Buds Form and Fuse
The pancreas arises early in gestation from the posterior foregut endoderm. A dorsal and a ventral bud first form before migrating and eventually fusing to form a single tissue. Specifically at day 26 of gestation, the foregut evaginates into a condensation of overlying mesenchyme to form the first morphological evidence of the dorsal bud; six days later, a ventral bud forms. At five to six weeks of gestation, the ventral pancreas rotates around the duodenum and fuses with the dorsal pancreas (see Figure 80-1 ).

Ductal System Forms
The adult pancreatic ductal system is contributed to by each of the two buds. The dorsal bud forms the distal portion of the main pancreatic duct (of Wirsung) and the entire portion of the minor accessory duct (of Santorini) ( Figure 80-1 ). The ventral bud provides the proximal portion of the main pancreatic duct. Pancreas divisum, discussed further in Chapter 81 , occurs when the two buds and their ductal systems, fail to fuse.
Annular pancreas is an anomaly in which the ventral pancreas fails to fully rotate and instead forms a ring of pancreatic tissue that constricts the duodenum. This condition presents in roughly half of patients during the neonatal period, with high-grade small bowel obstruction. An alternative hypothesis for annular pancreas formation is that the pancreas abnormally hypertrophies around the duodenum during development. For example, mice lacking the signaling molecule indian hedgehog (Ihh) develop increased ventral pancreatic mass, and just under half form an annulus around the duodenum. The clinical features of annular pancreas are detailed in Chapter 81 .
Differentiation of Pancreatic Progenitors
A remarkable feature of pancreatic differentiation is that all three major parenchymal cell types –including the two exocrine cells, the acinar and duct cells, and the endocrine islet cells – originate from a common progenitor ( Figure 80-2 ).

Programmed Development by Hierarchical Signals
Two major types of signals control the delicate morphological changes in the pancreas as well as the cellular growth, expansion and differentiation of pancreatic progenitors. They include (1) a repertoire of transcription factors and (2) inductive signals from adjacent structures and tissues.
Transcriptional Factors Mediate Pancreatic Development
Transcription factors are identified by their DNA-binding domains, and they either activate or suppress gene transcription. A summary of a few key factors is provided below.
PDX1
The transcription factor PDX1 or Pancreatic and duodenal homeobox 1, identifies early pancreatic progenitors. Absence of PDX1 expression results in pancreatic agenesis.
During adulthood, PDX1 expression becomes largely confined to the beta cells, where it binds to and activates the insulin promoter. Conditional inactivation of Pdx1 in adult mouse beta cells results in diabetes. In summary, PDX1 is required for proper pancreatic development as well as beta cell function.
PTF1a
PTF1a, or the alpha subunit of pancreas-specific transcription factor 1, is a basic helix-loop-helix transcription factor that initially contributes to the specification of all three pancreatic precursors, but later in development its serves to promote acinar cell maturation.
Ptf1a deficiency in mice results in pancreatic agenesis with complete absence of exocrine development, but ectopic endocrine cells can still be found. In a human pedigree, a truncating single nucleotide mutation in PTF1a led to pancreatic and cerebellar agenesis.
MIST1
The muscle, intestine and stomach expression 1 (MIST1) is a basic helix-loop-helix transcription factor whose expression is restricted to acinar cells in the post-natal state. MIST1 is thought to function in the maintenance of acinar cell polarization. Ablation of Mist1 still allows the development of acinar cells, but they are more sensitive to injury and more prone to neoplastic transformation.
SOX9
The SRY-box 9 (SOX9) is a high mobility group protein. This transcription factor is essential for the secondary transition in pancreatic development. Lineage tracing experiments demonstrate that Sox9 is expressed in pancreatic progenitor cells. However, Sox9 expression in the mature pancreas is limited to the duct epithelium.
Additional transcriptions factors involved in endocrine specification include MAFA, ISL1, BRN-4, NEUROD, NKX6.1, NKX2.2, PAX4, PAX6, and ARX.
Signals from Adjacent Structures and Tissues
In addition to the critical role of transcription factors expressed from within the developing pancreas, fluctuating levels of signals from adjacent tissues and structures also control pancreatic development. Early on, pancreas specification from endoderm requires suppression of Wnt and fibroblast growth factor 4 (FGF4) signaling from adjacent mesoderm. Additionally, mesodermal retinoic acid signals refine the anterior-posterior position in which the pancreas develops.
The notochord, which early in development is in proximity to the dorsal pancreatic bud permits bud specification by suppressing the anti-pancreatic factor sonic hedgehog (Shh). By contrast, ventral bud development requires withdrawal of FGF signaling from nearby cardiac mesenchyme.
Endothelial Cells
At a later period endothelial tissue, such as dorsal aorta and the vitelline veins, influence pancreatic development. For example, the dorsal aorta could induce Pdx1 and insulin expression in isolated endoderm. In addition to points of endodermal-endothelial contact, sphingosine-1-phosphate secreted by endothelial cells in the circulation promotes dorsal pancreatic budding.
Pancreatic Mesenchyme and Stroma
Growth factors secreted by pancreatic mesenchymal cells are also important to development of the endodermal primordium. The presence of mesenchyme shifts the growth state of pancreatic progenitors from differentiation to proliferation. Notably, the mesenchymal factor FGF-10 promotes proliferation. Fgf-10-deficient mice exhibit severe growth retardation of the pancreas. Conversely, overexpression of Fgf-10 controlled by the Pdx1 promoter results in sustained proliferation rather than differentiation. Fgf-10 signaling causes activation of the Notch pathway. Mice deficient in various Notch signaling components show accelerated differentiation and less proliferation, resulting in pancreas hypoplasia. Overexpression of Notch1 in pancreatic epithelium prevents endocrine and exocrine differentiation. The Notch target, Hes1 prevents p57-mediated cell cycle exit, is required for differentiation during the early stages of pancreas formation. The importance of ensuring pancreatic progenitor proliferation is underscored by the finding that the final size of the pancreas is determined by the original number of progenitor cells present at an early embryonic stage. Stromal components also influence the pancreas during development. For example, neural crest cells that migrate into the pancreas influence the number of beta cells. Finally, it is also likely that, conversely, the pancreas contributes instructive signals to its adjacent tissues; however this postulate has not been well characterized.
Epigenetic Control
The epigenetic modification of chromatin by either loosening or compacting its structure via histone acetylation or deacetylation, respectively, constitutes an important mode of guiding pancreatic development. Both expression and activity of histone deacetylase (HDAC) are reduced over embryonic life into adulthood in the rat pancreas. Using an in vitro embryonic culture method of the pancreas, pharmacologic inhibition of HDAC classes I and II abolish acinar cell differentiation but enhance ductal and beta cell pools. The results indicate a role for histone modification in determining pancreatic cell fate.
Regenerative Capacity of the Pancreas
The importance of understanding early pancreatic development is underscored by work that shows various aspects of pancreatic development re-emerge in certain conditions. This re-emergence is not simply an epi-phenomenon, but is necessary for the regenerative component of recovery. Regeneration can occur to varying degrees in the pancreas during pregnancy, partial pancreatectomy, or pancreatitis.
Regenerative Capacity After Pancreatitis
A prime example of this regenerative potential occurs after the injury of pancreatitis. Pancreatitis induced in mice by intraperitoneal administration over a 2-day period with high concentrations of a cholecystokinin analog results in about a 50% dropout of acinar cells. However, 7 days after the injury, the exocrine gland is almost completely restored. In response to the injury, the pancreas activates regenerative processes to reestablish tissue mass. Whether the regeneration of exocrine tissue takes place through cell replication or through neogenesis is unclear. The prevailing notion is that, after injury, the surviving acinar cells replicate to give rise to new acinar cells. However, neogenesis can arise in at least 2 ways. Mature cells can re-express early developmental factors to take on the role of “facultative” progenitors ( Figure 80-3 ). Alternatively, progenitor niches might exist within ducts or at the junction of duct cells and acinar cells. The role of acinar cells as facultative progenitors is discussed briefly below.

Reemergence of Embryonic Transcriptional Factors
Acinar cells that survive pancreatic injury induce genes that are normally associated with early embryonic progenitor cells, including Pdx1 and Notch pathway components such as Notch1, Notch2, Jagged2, and Hes1. In addition, β-catenin signalling is activated, which is a marker that is normally found in undifferentiated, proliferating exocrine progenitor cells. Reactivation of the Notch signaling pathway during injury from cerulein-induced pancreatitis is required for acinar cell regeneration. Mice that were either chemically treated with a γ-secretase inhibitor to block Notch receptor cleavage or were conditionally deficient in Notch1 had fewer mature acinar cells during recovery from acute pancreatitis.
In addition, embryonic signaling by Hedgehog is upregulated in acinar cells after cerulein-induced pancreatitis. Hedgehog blockade, either pharmacologically or genetically using Pdx1- or elastase-Cre-recombinase, allows the formation of a ductal epithelium from acinar cells, but it does not permit redifferentiation into acini. Intriguingly, it was suggested that the “redifferentiation arrest” might provide a link between pancreatitis injury and subsequent neoplasia. The results also underscore the capacity of the acinar cell to revert to an earlier progenitor state in response to injury.
Significance of Pancreatic Development on Postnatal Pancreatic Disorders
The degree to which regeneration of the pancreas occurs after pancreatitis may turn out to be as important in determining the outcome of the disease as the level of inflammation induced by the injury. Thus, these and other studies might in the near future be utilized to enhance the recovery phase after pancreatitis and cause a net improvement in pancreatitis outcome.
The pluripotential of the pancreas has been utilized to cause trans-differentiation of acinar cells to beta cells that can secrete insulin. Adenoviral vectors expressing early progenitor and islet transcription factors Pdx1, Neurogenin3, and Mafa were directly injected into the tail of the pancreas in vivo. They primarily infected acinar cells and within days, more than 20% of former acinar cells, evidenced by genetic lineage tracing, began to express insulin and assumed beta-cell morphology. The results confirm the potential of the acinar cell to undergo forced transdifferentiation. They also offer the prospect of an endogenous pancreatic source of beta cells to treat diabetes. By borrowing genetic cues from a basic understanding of pancreatic development, this ingenious approach might provide a new paradigm to treat type 1 diabetes using the large acinar cell reserve.
Reemergence of Embryonic Transcriptional Factors or Their Forced Expression Can Result in Metaplasia
The change of acinar cells to either a progenitor-like state or another cell type, however, runs the risk of malignant transformation. Mounting evidence suggests that pancreatic ductal adenocarcinoma (PDAC) and it noninvasive precursor lesions known as pancreatic intraepithelial neoplasia (PanIN) are the result of acinar cell metaplasia to a ductal cell form. Conditional expression of oncogenic Kras along with Notch using Pdx1 Cre-recombinase synergistically causes mature acinar cells to convert to PanIN lesions. The finding further supports the theory that PDAC can arise from acinar or acinar-like cells when early embryonic factors reemerge.
Summary on Pancreatic Development
In summary, the pancreas is formed from the fusion of ventral and dorsal endodermal buds. All three pancreatic parenchymal cells arise from a common pancreatic progenitor cell. Pancreatic development is delicately controlled by the temporal expression of various transcriptional factors as well as the secretion of factors produced by adjacent tissues and mesenchyme. Factors observed primarily during embryonic development might reemerge in response to injury after pancreatitis. Harnessing the pluripotent capacity of the pancreas may yield future therapies aimed at enhancing recovery from injurious states. Thus, an improved understanding of pancreatic development may provide crucial translational tools to treat pancreatic disorders in both children and adults.
Pancreatic Secretion and Exocrine Function
Overview of Pancreatic Physiology
The pancreas is a vital exocrine and endocrine organ responsible for the release of hormones and the secretion of fluid, electrolytes, and enzymes that are intricately necessary for the digestion of food and glucose homeostasis. The remainder of this chapter will specifically focus on regulation of exocrine pancreatic secretion.
Functional Anatomy of the Exocrine Pancreas
More than 80% of the exocrine pancreas is composed of acini, which are a cluster of pyramidal acinar cells arranged with their apical membranes oriented toward a central lumen ( Figure 80-4 ). Acinar cells are identified by the presence of dense zymogen granules (ZGs) in the apical region. The basal region of the acinar cell contains the nucleus and ER, where proteins and digestive enzymes are synthesized. These enzymes are sorted and packaged into secretory vesicles in the Golgi complex. The vacuoles eventually bud off and mature into ZGs. The entire process from enzymatic synthesis to the point at which enzymes are ready for secretion takes about 50 minutes. The rate of synthesis is 10 million enzyme molecules per acinar cell per minute, which is among the fastest of any mammalian cell.

The ER is not only responsible for protein translation but is also involved in the regulation of cell signaling during times of stimulation or stress. The ER acts to store Ca 2+ , which is an important signaling molecule for enzyme secretion into the ductal lumen during digestion. Aberrant Ca 2+ signals, however, are implicated during pathologic processes, such as premature protease activation. The ER is also involved in regulating acinar cell stress by monitoring the kinetics of protein folding. Protein folding occurs largely in the ER and is influenced by many cellular stressors, including changes in pH, Ca 2+ , chaperones, and oxidative stress. The ER monitors stress responses and mediates the removal of misfolded proteins by an unfolded protein response (UPR) mechanism that works primarily through three sensing proteins: inositol-requiring protein-1 (IRE1), activating transcription factor-6 (ATF6), and protein kinase RNA (PKR)–like ER kinase (PERK). These three proteins interact with Binding immunoglobulin protein (BIP) and X-box binding protein 1 (XBP1) within the ER lumen to allow regulation of the UPR. At low levels of ER stress, the UPR increases the availability of chaperones involved in proper protein folding as well as causing the ER to enlarge to accommodate the need for increased protein synthesis. However, at high levels of ER stress, other mechanisms are initiated such as the NF-kB pathway, which leads to cell apoptosis.
Additional processing in the form of glycosylation occurs in the Golgi apparatus as proteins traffic to their storage locations. Upon receiving secretory signals, ZGs fuse with the apical membrane through complex transmembrane interactions called SNARE complexes, which results in the exocytosis of digestive enzymes into the duct lumen.
The basolateral membrane of the acinar cell harbors multiple receptors for secretagogues and neurotransmitters, notably acetylcholine, which regulate exocytosis.
The endocrine cells of the pancreas are contained within the islets of Langerhans, which are embedded within the exocrine acinar milieu. Islet cell hormones enter the systemic circulation via pancreatic blood flow through a complex capillary network associated with islets. Acinar cells are exposed to endocrine secretions (in a reciprocal relationship) via cell-to-cell contact as well as by direct capillary connections between the islets and acini within an insulinoacinar portal system. The pancreas has a complex neuronal network composed of parasympathetic (vagal), sympathetic, peptidergic, and sensory innervations of glandular cells and vessels. In addition, the pancreas also has its own intrinsic nerve plexus, comparable to the enteric nervous system.
Formation of Pancreatic Juice
About one liter of isotonic, alkaline pancreatic juice is secreted into the small intestine per day. The centroacinar and ductal cells are responsible for the secretion of the bicarbonate (HCO 3 − ) rich fluid that transports the digestive enzymes into the small intestine ( Figure 80-5 ).

Fluid and Electrolytes
Duct cells are responsible for the complex transport of electrolytes, including sodium (Na + ), potassium (K + ), HCO 3 − , and chloride (Cl − ) into the pancreatic duct lumen. HCO 3 − is generated in two ways: (1) HCO 3 − can be transported across the basolateral membrane of the duct cell via an Na + /HCO 3 − cotransporter, and (2) it can also be enzymatically produced by carbonic anhydrase from CO 2 and H 2 O. A Cl − /HCO 3 − antiporter is primarily responsible for driving HCO 3 − out of the cell and into the duct lumen. High extracellular Cl − at the apical membrane is generated by a cAMP-, Ca 2+ -dependent Cl − channel, the cystic fibrosis transmembrane conductance regulator (CFTR). HCO 3 − in pancreatic juice alters luminal pH for optimal digestion within the small intestine.
At rest, pancreatic juice is secreted at 0.2 mL per minute, and the bicarbonate concentration equals that of plasma. During secretin-induced stimulation, the rate of secretion increases 20-fold to about 4.0 mL per minute, and the HCO 3 − concentration increases asymptotically to a maximum of 140 mEq/L, creating a pH of about 8.2 in pancreatic juice. The sum of Cl − and HCO 3 − concentrations remains constant as the Cl − concentration falls with increasing secretion rates. Pancreatic juice also contains Ca 2+ and small amounts of magnesium (Mg 2+ ), zinc (Zn 2+ ), phosphate (HPO 4 − ), and sulfate (SO 4 2− ).
Pancreatic Enzymes
Pancreatic juice is composed of digestive enzymes, plasma proteins, trypsin inhibitors, and mucoproteins in an HCO 3 − -rich fluid. The pancreatic enzymes are responsible for about half of all the digestion of nutrients within the gastrointestinal tract. The digestive enzymes can be classified as proteolytic, lipolytic, and amylolytic enzymes ( Figure 80-6 ).

Proteases
The proteolytic enzymes, or proteases, are proenzymes, or zymogens, and the most abundant is trypsinogen, which makes up 19% of the total protein content in juice. The three major forms of trypsinogen are the cationic trypsinogen (PRSS1), anionic trypsinogen (PRSS2), and mesotrypsinogen (PRSS3). Trypsinogen, along with the other zymogens, is proteolyzed to its active form, trypsin, by exposure to enterokinase on the intestinal brush border. Trypsin in turn proteolytically activates the other zymogens, including chymotrypsinogen, proelastase, and procarboxypeptidase A and B.
Trypsin cleaves interior peptide bonds associated with basic amino acids. Chymotrypsin acts on interior peptide bonds involving the aromatic amino acids as well as leucine, glutamine, and methionine. Elastase cleaves peptide bonds at neutral aliphatic amino acids. Carboxypeptidase cleaves peptide bonds at the carboxy-terminal ends of proteins. The combined action of the activated pancreatic proteases, along with gastric pepsin, allows for the digestion of proteins into oligopeptides and amino acids, which can be transported across the intestinal brush border. There is overlap in the sites of cleavage for several of these proteases, a point that highlights the importance of protein digestion. The activation of the bulk of trypsinogen in the intestine is a seminal step in the physiologic activation of the zymogen cascade. In addition to the requirement of enterokinase for trypsinogen activation, there are two other key protective mechanisms that prevent pathologic, aberrant, premature activation. First, trypsinogen is tightly packed within the ZGs of the acinar cells, which shields it from the action of lysosomal proteases. Secondly, the acinar cell also secretes endogenous trypsin inhibitors, notably pancreatic secretory trypsin inhibitor (PSTI, also known as SPINK1), which block aberrantly active trypsin.
Lipases
Triglycerides are the predominant form of dietary lipids. They are de-esterified into fatty acids and monoacylglycerol before absorption by the intestine. Salivary and gastric lipases begin the process and cleave 15% to 20% of the triglycerides. However, the pancreatic lipases are responsible for the bulk of lipolysis during digestion. The three main lipases secreted by the pancreas are pancreatic triglyceride lipase (PTL), carboxyesterlipase, and phospholipase A2.
PTL is the predominant enzyme responsible for triglyceride hydrolysis at the sn-1 and sn-3 positions, yielding (from each triglyceride molecule) two fatty acids and one monoacylglycerol at the 2 position of glycerol. Another protein secreted by the pancreas, colipase, is required for the full activity of PTL. In concert with bile acids, which emulsify triglyceride droplets into smaller particles, colipase forms a 1 : 1 complex with PTL to greatly increase the surface area on which PTL can act. The pancreas synthesizes two other proteins with a high degree of sequence and structural homology to PTL, termed pancreatic lipase related proteins 1 and 2 (PLPR1 and 2). The function of PLPR1 remains undetermined. Human PLPR2 is a galactolipase, which plays a role in the digestion of plant lipids, as well as retinyl palmitate. Carboxylesterlipase has a wide spectrum of substrate specificity, including cholesterol esters, fat-soluble vitamin esters, tri-, di-, and monoacylglycerides, ceramides, and phospholipids. Phospholipase A2 hydrolyzes phospholipids such as phosphatidylcholine at its sn-2 position to lysophosphatidyl choline and a fatty acid.
Amylases
The salivary glands and the pancreas secrete α-amylase. It digests dietary starch from plants and glycogen from animal sources. The major dietary starches are amylose, a straight-chained α-1,4-linked glucose polymer, and amylopectin, which has α-1,4-glucose linkages and α-1,6 linked branches. α-Amylase hydrolyzes α-1,4-glucose linkages but not α-1,6 linkages or terminal glucose residues of starch and glycogen. The products of amylase digestion are short-chain α-1,6-linked polysaccharides, termed α-limit dextrins and are composed of maltose (an α-1,4-linked glucose dimer), maltotriose (a trimer of α-1,4-linked glucose molecules), and branched oligosaccharides with α-1,4 and α-1,6 linkages. Sucrase, glycoamylase, and isomaltase complete the digestion of dextrins into glucose at the intestinal brush border.
Regulation of Pancreatic Secretion
Pancreatic secretion is differentially regulated during various digestive phases by a complex interplay of gastrointestinal hormones and neuronal pathways ( Box 80-1 ).
Stimulatory
Cholecystokinin (CCK)
Secretin
Vasoactive intestinal peptide (VIP)
Gastrin-releasing peptide (GRP)
Insulin
Gastrin
Nitric oxide
Serotonin
Substance P
Pancreatic phospholipase A 2
Natriuretic peptides (ANP, CNP)
Melatonin
Adenosine
Inhibitory
Somatostatin
Pancreatic polypeptide (PP)
Peptide YY
Neuropeptide Y
Calcitonin gene–related peptide (CGRP)
Glucagon
Serotonin
Enkephalins
Leptin
Ghrelin
Interdigestive Phase of Pancreatic Secretion
The interdigestive phase is cyclic and closely follows the pattern of the migrating myoelectric complex (MMC) in the intestine. Phase I of the MMC is characterized by a lack of motility, with phases II and III possessing increasingly more activity. During phase I, pancreatic secretion of enzymes and bicarbonate is at its nadir (about 10% and 2% of maximum, respectively). Every 60 to 120 minutes, there is a surge in intestinal motility associated with phases II and III of the MMC in the duodenum and a progressive increase in gastric acid, bile, and pancreatic secretion. It is thought that this cyclic secretion of pancreatic juice is important in the digestion of residual food, cellular debris, and pathogens in the duodenum during the interdigestive period. The gastrointestinal hormones motilin and pancreatic polypeptide, as well as the autonomic nervous system, are involved in the regulation of MMC cycling. Administration of motilin prematurely activates the MMC and shortens the frequency between peaks, whereas pancreatic polypeptide inhibits the process. MMC blockade also occurs after administration of atropine, which implicates a role for vagal cholinergic stimulation during the interdigestive period.
Postprandial Phase of Pancreatic Secretion
Exocrine pancreatic secretion begins almost immediately after ingestion of a meal and is associated with an increase in the plasma levels of several stimulatory and inhibitory pancreatic hormones as well as neural stimulation via the vagus nerve. Postprandial pancreatic secretion is divided into three phases: the cephalic, gastric, and intestinal phases, which contribute 20%, 10%, and 70% to this overall phase of secretion, respectively.
The cephalic phase is initiated by sight, smell, taste, or even the thought of food. These perceptions are accompanied by a rise in plasma gastrin and CCK, a minor rise in plasma secretin, and a concomitant rise in the inhibitory hormones pancreatic polypeptide and leptin. Sham feeding studies in which subjects smell, taste, and chew but do not swallow food suggest that direct vagal stimulation is the major stimulant during the cephalic phase. Sham feeding experiments that revealed an incomplete block of pancreatic secretion during atropine administration suggest that peptidergic neurons from the vagus nerve may also directly activate acinar cells by releasing stimulatory peptides such as vasoactive intestinal peptide (VIP) and gastrin-releasing peptide (GRP).
The gastric phase begins when food enters the stomach and increases the rate of pancreatic secretion of enzymes as the stomach distends. Stimulation of mechanoreceptors in the body of the stomach activates the vagovagal cholinergic reflex that leads to a low-volume, enzyme-rich secretion from the pancreas.
The largest and final phase of postprandial pancreatic secretion, the intestinal phase, begins when gastric juice and food contents, collectively called chyme, enter the duodenum. Among hormonal mediators, the major players in the intestinal phase are secretin and CCK. A vasovagal cholinergic neural input also contributes to secretion during this phase. Some of the hormones and the neural regulation are discussed in more detail below.
Secretin
Secretin is the most potent stimulant of pancreatic fluid and HCO 3 − from pancreatic duct cells. It is synthesized by S-type enteroendocrine cells in the proximal small intestine and is released in the presence of duodenal acidification, bile, and products of protein and fat digestion. The pH in the duodenum is the primary regulator of secretin release; the threshold for release of secretin is a pH of 4.5. The mechanism by which acid stimulates secretin release has not been fully elucidated. When secretin is given simultaneously with vagal stimulation, the response is also highly augmented, suggesting that there is a cholinergic influence on pancreatic secretion.
Cholecystokinin (CCK)
CCK is the most potent stimulus of pancreatic enzyme secretion from the pancreatic acinar cells, and it is primarily elevated in serum during the gastric and intestinal phases of digestion. It is secreted by the intestinal I cells in response to products of protein and fat digestion in the duodenum. Carbohydrate digestive products or intact proteins or fat have little effect on CCK release.
The CCK response to fatty acids differs based on chain length (C18 > C12 > C8), degree of saturation, concentration, and total amount of exposure in the gut. There is some thought that an enterocyte-secreted trypsin-sensitive peptide, CCK-releasing peptide (CCKRP), stimulates enterocytes to release CCK in response to exposure to digestive products. During fasting periods, CCKRP is inactive because it is digested by free trypsin in the small intestine. During the postprandial phase, a duodenal protein load binds trypsin, and much more CCKRP becomes free to stimulate CCK release from enterocytes. A second peptide, monitor peptide, is a promoter of CCK release with actions similar to those of CCKRP. Both proteins appear to be regulated by vagal input. In humans, CCK exerts its effect through extrapancreatic, cholinergic pathways via neural reflexes, rather than through a direct endocrine effect on acinar cells.
Serotonin
Serotonin (5-hydroxytryptamine; 5-HT) is found in intestinal enterochromaffin cells and exerts a role in postprandial pancreatic secretion. It is released in response to several stimuli such as duodenal acidification as well as vagal and mechanical stimulation. Serotonin stimulates the vagovagal reflex, leading to pancreatic secretion independent of CCK. Serotonin also appears to regulate acid-induced pancreatic secretion of fluid and HCO 3 − by effecting the release and action of secretin.
Other Stimulatory Factors
Although secretin, CCK, and serotonin are the predominant hormones involved in pancreatic secretion, additional factors include insulin, gastrin-releasing peptide (GRP), and nitric oxide. The physiologic significance of these hormones and factors is still unclear.
Insulin mostly potentiates the secretory response to CCK, secretin, and acetylcholine. GRP, also known as bombesin, is a polypeptide secreted from the intestinal tract that primarily stimulates pancreatic enzyme secretion and, to a lesser extent, HCO 3 − . It is unclear whether it exerts its effect by promoting the release of CCK from the small intestine, by direct activation of acinar GRP receptors, or via cholinergic pathways whereby GRP-containing nerves stimulate acetylcholine release from postganglionic cholinergic fibers.
Nitric oxide is produced in the epithelium, endothelium, and macrophages of the intestinal tract and is abundant in the ducts, neurons, and vascular endothelium of the pancreas. Nitric oxide inhibitors such as nitric oxide synthase inhibitor and N- (G)-nitro- l -arginine (L-NNA) are effective in reducing pancreatic secretion, suggesting an important role of nitric oxide in exocrine pancreatic function. Nitric oxide might inhibit pancreatic secretion by reducing vascular perfusion of the pancreas.
There has also been increased interest in the role of natriuretic peptides on pancreatic secretion. Although atrial natriuretic peptide (ANP) and C-type natriuretic peptide (CNP) are classically known for their role in blood pressure regulation, they also stimulate pancreatic fluid and protein secretion. Both are capable of modifying HCO 3 − and Cl − secretions in pancreatic fluid, and CNP is capable of stimulating amylase release. ANP and CNP bind to the natriuretic peptide receptor C on the pancreatic acinar cells, which activates phospholipase C and initiates the intracellular signaling pathway leading to secretion. CNP also exerts itself through vagovagal reflexes at the pancreatic acinus.
Although melatonin is known for its role in maintaining the circadian rhythm, its receptors are located on pancreatic exocrine and endocrine cells, and it is known to stimulate pancreatic enzyme release. Melatonin may play a role in maintaining intracellular Ca 2+ stores and act as an antioxidant in the pancreas.
Finally, adenosine also plays a role in secretion by stimulating the opening of Cl− channels. This is supported by the finding of A2 adenosine receptors in human duct cell lines.
Neural Regulation
For the most part, the parasympathetic nervous system innervates the pancreas through the vagus nerve, with the majority of its preganglionic fibers terminating on the pancreatic ganglia. As previously mentioned, cholinergic effects of the vagus nerve have a tremendous influence, from the cephalic phase through the postprandial phase of pancreatic secretion of fluid, electrolytes, and enzymes. It remains to be determined which neurotransmitters are involved in these processes. Adrenergic innervation of the pancreas occurs through postganglionic neurons whose cell bodies are in the celiac and superior mesenteric ganglia, and are distributed primarily among the pancreatic vasculature. Inhibition of fluid and HCO 3 − secretion is achieved by sympathetic stimulation and subsequent pancreatic vasoconstriction. Neurons in ganglia from the myenteric plexuses of the upper gastrointestinal tract also innervate the pancreas and have cholinergic and serotoninergic characteristics. Neurons containing VIP are the most common in the pancreatic peptidergic nervous system. VIP released from vagal neurons can stimulate both acinar cells to secrete enzymes, and ductal cells to secrete fluid and HCO 3 − . Other peptidergic neurotransmitters with possible roles in pancreatic secretion include substance P, neuropeptide Y, enkephalins, and calcitonin gene–related peptide. Similar to the enteric nervous system, the pancreas has its own intrinsic innervation whose role in pancreatic secretion has not been fully clarified.
Pancreatic Secretion Inhibitors
Much less is known about the mechanisms that inhibit pancreatic secretion, although there are several known peptides, hormones, and neural regulators involved in this process. Pancreatic exocrine secretion can be negatively regulated by the presence of activated pancreatic proteases in the gut lumen via hydrolysis of CCK and secretin-releasing factors, as well as other peptides in the pancreatic juice. However, there are other gut hormones and components of bile that can also additionally inhibit secretion. Most of the hormones and neurotransmitters involved do not act directly on pancreatic acinar cells, but instead modulate cholinergic influences on pancreatic secretion.
Somatostatin is a pancreatic hormone produced mainly in the gastric and duodenal mucosa as well as in the D cells of the islets of Langerhans. Its release is stimulated by cholinergic activation in response to exposure to fat and amino acids in the intestinal tract. Although somatostatin can act directly on pancreatic acinar cells, the prevailing belief is that it inhibits pancreatic secretion through a cholinergic pathway.
Pancreatic polypeptide (PP) is a peptide hormone, found in the islets of Langerhans and between the acinar cells, which inhibits pancreatic secretion of fluid, HCO 3 − , and enzymes. Plasma levels of PP increase upon sham or actual feeding, and with duodenal acidification. Although vagal cholinergic activation is the most powerful stimulant to PP secretion, exposure to CCK, secretin, VIP, gastrin, and GRP also results in its release. PP probably modulates cholinergic transmission to the pancreas by interfering with acetylcholine release by presynaptic neurons in the pancreas. The discovery of PP receptors within the central nervous system suggests a central target of PP in the negative feedback of pancreatic secretion.
Peptide YY is a small peptide that is predominantly released in response to fat in the distal ileum and colon; it significantly attenuates HCO 3 − and enzyme secretion by decreasing pancreatic responses to CCK and secretin. Peptide YY likely inhibits release of acetylcholine and mucosal CCK as its primary mechanism of action.
Glucagon inhibits pancreatic secretion stimulated by CCK, secretin, or both. Other hormones and peptides, such as pancreastatin, calcitonin gene–related peptide, and enkephalins, inhibit pancreatic secretion by modulation of cholinergic stimulation at central vagal sites. Ghrelin and leptin also appear to neurohormonally inhibit pancreatic secretion.
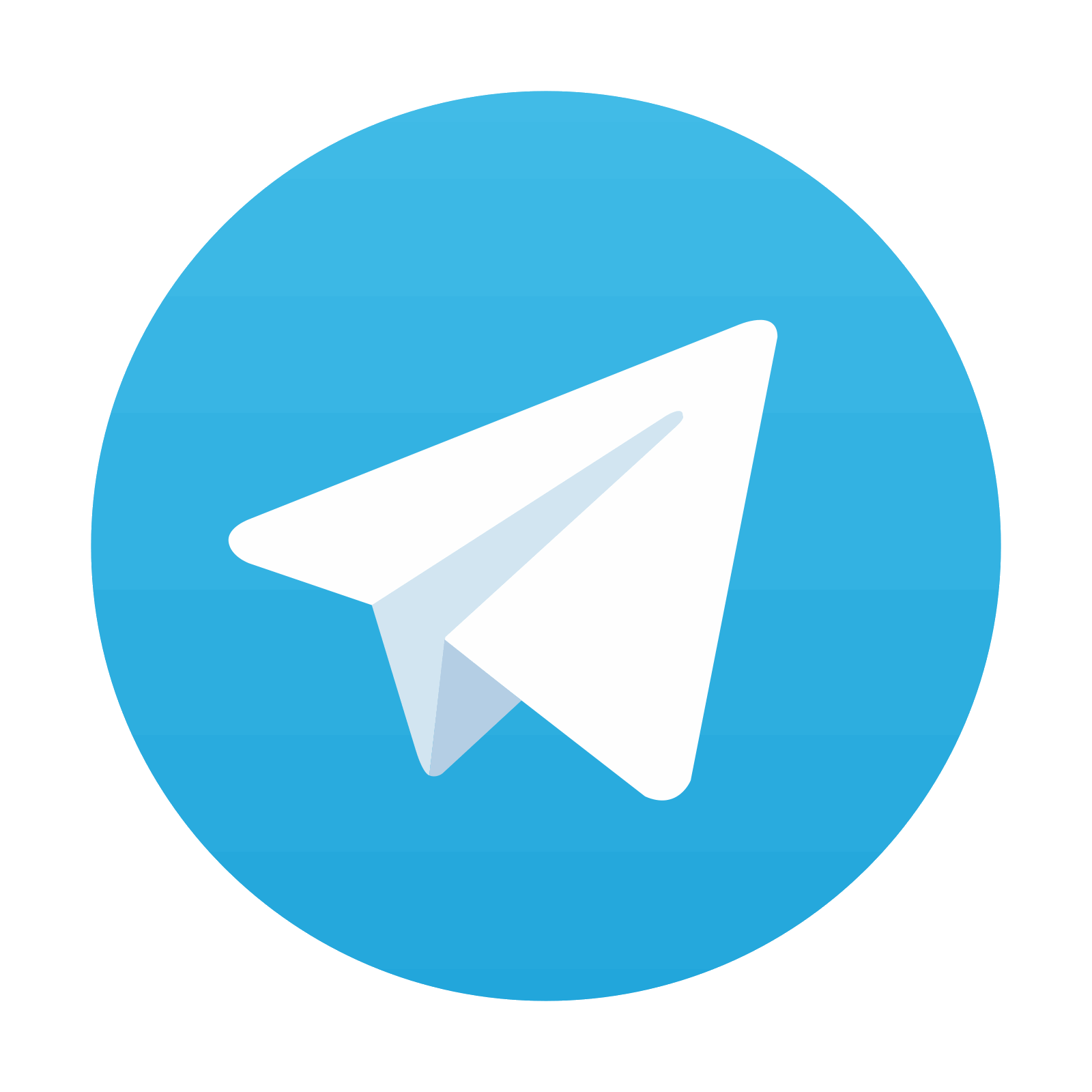
Stay updated, free articles. Join our Telegram channel
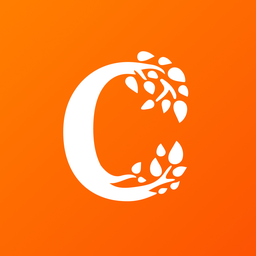
Full access? Get Clinical Tree
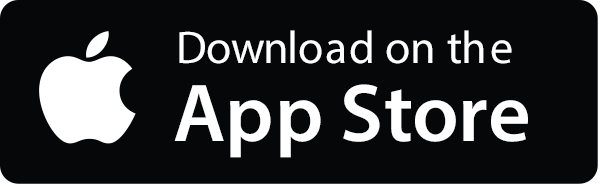
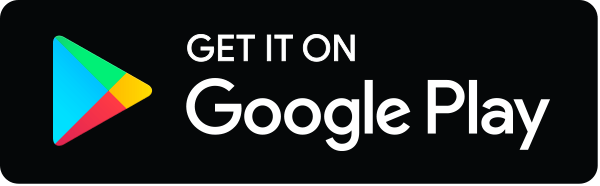