not different from the distal portion, express a different pattern of transporters than the distal portion. Even islets or interposed limb pieces of functionally different cells are encountered within an otherwise homogenous thin limb segment.189
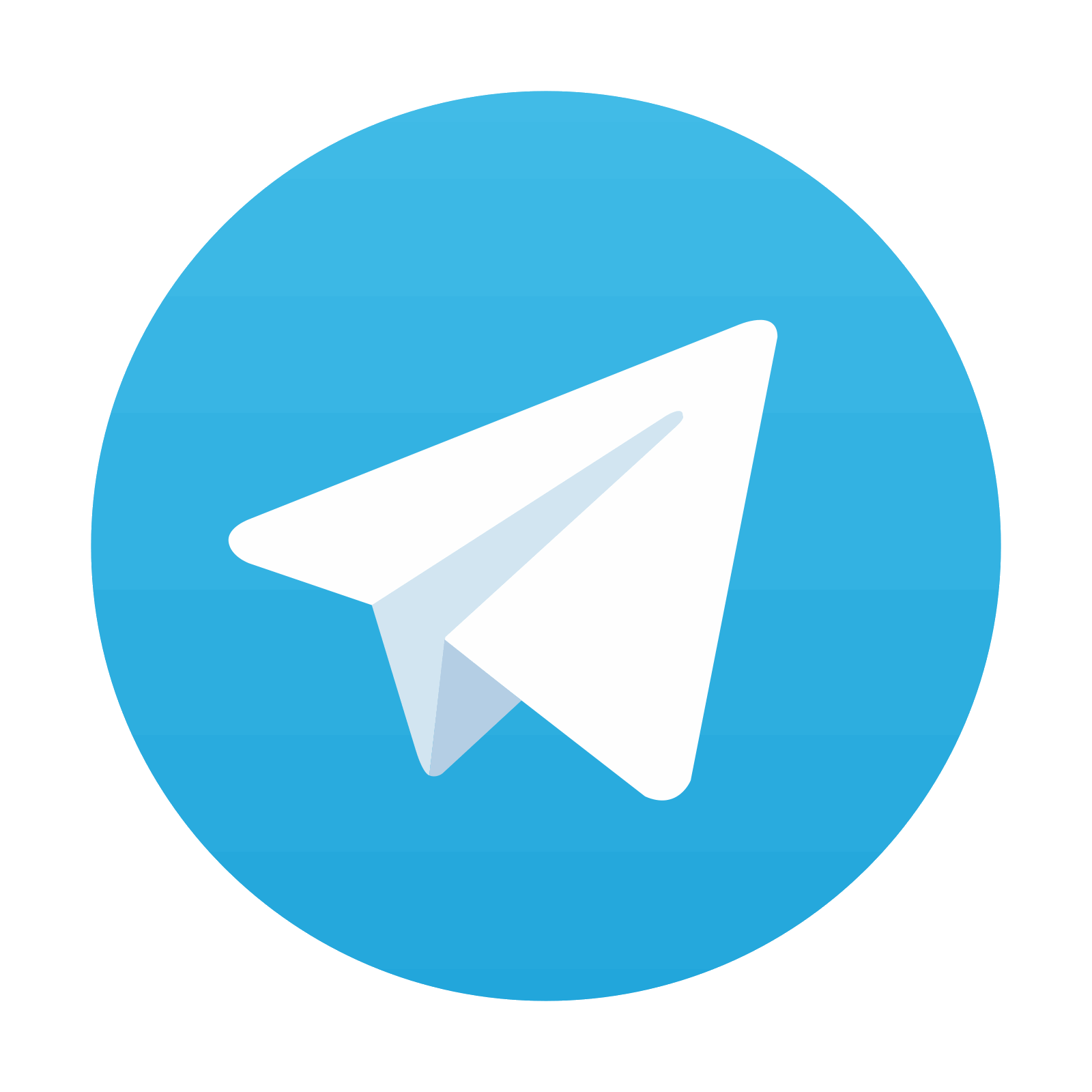
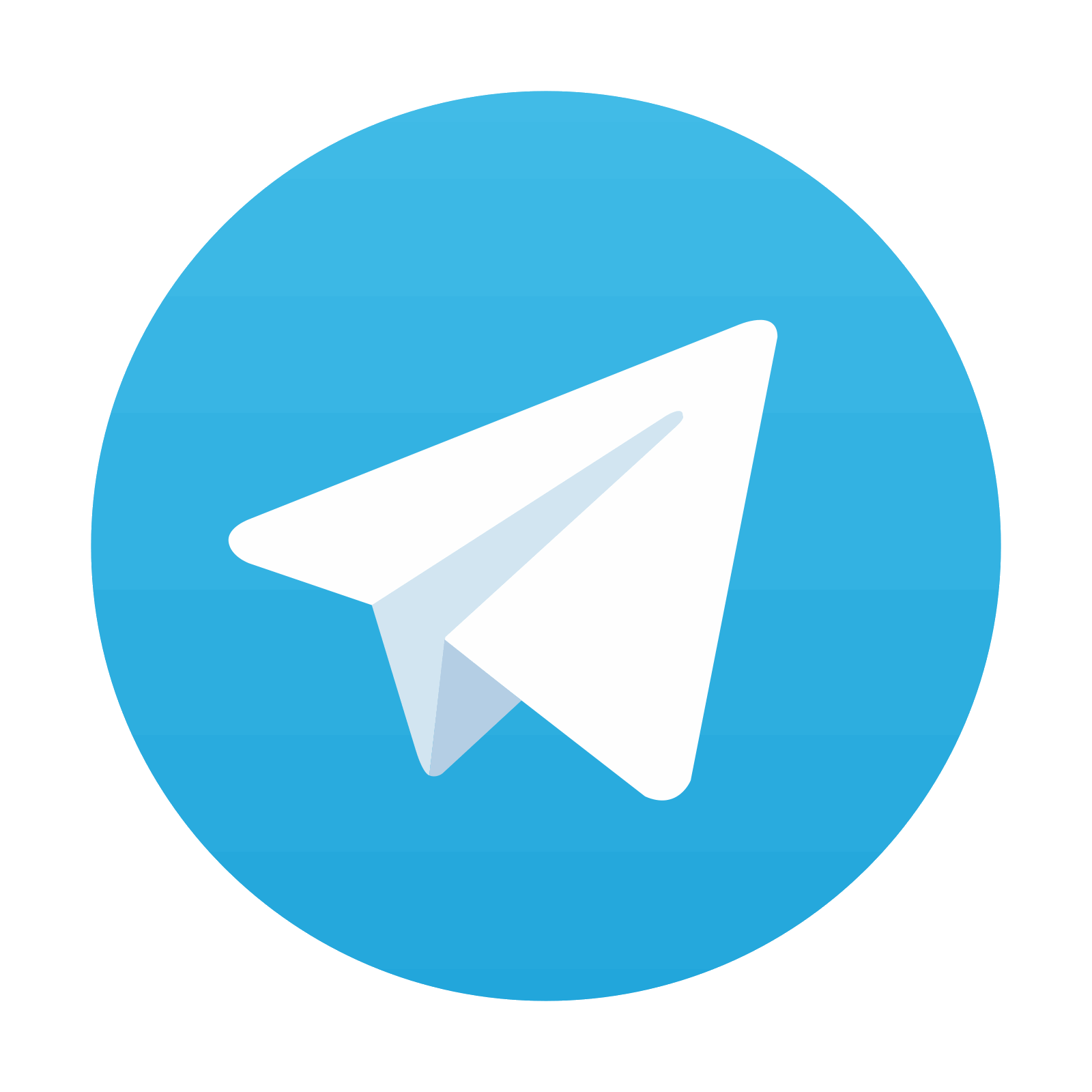
Stay updated, free articles. Join our Telegram channel
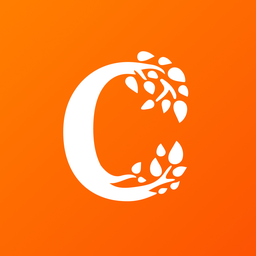
Full access? Get Clinical Tree
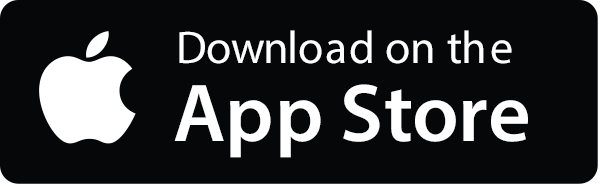
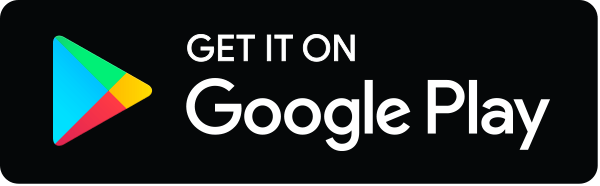
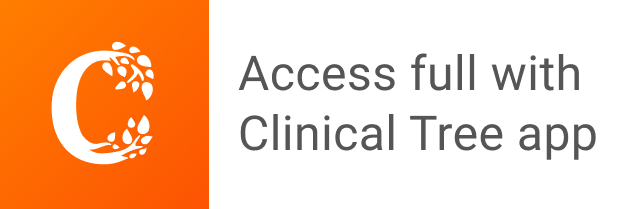