Regulation of Water Balance: Urine Concentration and Dilution
Yumi Noda
Sei Sakaki
Water is by far the largest component of the body and accounts for approximately 50% to 65% of body weight. Maintenance of body fluid homeostasis, including fluid volume and solute concentration, is essential for cell function and whole-organism survival. The osmolality of body fluid, a concentration of all of the solute in water, is kept within a remarkably narrow range (280 to 295 mOsm per kilogram of water), in spite of large fluctuations of solute and water intakes and losses. Although this constancy is made by a variety of regulatory mechanisms in the body, the most critical regulatory capacities are provided by the kidney’s urine concentration and dilution mechanisms.
Body water homeostasis is maintained by the balance between the input and output of water. Each side has regulated and unregulated components. The regulated component of water input is oral intake of fluids in response to a perceived sensation of thirst. The unregulated components of water input are oral intake of liquids and water in foods, and metabolic water of oxidation. Oral intake of water usually varies enormously in excess of homeostatic need because of social, cultural, or psychological influences. Solute-free water excretion by the kidney is the only route of regulated water output. The unregulated component of water excretion occurs via various kinds of insensible water losses including sweat, evaporative loss, gastrointestinal loss, and the obligate amount of water that is required to excrete the solutes in the urine. Sweat volume is determined by the requirements of temperature regulation. Evaporative loss is determined by body temperature and surface area, ventilation, and environmental temperature and humidity. Gastrointestinal water loss is affected by disturbance of its function. Both the input and output of water have very substantial unregulated components, and these can vary tremendously as a result of factors that are unrelated to the maintenance of body water homeostasis. Therefore, the regulated components, which are urine excretion and water intake caused by thirst, must compensate for whatever perturbations result from the unregulated water gains and losses. The daily urine excretion range is as low as 0.5 L to as high as 25 L depending on the requirements for water balance. When the kidney’s capacity to conserve water is maximized to the limit due to dehydration, a sensation of thirst is activated, causing oral water intake to be increased.
Because the solute concentrations in water in the body must be kept nearly constant, water loss must be regulated by a mechanism that decouples water and the solutes. The kidney can excrete the appropriate amount of water without marked perturbations in solute excretion. When water intake is too large and dilutes blood plasma, urine diluted more than plasma is excreted to concentrate the plasma. When water intake is too small to concentrate plasma, urine concentrated more than plasma is excreted to dilute the plasma. In both cases, the solute excretion varies little. Renal solute-free water excretion is mainly regulated by the antidiuretic hormone vasopressin. Vasopressin is secreted from the posterior pituitary gland into systemic circulation in response to increases in the tonicity, which is an effective osmotic pressure in the plasma, to decreases in the effective circulating volume or pressure, or to several other stimuli. In response to changing levels of vasopressin in the plasma, the kidney is capable of wide variations in free water excretion. The molecular entity of a major effector of vasopressin in the kidney is aquaporin-2 (AQP2), which is a member of the aquaporin (AQP) water channel family. Molecular identification of the AQP family has revolutionized the understanding of water transport in the body, including urine concentration and dilution.1,2 AQP2 is abundant in the collecting duct, which is the chief site of regulation of water reabsorption.3 Acute stimulation of vasopressin promotes AQP2 translocation from an intracellular reservoir to the luminal cell surface, and its chronic stimulation increases the cellular abundance of AQP2, both of which elevate the water permeability of the collecting duct cells, resulting in the promotion of water reabsorption from the urinary tubule. Its impairments result in various water balance disorders including nephrogenic diabetes insipidus (NDI). In addition to AQP2, six other AQP isoforms are expressed in the kidney and play key roles in water transport activities specific for their localization in the nephron segments without
affecting solute transport. In addition to AQPs, the localizations of urea transporters and ion transporters are highly specific through the renal tubule segments. Together with a three-dimensional configuration of the nephron, the specific transport activities of water, urea, and ion all through the renal tubule segments enable the mechanism of urine concentration and dilution.
affecting solute transport. In addition to AQPs, the localizations of urea transporters and ion transporters are highly specific through the renal tubule segments. Together with a three-dimensional configuration of the nephron, the specific transport activities of water, urea, and ion all through the renal tubule segments enable the mechanism of urine concentration and dilution.
ANATOMIC CONSIDERATIONS FOR URINE CONCENTRATION AND DILUTION MECHANISMS
General Features of the Nephron Structure
The kidney consists of two populations of nephrons: short-looped nephrons and long-looped nephrons (Fig. 4.1). Both types of loops have a hairpin or a U-shape configuration, and are defined by the length of their Henle loop. Short-looped nephrons originate from superficial glomeruli and have a Henle loop, which turns near the inner-outer medullary border and consists of a proximal straight tubule, a thin descending limb, and a thick ascending limb. Long-looped nephrons originate from the juxtamedullary glomeruli, extend into the inner medulla, bend at various levels of the inner medulla, and contain a thin ascending limb in addition to segments found in short-looped nephrons. Thin ascending limbs are found only in the inner medulla and their transition to thick ascending limbs defines the inner-outer medullary border. Thus, only thick ascending limbs are found in the outer medulla.
The Descending Part of the Henle Loop
The descending part of the Henle loop consists of the S2 proximal straight tubule in the medullary ray, the S3 proximal
straight tubule in the outer stripe of the outer medulla, and the thin descending limbs in the inner stripe of the outer and inner medulla. AQP1 is abundant in both the apical membrane and the basolateral plasma membrane in the S2 and S3 proximal tubules and the descending thin limbs.4,5,6 AQP1 is constitutively active and is not regulated by vasopressin. AQP1 is present in sufficient abundance to account for the observed water permeability of these tubule segments.7 The osmotic water permeability of the thin descending limb is extremely high due to the presence of the AQP1 water channel.6 However, there is an axial hete rogeneity in the expression levels of AQP1 in the thin descending limb; AQP1 is present in entirety through the thin descending limb of the short-looped nephron, whereas it is located in the upper half of the thin descending limb of long-looped nephron.8,9,10 A comparison of water permeability and the abundance of AQP1 in these tubule segments indicates that AQP1 is the main route for water movement across the tubules.8 The high water permeability of these segments is critical for the countercurrent multiplication system that is described in the section Countercurrent Multiplication in the Outer Medulla and Other Mechanisms in the Inner Medulla, which follows. As expected, a severely impaired urine concentrating ability is observed in AQP1 knockout mice.11
straight tubule in the outer stripe of the outer medulla, and the thin descending limbs in the inner stripe of the outer and inner medulla. AQP1 is abundant in both the apical membrane and the basolateral plasma membrane in the S2 and S3 proximal tubules and the descending thin limbs.4,5,6 AQP1 is constitutively active and is not regulated by vasopressin. AQP1 is present in sufficient abundance to account for the observed water permeability of these tubule segments.7 The osmotic water permeability of the thin descending limb is extremely high due to the presence of the AQP1 water channel.6 However, there is an axial hete rogeneity in the expression levels of AQP1 in the thin descending limb; AQP1 is present in entirety through the thin descending limb of the short-looped nephron, whereas it is located in the upper half of the thin descending limb of long-looped nephron.8,9,10 A comparison of water permeability and the abundance of AQP1 in these tubule segments indicates that AQP1 is the main route for water movement across the tubules.8 The high water permeability of these segments is critical for the countercurrent multiplication system that is described in the section Countercurrent Multiplication in the Outer Medulla and Other Mechanisms in the Inner Medulla, which follows. As expected, a severely impaired urine concentrating ability is observed in AQP1 knockout mice.11
In addition to AQP1, AQP7 is expressed in the apical membrane of the S3 proximal tubule. AQP7 transports glycerol as well as water, thus AQP7 is an aquaglyceroporin .12 Studies in AQP7 knockout mice suggest that water absorption mediated by AQP7 is small compared to that of by AQP1 and minimally contributes to urine concentration; whereas glycerol absorption by AQP7 may be important in glycerol metabolism in the body.13
A urea transporter UT-A2 is present in the thin limb of the long-looped nephron in the inner medulla and the thin descending limb of the short-looped nephron in the outer medulla. Thus, these segments have a high urea permeability14 and play an important role for urea-recycling pathways that serve to maintain high urea concentrations within the inner medullary interstitium, which is critical for the urinary concentration that is described in the section Urea Accumulation in the Inner Medulla, which follows.
The Ascending Part of the Henle Loop
The ascending part of the Henle loop consists of the thin ascending limb in the inner medulla, the medullary thick ascending limb in the inner stripe of the outer medulla, and the cortical thick ascending limb in the medullary rays. The thin ascending limb is located exclusively in the inner medulla, is present only in the long-looped nephron, and becomes a thick ascending limb at the inner-outer medullary border. The thin ascending limb has extremely low water permeability and AQPs are not detected. By contrast, the ClC-K1 chloride channel is expressed in the apical and basolateral membrane of the entire length of the thin ascending limb.9,15,16 Studies with immunohistochemical labeling and computer-assisted reconstruction in rats’ inner medullas show that the thin ascending limb marked by ClC-K1 expression occurs about 160 µm before the loop bend.9
The thick ascending limb has essentially no water permeability and does not express AQPs. On the other hand, Na-K-2Cl cotransporter (NKCC2) is present in the apical membrane,17 and Na-K-ATPase, a Na pump, is present in the basolateral membrane in the thick ascending limb. NaCl is actively absorbed by these transporters, resulting in the dilution of the luminal fluid in this segment, which works as the single effect in the countercurrent multiplication (described in the following sections). Urea permeability in the cortical thick ascending limb is higher than in the medullary thick ascending limb.18 This may also contribute to the dilution of the luminal fluid as it flows up to the cortex by passive urea absorption.
The Distal Convoluted Tubule and Connecting Tubule
After exiting the Henle loop, the luminal fluid enters the distal convoluted tubule that expresses the thiazide-sensitive Na-Cl cotransporter NCC.17,19 NCC is important for NaCl reabsorption that reduces fluid delivery to the collecting duct, leading to increases in urinary concentrating ability Water permeability in the distal convoluted tubule is low and the expression of AQP2 or vasopressin V2 receptor is not observed.
Several distal tubules merge to form a connecting tubule arcade. The connecting tubule cells express both AQP2 and vasopressin V2 receptor, suggesting that, like the collecting ducts, the connecting tubules are sites of regulated water absorption. Moreover, vasopressin-regulated water absorption in the connecting tubule can result in a considerable amount of free-water absorption during antidiuresis because the large aggregate epithelial surface area of the connecting arcades is estimated to be roughly equivalent to that of the cortical collecting ducts.20
An AQP2 knockin mice model of nephrogenic diabetes insipidus, a rare disease manifested by an inability to respond to vasopressin, shows a severe urinary concentrating defect that results in neonatal death.21 Meanwhile, AQP2 conditional selective knockout in the collecting duct but not in the connecting tubule rescues mice from the lethal phenotype that is observed in mice lacking AQP2 globally.22 Although all types of mice show a severe urinary concentrating defect, these findings confirm an important role of the connecting duct for urinary concentration.
The Collecting Duct
The collecting duct is the final structure in the nephron. The luminal fluid from the connecting tubules then enters the collecting tubules in the superficial cortex. The collecting duct spans all the regions of the kidney. From the superficial cortex, the collecting duct descends through the cortex and the outer medulla. In the inner medulla, these collecting ducts
continually merge, finally forming the ducts of Bellini that open into the renal pelvis at the papillary tip. The renal pelvis is continuous with the ureter. The collecting ducts are arrayed parallel to the Henle loop. The collecting ducts have several morphologically discrete tubule segments: the cortical collecting duct, the outer stripe portion of the outer medullary collecting duct, the inner stripe portion of the outer medullary collecting duct, the initial part of the inner medullary collecting duct (IMCD), and the terminal part of the IMCD.
continually merge, finally forming the ducts of Bellini that open into the renal pelvis at the papillary tip. The renal pelvis is continuous with the ureter. The collecting ducts are arrayed parallel to the Henle loop. The collecting ducts have several morphologically discrete tubule segments: the cortical collecting duct, the outer stripe portion of the outer medullary collecting duct, the inner stripe portion of the outer medullary collecting duct, the initial part of the inner medullary collecting duct (IMCD), and the terminal part of the IMCD.
The collecting duct, under the control of vasopressin and other factors, is the nephron segment responsible for the final control of water excretion. The collecting duct expresses the vasopressin V2 receptor and AQP2, which are the primary targets for vasopressin regulation. In the absence of vasopressin, AQP2 resides in the vesicles below the apical cell surface and the entire collecting duct is very water impermeable. When the body is dehydrated, plasma osmolality is increased, which is sensed by the hypothalamic osmoreceptors and results in vasopressin secretion by the posterior pituitary. Vasopressin binds to V2 receptors in the basola teral membrane of the principal cells of the collecting duct, stimulates adenylate cyclase to produce cAMP, activates protein kinase A, phosphorylates AQP2, and inserts this water channel into the apical cell surface, which results in a significant increase in the collecting duct water permeability and water reabsorption.23,24,25 This process is described in detail in the section Aquaporin-2, which follows. Not only are AQP2 water channels present, but also are AQP3 and AQP4 water channels in the collecting duct principal cells. There is axial heterogeneity in the abundance of AQP3 and AQP4; AQP3 is abundant in the connecting tubule, the cortical collecting duct, and the outer medullary collecting duct; whereas AQP4 is most abundant in the inner medullary collecting duct.26 AQP3 and AQP4 are located in the basolateral membrane of these cells and represent a potential exit pathway from the cell to the interstitium for water entering via AQP2.
Water reabsorption mainly occurs in the cortex and the outer medulla. The inner medulla has the highest osmolality and is important for the reabsorption of the remaining water when maximal water reabsorption is required. Rather, water reabsorption by the terminal part of IMCD is greater during diuresis than during antidiuresis27 because of its large transepithelial osmolality difference and relatively high basal water permeability compared to other portions of the collecting duct.
The connecting tubule and the cortical collecting duct express the amiloride-sensitive sodium channel ENaC.28,29 Sodium is actively absorbed via ENaC in the apical membrane of the principal cells and exits the cells via Na-K-ATPase in the basolateral membrane. Sodium reabsorption via ENaC is increased by vasopressin or aldosterone and plays an important role for reducing fluid delivery to the medullary collecting duct, thus leading to increases in urinary concentrating ability.30 On the other hand, the sodium permeability is low in the medullary collecting duct.
The urea permeability is extremely high only in the terminal IMCD and low in other portions of the collecting duct.31 UT-A1 and UT-A3 urea transporters are present in the terminal IMCD cells.32 Urea reabsorption in this segment is important for the preservation of high urea concentrations in the inner medullary interstitium, which are essential for its urine concentrating ability and are also important for minimizing the urinary loss of urea that is a major part of the urea recycling pathway. This process is described in detail in the section Urea Accumulation in the Inner Medulla, which follows.
The Vasculature
The blood vessels named the vasa recta carry blood into and out of the renal medulla. Like the Henle loop, there are the descending and ascending parts in the vasa recta, both of which are arranged in parallel and in mutual proximity. Blood from efferent arterioles of juxtamedullary nephrons enters into the descending vasa recta in the medulla, passes through the capillary plexus located at various depths within the medulla, then merges to form the ascending vasa recta. Different from the Henle loop, the descending and ascending vasa recta are separated by the capillary plexus (see Fig. 4.1). The AQP1 water channel and the UT-B urea transporter are present in the descending vasa recta and enhance the countercurrent exchange of water and urea between the descending and ascending vasa recta in the medulla.6,33 This countercurrent exchange reduces the effective blood flow, contributing to the conservation of osmolality gradients in the medullary interstitium.
The Medullary Interstitium
The interstitium of the outer medulla and the outer portion of the inner medulla is a narrow space that is important for limiting solute diffusion upward along the medullary axis.34 To the contrary, the interstitium of the inner half of the inner medulla is much larger. In this region, the interstitial cells are interspersed in a gelatinous matrix of highly polymerized hyaluronic acid,35 which is largely devoid of capillary plexuses or lymphatics. This structure slows the diffusion of solute and water in the inner medulla.
The Pelvis
The collecting duct finally opens at the papillary surface and urine enters the pelvic space. In the kidney with only one papilla, such as in hamsters and rats, the renal pelvis is an intrarenal urinary space surrounding the papilla. In the kidney with many papillae, such as in humans, each papilla is surrounded by a funnel-shaped calyx. In the human kidney, it is the compartment between the calyces and the ureter that is called the pelvis.36 This compartment is not present in kidneys with one papilla, where the pelvis is a direct extension of the ureter. The renal pelvic (calyceal) wall contains smooth muscle layers. Contractions of these muscles induce regular peristaltic contractions of the wall that strongly
compress the medullary tissue, resulting in intermittent flow in the collecting ducts and the Henle loop.37
compress the medullary tissue, resulting in intermittent flow in the collecting ducts and the Henle loop.37
URINE CONCENTRATION MECHANISMS
General Features of Urine Concentration
In an adult human, glomerular filtrate is about 180 L per day, most of which is reabsorbed by the high water permeable proximal tubule and descending limb of the Henle loop. However, regulation of water excretion mainly occurs after the luminal fluid reaches the distal tubule. The osmolalities of luminal fluid along the rat nephron are shown in Figure 4.2. The luminal fluid in the proximal tubule is isosmotic to plasma, regardless of antidiuresis or diuresis. This fluid then enters the thin descending limb of Henle and is concentrated as it flows down to the bend of the Henle loop because of water reabsorption. As the fluid flows up the ascending limb of the Henle loop, the luminal fluid becomes diluted because this segment is impermeable to water, and the thick ascending limb actively absorbs NaCl. This diluted fluid finally enters the collecting duct system. In diuresis (low AVP), the fluid remains hypotonic. On the other hand, in antidiuresis (high AVP), the fluid is concentrated to a level far greater than plasma by water reabsorption as it flows down the collecting duct. This is due to a vasopressin-induced significant increase in water permeability of this segment and the existence of an axial osmola lity gradient in the medulla. This osmolality gradient with the highest degree of hyperosmolality at the papillary tip is maintained by several mechanisms, including countercurrent multiplication, countercurrent exchange, and urea accumulation in the inner medulla, as described subsequently.
Countercurrent Multiplication in the Outer Medulla and Other Mechanisms in the Inner Medulla
Countercurrent multiplication is an essential process for generating a medullary osmotic gradient along the corticomedullary axis and occurs in the Henle loop.38 The counterflow arrangement of this loop makes the osmolality difference between the ascending and descending limbs to be multiplied, resulting in an enormous increase in osmolality toward the bend of this loop. Figure 4.3 illustrates the basic components of this mechanism in the short Henle loop. In the thick ascending limb of Henle, which corresponds to the right limb in Figure 4.3, NaCl is actively reabsorbed at any level of this ascending limb. Because this segment is impermeable to water, the luminal fluid is diluted and NaCl concentrations of the surrounding interstitium become higher than the luminal fluid. On the other hand, the descending limb of the Henle loop, which corresponds to the left channel in Figure 4.3, is highly permeable to water due to the presence of an AQP1 water channel, but is impermeable to NaCl. Water is passively reabsorbed from the descending limb lumen to the interstitium, which has a high osmolality due to NaCl accumulation. The luminal fluid in the descending limb is concentrated by water reabsorption and is continually concentrated downward to the bend of the loop, and then enters to the ascending limb. High NaCl concentrations of this fluid further promote NaCl reabsorption in the ascending limb. This fluid is then diluted by this NaCl absorption while flowing toward the top of the loop. Because the transverse concentration difference between the two limbs is always maintained by active NaCl absorption by the ascending limb (this is called the single effect ) and water permeability of the descending limb at each level of the longitudinal axis is high, the bend of the loop achieves a progressively higher osmolality. This results in an enormous increase in osmolality toward the bend of the Henle loop.
A countercurrent mechanism is accepted for generating the axial osmolality gradient in the outer medulla, where active NaCl reabsorption occurs in the thick ascending limb. However, in the inner medulla, there is little activity of NaCl transport despite the fact that osmolality continues to increase toward the tip of the papilla. The thin ascending limb of the Henle loop in the inner medulla cannot actively reabsorb NaCl. The high inner medullary interstitial osmola lity provides a critical driving force for osmotic water flow across the collecting ducts, where the water permeability is regulated by vasopressin and AQP2 water channel, as described later. For explaining this concentrating effect in the inner medulla, a passive mechanism is widely accepted.39,40 In this model, urea efflux from the terminal IMCD, which is mentioned previously, results in high urea concentrations in the inner medullary interstitium. This causes osmotic withdrawal of water from the thin descending limb and increases NaCl concentrations of the luminal fluid. This highly concentrated NaCl then exits passively from the thin ascending limb (works as single effect), and the luminal fluid is progressively diluted as it flows up. This passive transport process may produce an axial NaCl concentration gradient in the inner medulla. This model requires that the thin descending limb is highly permeable to water and but not to NaCl or urea, whereas the thin ascending limb is permeable to NaCl but not to water or urea. A microperfusion study of the thin ascending limb shows that the permeability of chloride and sodium are higher than urea, and that luminal dilution takes place when tubule segments are perfused in a condition simulating an in vivo situation.41 Consistent with these physiologic studies, the ClC-K1 chloride channel is exclusively expressed in the thin ascending limb. ClC-K1 knockout mice show a large urinary concentrating defect with impaired urea as well as NaCl accumulation in the inner medulla.42,43 This finding confirms the importance of a rapid chloride exit in the thin ascending limb and supports the passive mechanism.
Simulations based on many mathematical models incorporating physiologic parameters have been examined to show the validity of the passive model, but they usually failed to show satisfactory results. However, this does not necessary neglect the passive model because solute permeability and water permeability are sometimes different in species, in nephrons (long looped versus short looped), and within the segment (axial heterogeneity). Moreover, based on extensive studies of the rat inner medulla by immunohistochemical labeling and computer-assisted reconstruction, Pannabecker et al.10 show that three-dimensional tubular and vascular relationships are more complex than thought before and a more comprehensive understanding of three-dimensional functional architecture is critically important for modeling urine concentration mechanisms of the inner medulla.
Countercurrent Exchange
Although the hypertonic medulla is essential for the abi lity to concentrate urine, blood flow may decrease the high solute concentrations in the medulla. The osmolality of blood entering to the medulla from the general circulation is far lower than the medullary interstitium and may decrease the osmolality of the interstitium as they come to equilibrium. Meanwhile, blood going out the medulla to the general circulation can carry out the solutes from the interstitium. To minimize this dissipation of the high solute concentrations in the medulla, there is a process called countercurrent exchange . Blood supply to the medulla is done by the vasa recta with the descending and ascending limbs arranged in a counterflow configuration. The vasa recta are permeable to water, sodium, and urea. Therefore, in the descending vasa recta, blood loses water and gains solutes as it flows down because
of the increasing osmolality in the medullary interstitium. After entering the ascending vasa recta, blood gains water and loses solutes as it flows up because of the decreasing osmolality of the surrounding interstitium. This exchange of water and solute between the descending and ascending vasa recta is called countercurrent exchange. This mechanism minimizes the solute washout from the inner medulla to the systemic circulation. Thus, this exchange at each level in the medulla preserves the axial solute concentration gradients in the medullary interstitium. If the blood flow is decreased, for instance in the conditions of volume depletion in the body, the efficiency of countercurrent exchange is further increased by getting enough time for achieving osmotic equilibration, leading to an increase in urinary concentrating ability.
of the increasing osmolality in the medullary interstitium. After entering the ascending vasa recta, blood gains water and loses solutes as it flows up because of the decreasing osmolality of the surrounding interstitium. This exchange of water and solute between the descending and ascending vasa recta is called countercurrent exchange. This mechanism minimizes the solute washout from the inner medulla to the systemic circulation. Thus, this exchange at each level in the medulla preserves the axial solute concentration gradients in the medullary interstitium. If the blood flow is decreased, for instance in the conditions of volume depletion in the body, the efficiency of countercurrent exchange is further increased by getting enough time for achieving osmotic equilibration, leading to an increase in urinary concentrating ability.
Urea Accumulation in the Inner Medulla
The major solute responsible for the inner medullary osmolality gradient is urea and NaCl; although, in the outer medulla, it is NaCl.45 High osmolality in the inner medulla is maintained by urea accumulation in this region in addition to NaCl. There are several mechanisms to maintain the high urea accumulation in the medulla that are called urea recycling (Fig. 4.4).46,47
Among the collecting duct, only the terminal IMCD has a high urea permeability due to the presence of UT-A1 and UT-A3 urea transporters in this segment.31,32 During antidiuresis, water is absorbed in the collecting duct segments in the cortex and the outer medulla, which are impermeable to urea. Thus, the urea concentrations of the luminal fluid are progressively increased as it flows down through the connecting tubule, the cortical collecting duct, and the outer medullary collecting duct. When the luminal fluid reaches the terminal IMCD, which is highly permeable to urea, urea is rapidly absorbed from the lumen to the surrounding interstitium. During antidiuresis, the urea permeability of the inner collecting duct is increased by vasopressin, and this accounts for further rapid reabsorption of urea.48,49
Once reabsorbed, urea is not rapidly washed out to the general circulation because of countercurrent exchange and the low effective blood flow of the vasa recta, resulting in the high urea accumulation in the inner medullary interstitium. In addition, during antidiuresis, urea reabsorption by the terminal IMCD has another advantage for decreasing the luminal osmolality and preventing the osmotic diuresis when luminal fluid is very concentrated by enhanced water reabsorption in the upper portions of the collecting duct.
After being reabsorbed by the terminal IMCD, some urea in the inner medullary interstitium enters into the thin limbs of the long Henle loop where urea permeability is high due to the presence of UT-A2.50 Urea entered in the thin limbs is then carried upward to the cortex through the thick ascending limb, the distal convoluted tubule, the connecting tubule, and the cortical collecting duct, and again enters into the medulla through the outer and inner medullary collecting ducts. Because these segments before the terminal IMCD have low urea permeability, urea can be returned to this region with little loss. Urea is again reabsorbed by the terminal IMCD and recycled to the inner medullary interstitium (Fig. 4.4).
In addition to the recycling pathway via the long loop in the inner medulla, there is another recycling pathway using the short loop in the outer medulla. Some parts of the urea reabsorbed by the terminal IMCD exit the inner medulla via the vasa recta. The vasa recta and the short Henle loop are arranged in parallel and in mutual proximity, and the descending limb of the short loop expresses the UT-A2 urea transporter and has a high urea permeability. Therefore, urea carried by the vasa recta is able to enter the short loop, then is convected through the distal convoluted tubule, the connecting tubule, and the collecting duct. When the luminal fluid finally reaches the terminal IMCD, urea is again reabsorbed to the surrounding inner medullary interstitium (Fig. 4.4).
The reabsorption sites among the collecting duct system are different between water and urea. Water is reabsorbed mainly in the cortex and the outer medulla, where blood flow is so high that water can be rapidly supplied to the systemic circulation. Furthermore, this reabsorption does not dilute the inner medulla. On the other hand, urea is reabsorbed in the inner medulla. Owing to low blood flow in this region and urea recycling pathways, urea can be trapped in the inner medulla, which is required for the passive mechanism, as described previously.
Regulated Water Reabsorption in the Collecting Duct
A countercurrent multiplication in the Henle loop generates a hypertonic medulla. A countercurrent exchange in the vasa recta minimizes the dissipation of this osmotic gradient. However, either of these processes has the ability to regulate water reabsorption in response to the body water balance. This function is performed by the collecting duct. The collecting duct is responsible for the final control of urine concentration, and its water permeability is regulated by vasopressin and other factors. In the absence of vasopressin, the collecting duct has an extremely low water permeability. Because the luminal fluid exiting the Henle loop is diluted, the fluid remains diluted after passing through the collecting duct, yielding a large volume of hypotonic urine. In the presence of vasopressin, the water permeability of the collecting duct is dramatically increased. Based on the hypertonic medullary interstitium generated by countercurrent multiplication and other processes, as described previously, an increase in water permeability of the collecting duct results in significant water reabsorption by the osmotic gradient between the lumen and the surrounding interstitium (Fig. 4.2). The molecular entity of this regulation of water permeability of the collecting duct is vasopressin V2 receptors and the AQP2 water channel expressed in the principal cells of the collecting duct.26,51,52,53,54,55 V2 receptors and AQP2 are present in the connecting tubule and all segments of the collecting duct. When vasopressin binds to V2 receptors in the basolateral membrane of these cells, it stimulates adenylate cyclase to produce cAMP, activates protein kinase A, phosphorylates AQP2, and inserts this water channel into the apical cell surface, which results in a significant increase in the collecting duct water permeability and water reabsorption. In addition, vasopressin stimulation increases AQP2 protein abundance in the cell (Fig. 4.5). These processes are described in detail in the section Aquaporin-2, which follows. On the basola teral cell surface, AQP3 and AQP4 are located and represent a potential exit pathway from the cell to the interstitium for water entering via AQP2. Water reabsorption mainly occurs in the connecting tubule, the cortical collecting duct, and the outer medullary collecting duct. In the cortex and the outer medulla, blood flow is sufficiently high so that absorbed water can be carried out to the general circulation without diluting the interstitium. On the other hand, the inner medulla has the highest osmolality and is important for reabsorption of the remaining water when maximal water reabsorption is required.
URINE DILUTION MECHANISMS
Approximately 50 mOsm per kilogram of water is the limit of the urinary diluting ability of humans. The mechanisms responsible for urinary dilution nearly overlap with that for urinary concentration. These two conditions between diuresis and antidiuresis are switched mainly by the collecting duct water permeability, which is regulated by vasopressin stimulation and the AQP2 water channel.
In the thick ascending limb, the luminal fluid is diluted by active NaCl absorption through NKCC256 and Na-H exchanger NHE3,57 regardless of antidiuresis or diuresis. In addition, water impermeability of this segment preserves the luminal low osmolality by preventing water fluxes. The distal convoluted tubule also has the ability to actively absorb NaCl due to the presence of Na-Cl cotransporter NCC19 and is impermeable to water, resulting in the dilution of the luminal fluid to an osmolality of about 100 mOsm per kilogram of water. In diuresis, this diluted fluid passes through the collecting duct because its water permeability is very low. Active Na reabsorption through Na channel ENaC in the collecting duct29 can further dilute the luminal fluid.58
In addition to diuresis, there is another mechanism that further promotes urinary dilution. The terminal IMCD has a higher basal water permeability than other portions of the collecting duct and is surrounded by a high inner medullary interstitium. Because the osmolality of the luminal fluid reaching the terminal IMCD in diuresis is lower than that in antidiuresis, the transepithelial osmolality gradient in diuresis is larger than that in antidiuresis, resulting in a higher water reabsorption in this segment.27 This reduces the inner medullary interstitial osmolality, leading to a further decrease in urinary concentrating ability.
VASOPRESSIN
The primary determinant of solute-free water excretion is the regulation of urinary water excretion by circulating levels of vasopressin in plasma. This section describes the regulation of vasopressin secretion from the neurohypophysis.
Structure and Synthesis
Arginine vasopressin is the antidiuretic hormone of most mammals, although members of the pig family have lysine vasopressin, in which a lysine replaces the arginine in position 8 of arginine vasopressin. Vasopressin is produced by the hypothalamic neurohypophyseal tract. This tract is composed of magnocellular neurons that arise bilaterally in the supraoptic (SON) and paraventricular (PVN) nuclei of the hypothalamus and project medially to merge in the pituitary stalk and form the posterior pituitary gland in the sella tunica. Vasopressin is synthesized as part of a protein precursor of approximately 21,000 Da of molecular mass that incorporates a signal peptide at its amino terminus and vasopressin, neurophysin, and copeptin at its carboxyl terminus. In the endoplasmic reticulum, the signal peptide is removed. The prohormone then moves through the Golgi apparatus and into the neurosecretory granules that travel down the axon. In the neurosecretory granules, the prohormone is processed to yield amidated vasopressin, neurophysin, and copeptin.59 Vasopressin and neurophysin form an insoluble complex in the nerve terminal and dissociate from each other after its release into the general circulation.
Regulation of Vasopressin Secretion by the Tonicity of Body Fluid
Under physiologic conditions, the most important determinant of vasopressin secretion is the tonicity of body fluid. Tonicity defines the forces that determine the net flux of water between two solutions separated by a membrane permeable to water but impermeable to certain solutes. On the other hand, osmolality refers to the forces generated by solutes that reduce the random movement of water molecules. The osmolality is a concentration of all of the solute in water, whereas the tonicity is an effective osmotic pressure and a concentration of all of the osmotically effective solutes. There are two types of solutes: osmotically effective and noneffective. Osmotically effective solutes, such as Na and Cl, are characterized by their inability to move across the cell membrane, resulting in a difference in their concentrations between the intracellular and extracellular compartments. On the other hand, osmotically noneffective solutes, such as urea and ethanol, are characterized by their ability to diffuse freely across the cell membrane and their concentrations between these two compartments are similar. Glucose is confined in the extracellular compartment and is osmotically effective in the absence of insulin; whereas in the presence of insulin, glucose is able to enter the cell by insulin-activated transporters, thereby becoming osmotically noneffective. Vasopressin secretion is finely regulated by the tonicity of body fluid.
The changes in the tonicity of body fluid are sensed by a group of cells called osmoreceptor neurons. These neurons are located in several brain areas including the organum vasculosum laminae terminalis (OVLT), the SON, and the PVN nuclei of the hypothalamus.60 It is postulated that an increase in the tonicity of the extracellular compartment causes water withdrawal and shrinkage of the osmoreceptor neurons. Owing to the presence of mechanosensitive cation channels in these cells, cell shrinkage induces a positive charge influx and depolarizes the cell membrane, resulting in the triggering of neuronal action potentials. Such neurons send axonal projections to the SON, where they release the excitatory transmitter glutamate to synaptically excite the magnocellular neurons that release vasopressin in their distal axons.60,61
Recent studies show that the transient receptor potential vanilloid (TRPV) channels are likely to be the major component of the osmoreceptor. The N-terminal truncated TRPV1 channel is selectively expressed in osmosensory neurons in OVLT. The responses of these neurons to hypertonic stimuli, including reactive increases in cation channel conductance, membrane depolarization, and increased frequency of neuronal action potentials, were greatly inhibited in TRPV1 knockout mice. Furthermore, TRPV1 knockout mice showed a higher serum osmolality than wild-type mice, indicating a decreased sensitivity of osmosensation.62 However, these results were not reproduced by another study.63 In addition to TRPV1, TRPV2 and TRPV4 are present in osmoreceptor neurons and functional characteristics of these channels show responsiveness to the tonicity. Further studies, such as examining a possible hetero-oligomerization of these TRPV channels, are necessary for a molecular understanding of osomosensation.60,61
The functional properties of osmoregulatory mechanisms have a discrete threshold for vasopressin secretion, above which a linear relationship between plasma osmolality and vasopressin levels occurs.64 When the plasma osmolality is below a threshold level, vasopressin secretion is suppressed to low or undetectable levels (0.5 pg per milliliter). Above this threshold, vasopressin secretion increases linearly in direct proportion to plasma osmolality. Both the threshold level and the slope of the regression line relating vasopressin secretion to plasma osmolality vary between persons due to unknown genetic factors and between the different conditions in the same individual. In general, the threshold is approximately 280 mOsm per kilogram of water, and above this threshold, a rise in plasma osmolality of 1% increases plasma vasopressin by approximately 0.4 to 1.0 pg per milliliter. The renal response to circulating vasopressin is also linear, with urinary concentration that is proportional to vasopressin levels from 0.5 to 5 pg per milliliter, above which urinary osmolality is maximal and cannot increase further. Therefore, changes of as little as 1% in plasma osmolality are sufficient to cause a significant increase in urinary concentration, and maximal antidiuresis is achieved by an increase in plasma osmolality of only about 10 to 15 mOsm per kilogram of water above the threshold. Furthermore, vasopressin secretion occurs within minutes in response to changes in plasma osmolality. After release into the systemic circulation, vasopressin distributes quickly because of its small size, and the equilibration between the vascular and extravascular compartments is almost complete within 10 minutes. The half-life of vasopressin in plasma is within
30 minutes. Therefore, the changes in plasma osmolality are rapidly transferred to changes in urinary concentration.
30 minutes. Therefore, the changes in plasma osmolality are rapidly transferred to changes in urinary concentration.
There are several factors that can alter the threshold and the sensitivity, which is the slope of the regression line relating vasopressin secretion to plasma osmolality. The most important factor is hemodynamic changes, including blood pressure and effective arterial blood volume, which are described in the next section. Aging enhances the sensitivity of vasopressin secretion.65 In pregnancy, relaxin, an ovarian hormone produced by the corpora, reduces the threshold and increases vasopressin secretion, contributing partly to the increase in blood volume.66
Hemodynamic Regulation of Vasopressin Secretion
Vasopressin secretion is also affected by changes in blood volume and pressure.67 A decrease in blood volume, such as with bleeding, sequestration or redistribution of blood, or fluid loss by sweating, diarrhea, or vomiting, can increase vasopressin secretion. The vasopressin release mechanism is much less sensitive to small changes in blood volume than to comparable changes in osmolality. Small reductions under 8% in blood volume usually have little effect on plasma vasopressin concentration. On the other hand, further acute reduction in blood volume significantly stimulates vasopressin secretion. Usually, 20% to 30% reductions in blood volume increase vasopressin secretion to the levels of 20 to 30 times normal. The stimulus-response relationship follows an exponential pattern. The vasopressin response to an acute reduction in blood pressure is similar to the response to blood volume. Reductions in blood pressure under 10% have little effect on vasopressin secretion, whereas blood pressure decreases of 20% to 30% result in significant increase in plasma vasopressin. The effects of reductions in blood volume and pressure are exerted through shifting the threshold and sensitivity of vasopressin secretion to osmotic stimuli.68
These hemodynamic effects on vasopressin secretion are mediated at least in part by neural pathways that originate in stretch-sensitive receptors called baroreceptors in the cardiac atria, the aorta, and the carotid sinus. From these receptors, afferent nerve fibers ascend in the vagus and glossopharyngeal nerves to the nuclei of the tractus solitarus (NTS) in the brainstem.69 A variety of postsynaptic pathways from the NTS then project, both directly and indirectly, to the SON and the PVN nuclei of the hypothalamus.
Other Influences for Vasopressin Secretion
The sensation of nausea, with or without vomiting, is a powerful stimulus to vasopressin secretion.72 Nausea increases plasma vasopressin in excess of 200 to 400 pg per milliliter. The pathways responsible for this effect are located in the chemoreceptor zone in the postrema area of the brainstem. Water loading blunts, but does not abolish, the effect of nausea on vasopressin secretion, suggesting that the mechanism of emetic effect has a possible common pathway with that of an osmotic effect.
The renin-angiotensin system also influences the osmotic regulation of vasopressin secretion.73 Neurons in the subfornical organ (SFO) contain angiotensin II and project axons onto hypothalamic magnocellular neurosecretory cells (MNCs) located in the SON and PVN nuclei. It is suggested that the release of angiotensin II may contribute to the potentiation of osmotically evoked action potential firing and vasopressin release.
Acute hypoglycemia induces a modest increase in plasma vasopressin,65 although the pathways responsible for this effect are unknown.
The effects of pregnancy on the osmotic regulation of vasopressin secretion are complex. The systemic hemodynamic profile of pregnancy is characterized by a decrease in mean arterial pressure, a rise in cardiac output and plasma volume, and a decrease in body tonicity. This is due to a decrease in the osmotic threshold for vasopressin secretion because of arterial underfilling secondary to systemic arterial vasodilatation.74,75 In addition, relaxin, an ovarian hormone produced by the corpora, reduces the threshold and increases vasopressin secretion, which contributes partly to the increase in blood volume.66 On the other hand, the metabolic clearance of vasopressin is increased, owing to circulating cystine aminopeptidase (vasopressinase) produced by the placenta. This mechanism rarely causes transient diabetes insipidus in late pregnancy76 because of the increased release of vasopressin.
MOLECULAR MECHANISMS OF VASOPRESSIN-REGULATED WATER PERMEABILITY OF THE COLLECTING DUCT
Vasopressin-regulated water permeability of the collecting duct is responsible for the final control of urine concentration. Upon vasopressin stimulation, the water permeability of the collecting duct is dramatically increased. Based on the hypertonic medullary interstitium, the increased water permeability results in significant water reabsorption and urine concentration. The vasopressin V2 receptor and AQP2 in the collecting duct are the primary targets for vasopressin. Vasopressin-regulated AQP2 translocation and abundance change the water permeability of the collecting duct principal cells. The essential roles of the vasopressin V2 receptor and AQP2 in urine concentrations are demonstrated by mouse models. Male mice that were introduced with a nonsense mutation into the AVPR2 gene, which codes the vasopressin V2 receptor and is located in the × chromosome, died within 7 days after birth due to hypernatremic
dehydration.77 Mice models with AQP2 protein deletion in the collecting duct also showed a severe defect in urinary concentration.22,78 This section describes the molecular mechanisms for the regulation of water permeability of the collecting duct principal cells. Figure 4.5 illustrates several major mechanisms.
dehydration.77 Mice models with AQP2 protein deletion in the collecting duct also showed a severe defect in urinary concentration.22,78 This section describes the molecular mechanisms for the regulation of water permeability of the collecting duct principal cells. Figure 4.5 illustrates several major mechanisms.
Vasopressin V2 Receptor
When circulating vasopressin reaches the kidney, vasopressin binds to the vasopressin V2 receptor expressed on the basolateral plasma membrane of the collecting duct principal cells and initiates the signal transduction in the cell. The V2 receptor is a 371 amino acid protein with 7 membrane- spanning domains and couples to heterotrimeric G-proteins.79,80 The binding of the V2 receptor to vasopressin promotes the disassembly of the bound heterotrimeric G-protein (Gs) into Gα and Gβγ subunits. GDP-GTP exchange occurs in the Gα subunit and this activated Gsα then stimulates adenylate cyclase, resulting in an increase in intracellular cAMP levels (Fig. 4.5). Increased cAMP activates protein kinase A, which phosphorylates AQP2, and this phosphorylation event is required to increase the water permeability and water reabsorption of renal principal cells, which is described in the next section.
Aquaporin-2
AQP2 is a 271 amino acid protein with 6 membrane-spanning domains and 2 conserved, membrane-embedded asparagine-proline-alanine (NPA) motifs, and selectively permeates water.3 AQP2 is predominantly expressed in the collecting duct principal cells (Fig. 4.6).81,82 In response to vasopressin, AQP2 recycles between the luminal cell surface membrane (also called the apical membrane) and the intracellular subapical storage vesicles of the collecting duct principal cells. In the absence of vasopressin, AQP2 predominantly resides in the subapical vesicles. Because water molecules slowly diffuse through the lipid bilayer, the water permeability of the cell membrane without AQPs is low. Therefore, basal water permeability of the principal cells is also low. In the presence of vasopressin, AQP2-containing vesicles fuse with the apical membrane via exocytosis, thus inducing an exceedingly high
water permeability of this apical membrane (Fig. 4.7).23,24,25 Vasopressin increases the water permeability by a factor of 10 to 100 in the cortical collecting duct,49 20 to 30 in the outer medullary collecting duct,83 and 10 to 30 in the initial IMCD.49 The terminal IMCD has a higher basal water permeability and vasopressin increases the water permeability by a factor of 10 in the terminal IMCD.49
water permeability of this apical membrane (Fig. 4.7).23,24,25 Vasopressin increases the water permeability by a factor of 10 to 100 in the cortical collecting duct,49 20 to 30 in the outer medullary collecting duct,83 and 10 to 30 in the initial IMCD.49 The terminal IMCD has a higher basal water permeability and vasopressin increases the water permeability by a factor of 10 in the terminal IMCD.49
The water permeability of the basolateral membrane of the principal cells is always high because of the presence of AQP3 and AQP4, and is not rate limiting for water transport. Thus, water that enters into the cell from the lumen via AQP2 can exit to the hypertonic medullary interstitium by the osmotic gradient. Upon the binding of vasopressin to its receptors, AQP2 is phosphorylated and this phosphorylation event is a requisite for AQP2 translocation to the apical membrane (Fig. 4.5).
Aquaporin-2 Phosphorylation and Trafficking
AQP2 forms homotetramers,84 and at least three of four monomers in AQP2 tetramers must be phosphorylated for successful apical membrane localization.85 Phosphorylation of serine 256 is required for the trafficking of AQP2 to the cell surface in cultured cells.86,87 Protein kinase A and its substrates are present throughout the cell; therefore, localization of protein kinase A in specific sites is necessary for PKA to effectively phosphorylate its target. The phosphorylation process is assisted by protein kinase A anchoring proteins
(AKAPs). The tethering of PKA to AKAPs is required for AQP2 shuttling to the cell surface.88 A splice variant of AKAP-18, AKAP-18δ, is specifically involved in AQP2 shuttling89 and the involvement of AKAP-220 has also been reported.90
(AKAPs). The tethering of PKA to AKAPs is required for AQP2 shuttling to the cell surface.88 A splice variant of AKAP-18, AKAP-18δ, is specifically involved in AQP2 shuttling89 and the involvement of AKAP-220 has also been reported.90
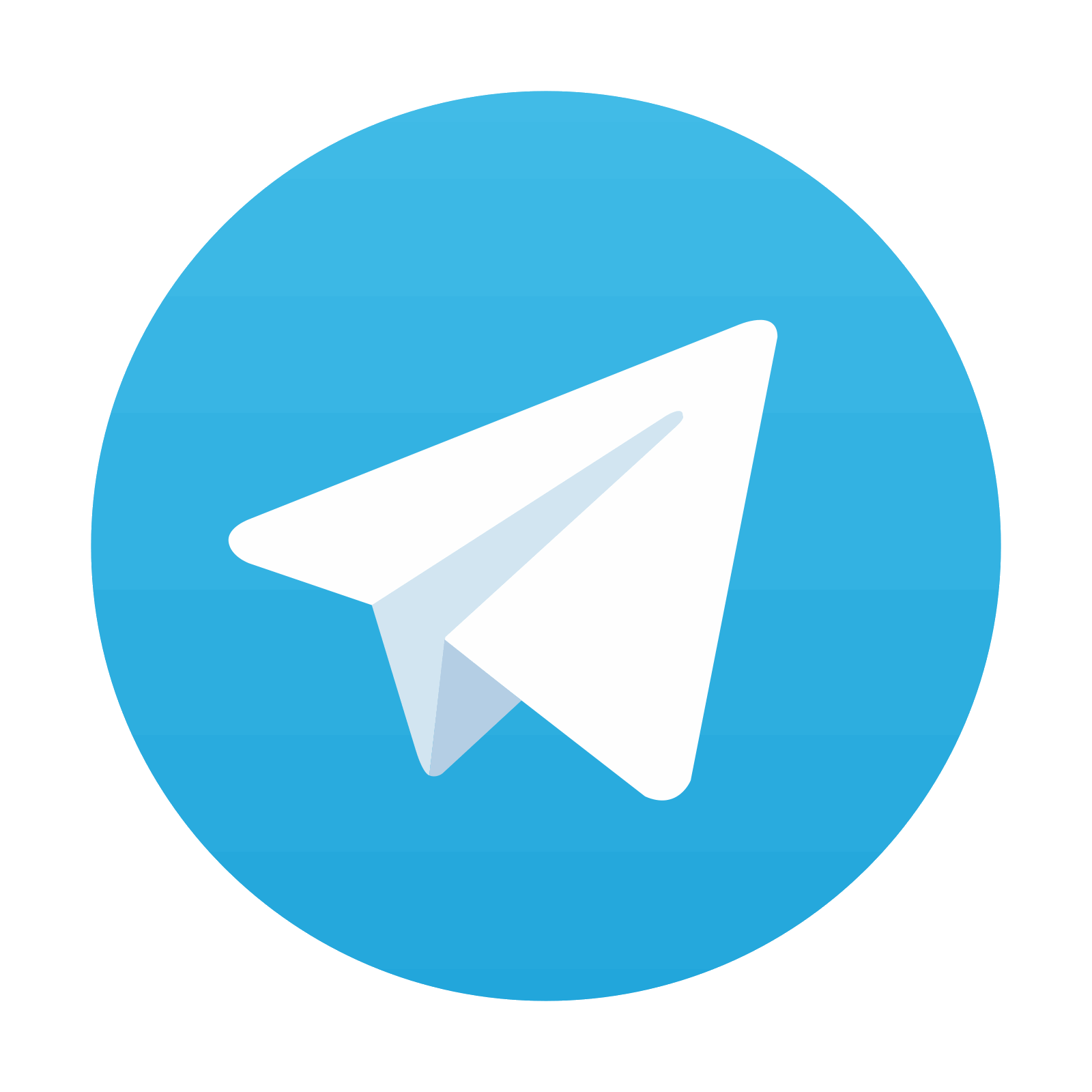
Stay updated, free articles. Join our Telegram channel
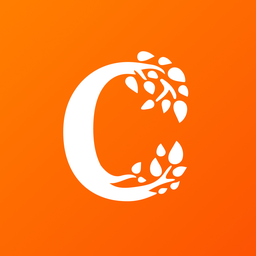
Full access? Get Clinical Tree
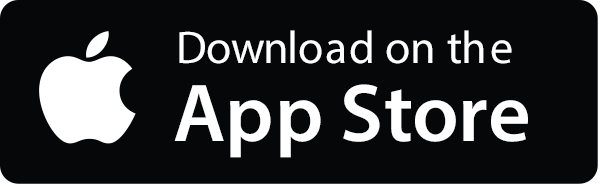
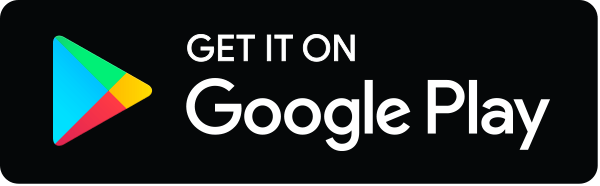