Abstract
This chapter describes the anatomy and physiology of the peritoneal membrane anatomy in normal conditions and during peritoneal dialysis. A primary area of focus is the exchange of fluid, small solutes, and macromolecules over the peritoneal membrane. The chapter discusses different factors that affect the peritoneal transport characteristics under normal conditions, as well as during different complications such as peritonitis and loss of ultrafiltration capacity. Kinetic models for peritoneal transport and clinical tests to assess the peritoneal transport capacity of individual patients are also described.
Keywords
anatomy, effluent markers, host defense, inflammation, kinetic modeling, lymphatics, peritoneal membrane, peritoneal transport, physiology
Outline
Peritoneal Anatomy, 450
Histology, 451
Peritoneal Blood Flow, 452
Peritoneal Lymphatics, 453
The Anatomy of Peritoneal Lymphatics, 453
Importance of Lymphatic Flow for Peritoneal Fluid Absorption, 453
Peritoneal Local Reaction to Infection, 453
Peritoneal Transport Physiology, 454
Barriers to Transperitoneal Exchange, 454
Modeling of Peritoneal Transport, 454
Fluid Transport, 454
Solute Transport, 457
Diffusive Transport, 457
Convective Transport, 458
Tests to Assess Peritoneal Transport, 459
Effluent Soluble Markers of the Peritoneal Membrane, 461
Factors Affecting Peritoneal Transport, 462
Effect of Body Posture on Peritoneal Transport, 462
Effect of Dialysate Composition on Peritoneal Transport, 463
Effect of pH and Different Buffers on Peritoneal Transport, 464
Effect of Biocompatible Solutions on Peritoneal Transport, 464
Pharmacological Effects on Peritoneal Transport, 464
Changes in Peritoneal Transport During Peritonitis, 464
Changes in Water and Solute Transport With Time on Peritoneal Dialysis, 465
Changes in Peritoneal Transport With Long-Term Peritoneal Dialysis, 465
Relation Between Peritoneal Transport Characteristics and Clinical Outcome, 466
Changes in Peritoneal Morphology With Time on Peritoneal Dialysis, 467
Pathophysiological Considerations, 468
In patients with chronic renal failure, waste products, which normally are excreted in the urine, accumulate in the blood, resulting in uremic intoxication, and the obvious goal of dialysis treatment is to remove uremic toxins (including water) from the patient. Peritoneal dialysis (PD) uses dialysis fluid infused into the peritoneal cavity and a system of biological membranes—the peritoneal barrier—for this purpose. Whereas the artificial hemodialysis membrane has well-characterized, reproducible, solute, and fluid transport characteristics, the peritoneum is not really a membrane but rather a complex structure of living tissues with different transport characteristics, which, furthermore, will differ between patients, a fact that will affect the fluid and solute transport kinetics of PD and dialysis efficiency in PD patients. In addition, the transport characteristics of the peritoneal membrane may not be constant in an individual patient, but may be altered with time because of effects of the dialysis procedure or the dialysis fluids in response to various physiological reactions or pharmacological effects of different drugs.
Peritoneal Anatomy
The peritoneal cavity is the largest serosal cavity in the body, with a surface area of approximately 1 to 2 m 2 . Although the peritoneal area is commonly suggested to be similar to the body surface area, recent studies suggest that the anatomical surface area of the peritoneum may be only about 50% of the body surface area in adults. Peritoneum etymologically means wrapped tightly around, which is a good description of the arrangement of this serous membrane that consists of two parts: the parietal peritoneum that covers the abdominal wall, and the diaphragm and the visceral peritoneum that covers the intraabdominal viscera. The parietal peritoneum represents a smaller portion (approximately 10% to 20%) of the total peritoneal surface area and receives its blood supply from the vasculature of the abdominal wall. The visceral peritoneum represents the larger part (approximately 80% to 90%) of the total peritoneal surface area and receives its blood supply through the mesenteric vessels. However, it should be pointed out that it is the functional peritoneal surface area that is important and not the anatomical surface area. The functional area will be related to the surface area of the capillaries in the peritoneal interstitium, the capillary density, and the spatial arrangement of these capillaries. In addition, the peritoneal cavity is only a potential space under normal conditions, and the functional contact area between the peritoneum and the dialysis fluid in the peritoneal cavity during PD will be lower than the anatomical area. In particular, the functional area of the visceral peritoneum is reduced because of the incomplete contact and poor mixing in small fluid compartments within pockets of the visceral peritoneum. In mice, less than half of the peritoneal surface is in contact with a large volume of solution in the peritoneal cavity, but the contact area could be improved by shaking the animal and, particularly, by adding dioctyl sodium sulfosuccinate (a surface tension–lowering agent).
Histology
Mesothelium
The surface of the peritoneal cavity is lined by a single layer of mesothelial cells fixed to a continuous basement membrane that, under normal physiological conditions, are covered with a thin (5 μm) film of peritoneal fluid that is kept in place by numerous microvilli. The microvilli and the peritoneal fluid have a lubricating function to prevent formation of adhesions and to allow the free movement of the visceral organs during respiration, peristalsis, and body movement. The peritoneal fluid contains protein, electrolytes, and cells (mainly macrophages, lymphocytes, and desquamated mesothelial cells) and has a high content of phospholipids that are secreted in from the mesothelium by the formation of lamellar bodies, similar to the production of surfactant from type II pneumocytes.
The mesothelial cells may modulate the peritoneal microcirculation by secretion of vasodilators like PGE 2 and nitric oxide (NO) and vasoconstrictors, such as endothelin, and, furthermore, the mesothelial cells have an important role in the initiation of the local immune response regulating leukocyte infiltration through the secretion of chemokines and expression of adhesion molecules. Mesothelial cells have a capacity to produce tissue plasminogen activator (tPA), and the mesothelium normally expresses high fibrinolytic activity. However, the mesothelium also has antifibrinolytic capacity by synthesis of fibrinolytic inhibitors like plasminogen activator inhibitor 1 (PAI-1) and PAI-2, and the balance between the synthesis of fibrinolytic activity and agents in mesothelial cells will determine their capacity to promote fibrin degradation. Under normal conditions the fibrinolytic activity strongly dominates, but the balance may change completely during inflammation, when the antifibrinolytic activity of the mesothelium will dominate, and furthermore, the mesothelium may also exhibit procoagulant activity with expression of tissue factor (which is markedly upregulated in mesothelial cells during inflammation). Thus the mesothelium plays an important role in regulating the balance between fibrinolytic and procoagulant activity in the peritoneal cavity that makes it possible to form adhesions and physically limiting the spread of intraperitoneal infections under normal conditions.
The underlying basement membrane is a very thin laminar network containing collagen type IV, proteoglycans, and glycoproteins such as laminin, and allows macrophages and lymphocytes to pass through it, whereas fibroblasts cannot pass this basement membrane. The thin mesothelial cell layer and their basement membrane seem to offer very little resistance to the transport of small and large solutes, either in vitro or in vivo. Thus the mesothelium does not seem to have any major impact on the transport across the peritoneal barrier under normal conditions.
The greater omentum is a large fold of visceral peritoneum that hangs down from the greater curvature of the stomach. It serves as a fat deposit and may, under normal conditions, physically limit the spread of intraperitoneal infections. In addition, the omentum contains “milky spots”—accumulations of mononuclear cells comprising B cells, T cells, macrophages, and mast cells. The milky spots function as secondary lymphoid organs that promote immunity to peritoneal antigens and orchestrate peritoneal cell recruitment. The number of milky spots increases markedly with PD catheter insertion, as well as with start of PD in animal experiments. They are important for the immune response during peritonitis, but their exact role is not completely clear.
Interstitium
Beneath the mesothelium lies the interstitial tissue, comprising an amorphous ground substance or gel-like extracellular matrix interlaced with collagenous, reticular, and elastic fibers; adipocytes, fibroblasts, and granular material; and containing blood capillaries, nerves, and lymphatic vessels. The collagen fibers constitute the largest component of the space between the cells in the peritoneum and form a fibrous skeleton in the interstitium. The collagen fibers bind through β 1 -integrins to fibroblasts and other cells in the tissue. The interstitial ground substance may be subdivided into a colloid-rich and a water-rich phase, with the two phases being in equilibrium with each other. The colloid-rich phase contains several different glycosaminoglycans (GAGs), including hyaluronan (HA, which is the major component). All GAGs except HA are covalently bound to a protein backbone forming proteoglycans (the combination of a GAG and a protein), for example, chondroitin sulfate, dermatan sulfate, keratan sulfate, and heparan sulfate. The GAGs are polyanionic and have low isoelectric points, and, consequently, the interstitial ground substance has a high density of negative colloidal charge at physiological pH. Water and small solutes can easily enter the colloid-rich phase, whereas macromolecules are excluded from large parts of this phase. In a complex manner, the interstitium may act as a mucopolysaccharide hydrogel, penetrated with more or less continuous channels of free fluid. Whereas small solutes may pass through interstitial matrix hydrogels without much hindrance, the diffusion of macromolecules may be markedly slowed. However, it is important to remember that the capillary wall determines the amount of solutes that are transported from blood to interstitium, and both the interstitium and the capillary wall need to be taken into account when describing the peritoneal transport process.
In general, changes in aggregation and hydration of the ground substance in interstitial tissues affect the physicochemical properties and the functional characteristics of the interstitium, but it is at present not established exactly how PD may affect the functional characteristics of the peritoneal interstitial tissues. However, thickening of the submesothelial interstitial tissue and fibrosis is common in patients on long-term PD 15,16 and may contribute to the observed long-term changes in peritoneal transport by causing uncoupling between increased small solute transport and decreased ultrafiltration coefficient, on one hand, and unchanged or slowed protein transport, on the other hand.
Capillary Wall
The microvascular exchange vessels in the peritoneal membrane consist of both true capillaries (diameter 5 to 6 μm) and postcapillary venules (diameter 7 to 20 μm), and the capillary wall is considered to be the major transport barrier for transperitoneal exchange of fluid and solutes during PD. The peritoneal capillaries belong to the continuous type (in which endothelial cells form a continuous layer enwrapped in a negatively charged glycocalyx), which functionally restrict solute exchange to less than 0.1% of the total capillary surface area (= the small pores, see later). However, the exact role of the glycocalyx is controversial. The peritoneal capillaries behave functionally as having a heteroporous structure, with a small number of large pores (radius 200 to 400 Å) through which macromolecules are filtered because of convective flow and a large number of “small pores” (radius 40 to 65 Å), which are impermeable for macromolecules larger than albumin (molecular weight 69,000 daltons) but do not restrict the passage of small solutes. In addition, “ultrasmall” pores (radius 4 to 6 Å) were postulated to be involved in the water flow induced by the osmotic effect of low-molecular-weight osmotic agents, for example, glucose ( Fig. 29.1 ). The anatomical correlate of the water channels was later demonstrated to be aquaporin-1, a protein 28 KDa intramembrane protein shown to be one of the water channels in human proximal tubular cells in the kidney and in various nonfenestrated epithelia. Aquaporin-1 has been demonstrated in peritoneal endothelial cells, at mRNA protein, and at functional levels. The anatomical correlates to the small pores are possibly the interendothelial clefts. The three-dimensional structure of the interendothelial clefts has been described in detail. However, the morphological counterpart to the large pores is not established, although it most likely corresponds to larger interendothelial gaps. Though there has been considerable controversy about the mechanism of macromolecular transport through the endothelium and the potential role of vesicular transport (transcytosis), it is now established that the quantitative role of transcytosis is negligible.

The three-pore concept of transcapillary exchange has been successfully applied by Rippe and colleagues to model the peritoneal transport of small solutes and macromolecules and the peritoneal fluid transport, supporting the view that the capillary wall is the main resistance for transperitoneal fluid and solute transport.
Peritoneal Blood Flow
The mesenteric blood flow is generally supposed to be about 10% of cardiac output. The effective peritoneal blood flow, that is, the blood flow to the capillaries that are directly involved in peritoneal transport, cannot be directly measured. Indirect estimations suggest that the effective peritoneal blood flow may vary from 20 to 40 mL/min (using estimations of the maximal possible ultrafiltration rate) to more than 100 mL/min (based on the measurements of the clearance of gases). The effective peritoneal blood flow is generally not believed to limit the clearance of small solutes during PD, because the diffusive mass transport coefficient for urea is approximately 20 mL/min. Also, tracer disappearance from small plastic chambers glued to the serosa was not reduced with a 30% decrease in blood flow and only to a minor degree with no blood flow (in dead rats). However, this issue is still controversial, and there are some observations indicating that peritoneal urea clearance may be blood flow limited. Rosengren and Rippe reported that a reduction of blood flow by 40% (caused by bleeding of rats) resulted in a decreased transport of glucose and Cr-EDTA by 13% and 24%, respectively. They concluded that there is to some extent a blood flow limitation of peritoneal transport, but that the level of blood flow limitation is much smaller than in other organs. Note that the diffusion rate of small solutes theoretically will not be proportional to the blood flow, but to the square root of the perfusion rate, which to a large extent may explain the small change in transport with marked alterations in blood flow. Vasodilators have been shown to increase peritoneal clearances because of a possible increase in the capillary surface area as a result of vasodilatation and recruitment of capillaries. Furthermore, changes in the distribution of the blood flow may also affect the peritoneal transport rate.
Peritoneal Lymphatics
The Anatomy of Peritoneal Lymphatics
About 4% of the mesothelial surface area is reported to be covered by lymphatic vessels, but the major part of the lymphatic drainage is considered to occur through the lymphatic stomata in the diaphragmatic part of the peritoneum. The diaphragmatic lymphatic stomata, which were first described by von Recklinghausen in 1862, are small openings (diameter 4 to 12 μm) that are formed by intercellular junctions between both mesothelial cells and lymphatic endothelial cells and open directly into underlying lymphatic lacunae. It is through these specific openings that large particles like erythrocytes and bacteria can directly leave the peritoneal cavity. The underlying lymphatic plexuses (which in humans are situated mainly on the muscular portion of the diaphragm) intercommunicate directly with the plexuses on the pleural surface through intercommunicating vessels. After leaving the diaphragm, the lymph is drained through the large collecting ducts associated with the internal thoracic vessels to reach the venous circulation through the right lymphatic duct. The lymphatic drainage of the peritoneal cavity is to a large extent dependent on the periodic compression and release of the lymphatic vessels caused by the movements of the diaphragm during respiration.
In addition to the lymphatic vessels in the diaphragmatic part of the peritoneum, subserosal lymphatic vessels can be found in other parts of the peritoneal cavity, including the omentum, and, furthermore, local lymphatic vessels are present in the tissues surrounding the peritoneal cavity, although their role in peritoneal transport seems to be negligible under normal conditions.
Importance of Lymphatic Flow for Peritoneal Fluid Absorption
The disappearance of a macromolecular marker from the peritoneal cavity has often wrongly been used to estimate lymph flow from the peritoneal cavity during PD. It is now well established that the peritoneal absorptive flow of fluid and solutes is composed of two different pathways : (1) direct lymphatic absorption (mainly through the lymphatic stomata in the diaphragm, and, to a lesser extent, through visceral lymphatic pathways ; and (2) fluid absorption into tissues where the fluid is absorbed through the Starling forces. Studies using tracer appearance in plasma have demonstrated that the direct lymphatic flow represents about 20% of the fluid absorptive flow from the peritoneal cavity in clinically stable peritoneal dialysis (PD) patients. (See the later discussion on fluid absorption.)
Peritoneal Local Reaction to Infection
The peritoneal host defense reaction to infection is a complex network of interactions among mesothelial cells, peritoneal macrophages (PMø), dendritic cells, infiltrating neutrophils, monocytes, and other inflammatory cells and orchestrated by the secretion of vasoactive substances, cytokines, chemokines, growth factors, and components of extracellular matrix. The initiation, resolution, and repair process of inflammation in the peritoneal cavity are very complex processes, which presently are under intense study, and the regulation of these processes is starting to be understood.
The initial inflammatory activation by bacteria entering the peritoneal cavity is likely to occur on the mesothelial surface, where mesothelial cells, together with dendritic cells and PMø, have an important role in the initiation of the local immune response. The characteristics of the antigen-presenting cells are starting to be understood. The mesothelial cells are able to contribute to the massive neutrophil influx by generation of chemokines like CXCL8 (previously called interleukin-8 ), a process that is amplified by the PMø-derived cytokines tumor necrosis factor-α (TNF-α) and interleukin (IL)-1β; and the mesothelial cells are also capable of expressing adhesion molecules like ICAM-1, VCAM-1, PECAM-1 and integrins, which may promote leukocyte attachment to the mesothelial cells. The PMø–produced TNF-α and IL-1β are thought to be key mediators in the activation of mesothelial cells. The mesothelial cells are the principal source of IL-6 in the peritoneal cavity and synthesize large amounts of IL-6 upon inflammatory challenge. However, mesothelial cells do not express the cognate IL-6 receptor, and therefore they initially are unresponsive to IL-6. There is a rapid accumulation of neutrophils within the peritoneal cavity during the first 12 to 24 hours. The proinflammatory cytokines will also lead to differentiation and activation of dendritic cell precursors in the peritoneum into mature dendritic cells that migrate to the lymphoid tissue to active adaptive immunity. The activated Th1 cells and resident natural killer cells will produce interferon-γ, which upregulates the bactericidal activity of the peritoneal macrophages and is also involved in the recruitment and clearance of neutrophils. Early upregulation of the receptor-interacting protein-2 (RIP2) in peritoneal macrophages is required for the rapid resolution of peritonitis, and RIP2 expression likely is required for the decrease of intracellular infection and regulation of migration of antigen-presenting cells. RIP2 has been suggested as a biomarker for resolution of peritonitis to be used in the clinic.
After a few days the neutrophils are replaced by a more sustained population of monocytes and lymphocytes. In fact, this temporal switch in the recruitment of leucocytes (which is under complex regulation) determines whether or not the infection is cleared. Liberation of the soluble IL-6 receptor (SIL-6R) from the initial neutrophils allows for the formation of the IL-6 and SIL-6R complex that allows IL-6 responsiveness in cell types (including mesothelial cells) lacking the cognate IL-6 receptor. The IL-6 and SIL-6R complex will downregulate the expression of CXCL8 and other neutrophil-activating chemokines, and the SIL-6R may also directly promote MCP-1 expression, resulting in the more sustained mononuclear leukocyte infiltration. In addition, the release of oncostatin M from the infiltrating neutrophils will have a synergistic effect with the SIL-6R for the temporal switch from neutrophil influx to mononuclear cell recruitment as oncostatin M suppresses IL-1β–mediated expression of CXCL8. Interferon-γ also has an important role in this process by controlling both the initial neutrophil recruitment independently of IL-6 (through regulation of chemokine expression) and the neutrophil clearance phase by regulating local IL-6 levels. The neutrophils will, to a large extent, undergo apoptosis and then be phagocytized by mononuclear cells. This transition from the recruitment of neutrophils (typically associated with innate immunity) to the leukocytes typically associated with acquired immunity is considered to facilitate the successful resolution of the inflammatory reaction.
Peritoneal Transport Physiology
Barriers to Transperitoneal Exchange
The peritoneum is a complex structure of at least five different resistance barriers coupled in a series: (1) the unstirred fluid layer in the capillaries, (2) the capillary wall (endothelium and basement membrane), (3) the interstitial space, (4) the mesothelium and its basement membrane, and (5) the unstirred fluid layers in the peritoneal cavity. Each of these barriers has its specific transport properties. The capillary wall is considered to represent the major transport barrier for transperitoneal exchange, but the interstitium is also important, particularly in long-term PD patients with markedly increased thickness of the submesothelial tissue. The mesothelium, on the other hand, is highly permeable and is not a significant transport barrier. The mucopolysaccharide hydrogel of the interstitium will exclude solutes from part of the interstitial water volume and force solutes to follow a tortuous path, and furthermore, the negative charge of the interstitial ground substance may markedly slow the transport of charged molecules through the interstitium. Unstirred fluid layers in the peritoneal cavity may represent transport resistances for the diffusion of small solutes, but are likely of much less importance than the interstitium because the diffusibility is much less in the interstitium compared with the stagnant fluid layers.
Modeling of Peritoneal Transport
To completely model the peritoneal transport process, all transport barriers and their specific transport characteristics should be taken into account, including the distribution of the capillaries within the peritoneal interstitium. This will result in very complex models that are difficult to apply in the clinical situation, and, at present, even complex models fail to predict ultrafiltration with better accuracy than simpler models.
Single-membrane models have been used to estimate transport parameters in clinical PD. In the single-membrane models, the peritoneal barrier is regarded as a single membrane separating the well-mixed blood and dialysate compartments. The single-membrane models will work very well to describe the transport of small solutes (up to the size of small proteins like β 2 -microglobulin) from plasma to dialysate, but they will not work as well for the description of dialysate to plasma transport, and in particular, they cannot correctly describe the osmotic fluid transport when a high-molecular solute (e.g., icodextrin) is used as an osmotic agent. The distributed model by Flessner et al. takes into account the distribution of capillaries in the interstitium and should be preferred from a theoretical point of view. However, the simpler three-pore model by Rippe and colleagues, which takes into account the three pore systems in the capillary wall (see earlier text), is still as accurate in predicting both fluid and solute transport during clinical PD, using both small-molecular-weight and macromolecular osmotic agents ( Fig. 29.2 ). Also, a model describing the peritoneum as two heteroporous membranes in series (presumably the capillary wall and the interstitium) has been developed. This model was further developed based on the current understanding of the long-term changes in peritoneal membrane, combining the concept of the capillary three-pore model with an interstitial fiber-matrix barrier, and has been successful in describing the long-term changes in peritoneal transport of fluid (with uncoupling of small solute transport from the peritoneal ultrafiltration coefficient) and of small and large solutes. A detailed description of the different models lies outside the scope of this chapter.

Fluid Transport
Ultrafiltration
The intraperitoneal dialysate volume over time curves during a PD exchange are characterized by three phases: (1) initial net ultrafiltration (rate and duration depend on the osmotic pressure of the solution); (2) dialysate isovolemia (during which ultrafiltration is counterbalanced by fluid absorption); and (3) net fluid absorption (independent of the osmolality of the solution) ( Fig. 29.3 ).

Ultrafiltration in PD is achieved by the application of a high concentration of an osmotic agent (usually glucose) in the dialysate, resulting in a high osmotic pressure gradient across the peritoneal barrier. However, the osmotic pressure gradient across the peritoneal barrier decreases rapidly because of the absorption of the osmotic agent when small solutes like glucose, amino acids, or glycerol are used as osmotic agents. When a large molecular solute—for example, icodextrin—is used as an osmotic agent, the absorption of the osmotic agent is much slower, resulting in a much longer-lasting osmotic gradient and positive net ultrafiltration.
Applying the thermodynamic theory of volume transport through selective membranes to the peritoneal membrane, the ultrafiltration rate (Q U ) is directly proportional to the ultrafiltration coefficient (L P A), which, in turn, represents the product of the hydraulic conductance (L P ) and the effective surface area (A). The ultrafiltration rate is therefore described as:
Q U = L P A ( Δ P − σ prot Δ π prot − ∑ i = 1 n σ i Δ π i )
where ΔP is the hydrostatic pressure gradient, σ prot is the reflection coefficient for total protein, Δπ prot is the colloid osmotic pressure difference caused by the plasma proteins, and the third term within the parentheses represents the sum of all effective crystalloid osmotic pressure gradients across the peritoneal barrier. Note that this equation is a simplification that applies to the capillary wall and, for the full description of the total process, local effects in peritoneal tissue (e.g., the distribution capillaries in the interstitium, interstitial tissue pressure gradients) also will have an impact on the ultrafiltration rate. Thus the ultrafiltration induced when glucose is used as an osmotic agent in PD is dependent on the osmotic pressure difference for glucose, the hydraulic conductance (L P ), the surface area (A), and the reflection coefficient for glucose (σ g ). A wide range of values for the ultrafiltration coefficient (L P A) has been reported in the literature because markedly different values of σ g have been estimated. The reason for these discrepancies is that σ g in several studies has been calculated as σ g = 1 − S g (where S g is the “lumped” glucose sieving coefficient for the whole peritoneal membrane), which is true for homogeneous membranes. However, as the peritoneal membrane is a heteroporous membrane, the relationship between σ g and S g may vary. In fact, one of the most convincing arguments for the heteroporous character of the peritoneal membrane is that the direct determinations of L P A for the peritoneal membrane (assuming σ albumin = 0.9) in cats and rats (scaled to humans using the scaling factor body weight (BW) 0.7 ) yielded an L P A value of approximately 0.1 mL/min/mmHg, which, in turn, yielded a σ g of approximately 0.02. These values are well in agreement with the reported initial ultrafiltration rate of 10 to 20 mL/min with 3.86% glucose solution (4.25% dextrose).
For the peritoneal barrier, the total σ of a solute is equal to the sum of the product of σ and the fractional hydraulic conductivity α for each set of pores. Thus when applying the three-pore model for the peritoneal membrane, the total σ for a solute will be given by the equation:
σ = α A × σ A + α S × σ S + α L × σ L
where subscripts A, S, and L denote aquaporins, small pores, and large pores, respectively. Because the aquaporins are impermeable for glucose, σ A =1 for glucose across the aquaporins, whereas the relative osmotic efficiency of glucose is much less across the small pores (σS = 0.03) and negligible across the large pores (σL approximately 0). During normal conditions, the aquaporins account only for a small fraction (approximately 2%) of the LpA, and they will play a minor role in fluid transport, whereas the small pores account for about 90% of LpA. However, when applying a high crystalloid osmotic pressure over the membrane by using a small-molecular osmotic agent, the importance of the aquaporins for fluid transport markedly increases. As α ⋅ σ will be quite similar for the aquaporins (0.02 ⋅ 1 = 0.02) and the small pores, (0.90 ⋅ 0.03 = 0.027) about half of the ultrafiltration will pass through the aquaporins, resulting in marked sieving of solutes (for the large pores, α ⋅ σ will approximately be zero, as σ L is approximately 0).
Fluid Absorption
During PD, ultrafiltration is partly counterbalanced by the peritoneal fluid absorption (Q A ). Thus the net change in dialysate volume (V D ) is equal to:
d V D d t = Q U − Q A
Because Q A is considered to be bulk flow, it can be estimated by the disappearance rate (K E ) of a macromolecular marker applied in the dialysate (see Ref. 99 for a detailed discussion).
Pathways for Peritoneal Absorptive Flow
The peritoneal absorptive flow consists of two different pathways : (1) direct lymphatic absorption (through the lymphatic stomata mainly in the diaphragm and, to a lesser extent, through visceral lymphatic pathways) ; and (2) fluid absorption into tissues (where the fluid is absorbed into the capillaries because of the Starling forces, whereas the macromolecules are absorbed slowly through local lymphatics). Sieving of macromolecules is assumed to be negligible with the direct lymphatic absorption, and with the second pathway (fluid absorption into tissues), sieving of macromolecules at the site of the mesothelium is considered to be negligible from a practical point of view. Thus the macromolecular disappearance rate from the peritoneal cavity may be used as an estimate of the peritoneal bulk absorptive flow because it is mainly dependent on the two components of peritoneal absorption, which both are considered to be bulk flows. When the fluid, which has entered the peritoneal interstitial tissue compartment, is absorbed across the capillary wall (because of the Starling forces), sieving of macromolecules should occur at the site of the capillary wall. It is generally agreed that almost no protein may enter the plasma compartment directly through the capillary wall (although direct capillary uptake of radioiodinated human serum albumin [RISA] has been demonstrated under certain conditions). The macromolecules that have entered the interstitial tissue compartment may thus accumulate in the interstitial tissue compartment before they are slowly absorbed by local lymphatics.
The peritoneal absorptive flow is independent of the intraperitoneal osmotic pressure and thus not influenced by ultrafiltration induced by the osmotic agent in the dialysate (i.e., osmotic pressure–driven convective flow). On the other hand, the peritoneal fluid and protein absorption rate in animal experiments has been shown to be directly proportional to the intraperitoneal hydrostatic pressure. Studies by Flessner of tissue concentration profiles of RISA and labeled immunoglobulin G (IgG) (absorbed from the peritoneal cavity in rats) strongly support the notion that hydrostatic pressure–driven convection is the most likely mechanism driving the fluid and protein transport into adjacent tissues. It may seem puzzling that osmotic pressure–driven convection during dialysis and hydrostatic pressure–driven convection are considered to go simultaneously in different directions through the peritoneal barrier without any major interaction. However, this apparent paradox may be explained by the nonhomogenous nature of the peritoneal barrier, where different parts have different vascularization, hydrostatic pressure gradients, and so forth. Furthermore, osmotic pressure–driven convection will take place close to the capillaries only, whereas the major part of the peritoneal surface area will allow hydrostatic pressure–driven convection in the opposite direction.
Relative Importance of Lymphatic Absorption and Absorption into Adjacent Tissues
The relative contribution of the two different components of peritoneal absorptive flow (lymphatic absorption and absorption into tissues) has been controversial. In fact, the disappearance rate of a macromolecular marker has previously been assumed to provide an estimate of the lymphatic absorption rate in PD patients. However, several studies have shown that the plasma appearance rate of a macromolecular marker is, on average, only about 10% to 20% of its disappearance rate from the peritoneal dialysate (in clinically stable PD patients and in animals). Furthermore, studies in animals have demonstrated that a major part of the lost marker accumulates inside the tissues adjacent to the peritoneal cavity, mainly in the liver, diaphragm, and anterior abdominal wall. Thus the interstitial adjacent tissues may serve as a reservoir for RISA, from which it is slowly absorbed into local lymphatic vessels.
Theoretically, it is also possible that RISA transport is delayed in lymph nodes compared with the fluid accompanying RISA in the lymphatic vessels. However, trapping in lymph nodes has not been found to be of major importance, and furthermore, this would not explain the high tissue concentrations of macromolecular tracers reported by Flessner from studies in the rat.
Solute Transport
During PD, solutes are transported bidirectionally through the peritoneal barrier mainly by diffusion (as a result of the concentration gradient between blood and dialysate) and, to a lesser extent, by convection into the peritoneal cavity (as a result of hydrostatic pressure differences and the osmotic disequilibrium caused by the osmotic agent). Also, the solute transport accompanying the convective fluid absorption from the peritoneal cavity into the surrounding tissues and to blood through the lymphatics (see earlier mention) needs to be taken into account.
Diffusive Transport
Diffusive transport through a membrane is driven by the concentration gradient over the membrane. If diffusion is unrestricted, the solute transfer rate (J S ) is proportional to the concentration gradient between dialysate and plasma (ΔC), the solute’s diffusion constant (D, which is inversely proportional to the solute’s radius), the surface area available for diffusion (A), and inversely proportional to the diffusion distance (Δx) :
J S = D Δ x A Δ C
The ratio of the solute’s diffusion constant to the diffusion distance (D/Δx) is called permeability (P), and the product of P and surface area is usually denoted permeability surface area product (PS), which in PD has also been denoted as diffusive mass transport coefficient (K BD ), mass transfer coefficient (MTC), or mass transfer area coefficient (MTAC). Thus PS = K BD = MTC = MTAC. Inserting PS into Eq. 4 yields the following description of diffusive solute transfer rate for a solute across the peritoneal barrier:
J S = PS Δ C
However, because the peritoneal barrier behaves as a porous structure, the diffusion of a solute may be restricted by the pore passage; the solute has to hit the pore entrance area and may also be restricted by interaction because of friction with the pore wall. The diffusion through the peritoneal barrier will therefore be restricted, and a restriction factor (A/A 0 ) needs to be introduced (where A is equal to the apparent pore surface area and A 0 the total cross-sectional pore surface area), and inserting this into Eq. 4 yields:
J S = D A Δ x Δ C = D A 0 Δ x A A 0 Δ C
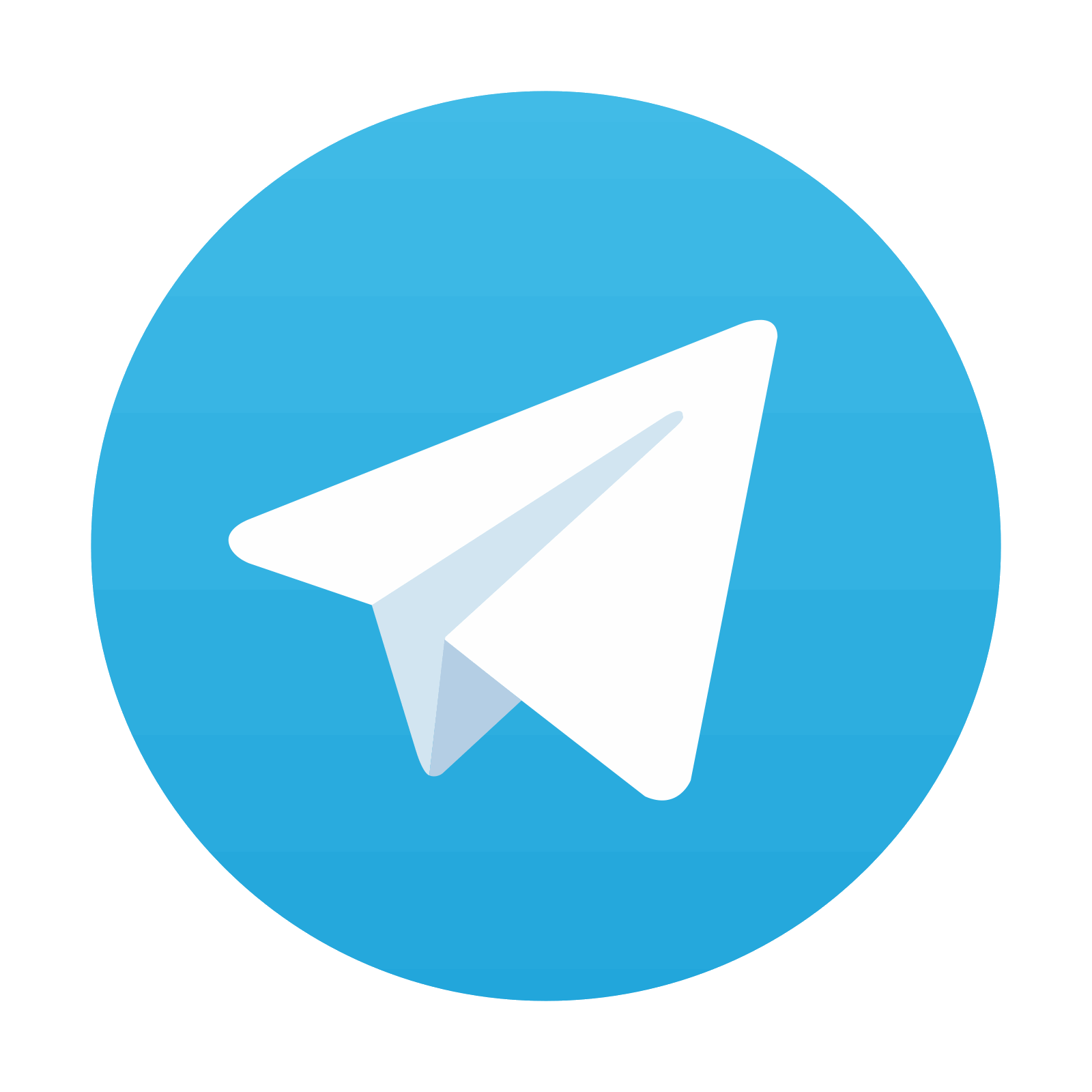
Stay updated, free articles. Join our Telegram channel
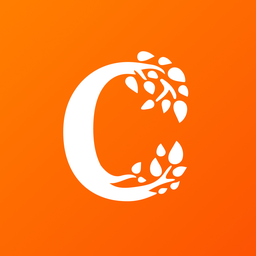
Full access? Get Clinical Tree
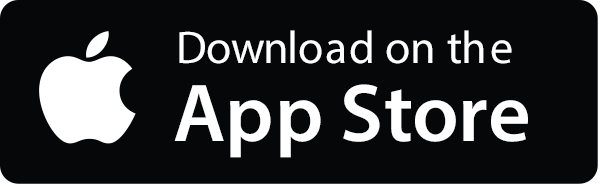
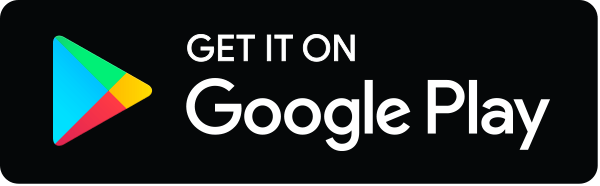