Three disorders are discussed in this chapter, including α1-antitrypsin deficiency, tyrosinemia, and urea-cycle enzyme defects.
α1-Antitrypsin Deficiency
The description of α1-antitrypsin deficiency and its association with lung disease was reported 40 years ago by Laurell and Eriksson. The association between α1-antitrypsin deficiency and hepatic cirrhosis in children was initially identified in 1969 by Sharp and coworkers. Since these original observations, it is clear now that α1-antitrypsin deficiency is a relatively common genetic disorder, affecting one in 1,600 to one in 2,000 live births, and resulting in liver disease in infants, children, and adults, as well as lung disease primarily in adults.
The Characteristics of the α1-Antitrypsin Protein
α1-Antitrypsin is a 52-kDa glycoprotein that is secreted by the hepatocytes, and, to a minor extent, by other tissues including lung and intestinal epithelial cells, neutrophils, and alveolar macrophages. The half-life of α1-antitrypsin is approximately 4 to 5 days. The function of α1-antitrypsin protein is to inhibit chymotrypsin, pancreatic elastase, skin collagenase, renin, urokinase, Hageman factor/cofactor, and the neutral proteases of neutrophils. α1-Antitrypsin protein belongs to a large gene family of serine protease inhibitors referred to as serpins.
α1-Antitrypsin is composed of 394 amino acids arranged into three β-sheets (A, β, and C), nine α-helices (A-I), and immobile inhibitory reactive center loop. The interaction between α1-antitrypsin and proteases occurs by the formation of a 1-1 complex. α1-Antitrypsin protein is present in tears, duodenal fluid, saliva, nasal secretions, cerebral spinal fluid, pulmonary secretions, and breast milk. α1-Antitrypsin acts as an acute phase reactant, and increases in the setting of inflammation, neoplastic disease, and pregnancy. However, in patients with α1-antitrypsin deficiency, these stimuli do not induce α1-antitrypsin protein.
Phenotyping of α1-Antitrypsin
The serum level of α1-antitrypsin in the plasma ranges from 100 to 200 mg/dL. This plasma level is determined by both α1-antitrypsin gene alleles, which are codominantly inherited. Several techniques including protein electrophoresis on starch gels and isoelectric focusing have contributed to our understanding of the variation in α1-antitrypsin. α 1 -Globulins appear in this system as a series of characteristic bands of variable intensity. The α1-antitrypsin variants included in an allelic system are called the Pi (protease inhibitor) system, and are named according to their migration velocity in the starch-gel electrophoresis. Faster moving protein complexes are identified by earlier letters in the alphabet, and the slowest moving protein is labeled Z. Thus, the variants of α1-antitrypsin are labeled as M (medium), S (slow), F (fast), or Z (very slow).
Three major categories of α1-antitrypsin variants have been identified as useful clinical markers:
- 1.
Normal, in this category are included the four more common M variants (M 1 to M 4 )
- 2.
Deficient, are characterized by the α1-antitrypsin variants Z and S, and a number of less-frequent variants, such as M Malton , M Procida
- 3.
Null, in which no detectable α1-antitrypsin level is seen
At least 100 different alleles of α1-antitrypsin have been described. The normal allele is PiM type with overall allelic frequency of 0.95. The next two most common alleles in the United States are PiS at 0.03 and PiZ at 0.01. Blacks have lower frequencies of these alleles. The highest prevalence of PiZ variant has been reported in Northern and Western European countries, peaking in Southern Scandinavia, Denmark, The Netherlands, the United Kingdom, and Northern France. Table 72-1 depicts the relationship between Pi phenotypes and serum concentration of α1-antitrypsin.
Phenotype | Serum Concentration (%) |
---|---|
MM | 100 |
MZ | 60 |
SS | 60 |
FZ | 60 |
M- | 50 |
PS | 40 |
SZ | 42.5 |
ZZ | 15 |
Z- | 10 |
— | 0 |
Genetics of α1-Antitrypsin Deficiency
The gene encoding α1-antitrypsin ( SERPINA 1) has been cloned, and is located on chromosome 14q31-32.2. The α1-antitrypsin gene is 12.2 kb in length, and consists of seven exons, designated I A , I B , I C , I (noncoding), and II, III, IV, and V (coding). Exons II to IV are translated into 52-kDa protein. The same gene is responsible for the α1-antitrypsin production in the liver, lung, and macrophages. The first two exons (I A , I B ) and a short 5′ segment of I C are included in the primary transcript in the macrophages, but not in hepatocytes. The protein has three asparagine-linked branched oligosaccharide moieties. The basis for the genetic defect in the PiZ type of α1-antitrypsin deficiency is the substitution of lysine for glutamic acid at position 342 from the carboxy terminus in the Z-type protein.
Several mutations within the SERPINA1 gene have been found to cause deficiency. The prevalence of the three major α1-antitrypsin variants (PiM, PiZ, and PiS) is reported as gene frequencies (the frequency of a variant in homozygotes). The highest prevalence of the PiZ variant has been recorded in European populations with a peak in Southern Scandinavia, Denmark, The Netherlands, the United Kingdom, and Northern France. Most recent surveys indicate that α1-antitrypsin deficiency is also prevalent in populations in the Middle East and North Africa, Central and Southern Africa, and Central and Southeast Asia. However, in Far East Asia, the gene frequency of α1-antitrypsin deficiency is rather rare, especially in Japan and Far Eastern populations.
Clinical Manifestations of α1-Antitrypsin Deficiency
Liver Disease in Children
Neonatal cholestasis is the first manifestation of α1-antitrypsin deficiency, and is commonly seen in the first few weeks of life. The affected infants are generally small for gestational age, and the liver is mildly enlarged. Acholic stools and dark urine may be seen. These patients appear to have elevated conjugated bilirubin, and mildly elevated serum amino transferase levels. Alkaline phosphatase and γ-glutamyl transpeptidase levels are also elevated. The jaundice usually disappears during the second to the fourth months of life. Neonates may present with liver cirrhosis or bleeding diathesis secondary to vitamin K deficiency.
The histologic picture of the liver may be helpful in predicting the outcome of the liver disease. A picture similar to that of neonatal hepatitis, portal fibrosis with bile duct proliferation, or intrahepatic duct hypoplasia may be noted in the biopsies. Patients with portal fibrosis and bile duct proliferation appear to have worse outcomes. However, the hallmark of the liver biopsy in these patients is the deposition of the PAS (periodic acid–Schiff) stain diastase resistant α1-antitrypsin depositions in the periportal hepatocytes.
The number of infants presenting with neonatal cholestasis was addressed in a large prospective study of 202,000 Swedish newborns in which 120 PiZ patients were identified. Fourteen of the 120 PiZ infants had prolonged obstructive jaundice, and nine had severe clinical laboratory evidence of liver disease. Five patients had only laboratory evidence of liver disease. Eight other PiZ infants had minimal abnormalities in serum bilirubin and hepatic enzyme activity, and variable hepatosplenomegaly. Approximately 50% of the remaining patients with PiZ had abnormal levels of aminotransferase only. Follow-up studies of these patients at 18 years of age showed that more than 85% had persistently normal serum transaminase levels. Forty-eight patients with the phenotype PiSZ were identified in this study. None of these infants had clinical liver disease, but 10 of 42 patients at 3 months and one of 22 at 6 months of age had abnormal liver function tests.
In the United States, screening of 107,038 newborns showed the Piz phenotype in 21 infants. Of the 18 infants followed, only one had neonatal cholestatic jaundice and 5 had hepatomegaly and biochemical abnormalities, or both. At 3 to 6 years of age, none of the children had evidence of hepatic cirrhosis. Other reports indicate that patients with PiZ who present with neonatal cholestasis are more likely to develop serious liver disease in the future compared to those infants without a history of neonatal cholestatic jaundice. The overall risk of death from liver disease in children with PiZ during childhood is estimated at 2% to 3%. Boys are at higher risk compared to girls. Why some patients with PiZ have worse liver disease than others is not known. However, genetic and/or environmental factors may play a role.
Liver Disease in Adults
Single case reports and retrospective studies have suggested that adult patients with PiZ are likely to develop liver disease and hepatocellular carcinoma. Therefore, it does appear that α1-antitrypsin deficiency should be considered in the differential diagnosis of any adult patient with abnormal liver function tests, liver cirrhosis, portal hypertension, or hepatocellular carcinoma. It is estimated that the risk of developing hepatic cirrhosis in adults with α1-antitrypsin deficiency is about 10%.
A retrospective study on 17 autopsied cases of α1-antitrypsin deficiency identified in Sweden indicated a strong relation between α1-antitrypsin deficiency, liver cirrhosis, and primary liver cancer. However, the study suggests that male patients are at higher risk of development of liver cirrhosis and hepatoma in α1-antitrypsin deficiency.
The relationship between liver cirrhosis and partial deficiency or heterozygotic phenotype of α1-antitrypsin has not been addressed on a larger scale in the literature. A number of case reports indicate the association of adult-onset liver cirrhosis with PiSZ. In one study, there was an increased prevalence of phenotype MZ in patients with cryptogenic liver cirrhosis and with non-B chronic hepatitis. However, in another prospective study, the heterozygote state occurred with approximately equal frequencies in patients with and without hepatobiliary disease.
A subset (approximately 3% to 5%) of patients with cystic fibrosis (CF) develop severe liver disease with portal hypertension. It has been reported recently that the α1-antitrypsin deficiency ( SERPINA1 ) Z allele is a risk factor for liver disease in CF. Patients who carry the Z allele are at greater risk (odds ratio ~5) of developing severe liver disease with portal hypertension.
In general, there is a suggestion that a partial deficiency of α1-antitrypsin is more likely to predispose these patients to liver injury. What is clear is that patients with liver cirrhosis and α1-antitrypsin deficiency are at risk of developing hepatocellular carcinoma. Indeed, Berg and Eriksson found six hepatomas in the nine cirrhotic adults who were phenotypically PiZ patients. Four of these tumors were hepatocellular carcinoma and two were cholangiocarcinoma.
Liver disease has also been associated with several other allelic variants of α1-antitrypsin deficiency, such as PiM Malton , Pi FZ , Pi W , PiM Duarte , and PiS iiyama .
Lung Disease
The development of lung disease in the pediatric patient is exceedingly rare, despite several reports that suggest that these patients do have increased respiratory infections. However, it is clear that adults with PiZ who are smokers will most likely develop emphysema. Autopsy studies indicate that approximately 60% of patients with PiZ develop clinically significant lung injury.
Pathophysiology of Liver Disease in α1-Antitrypsin Deficiency
The mutation in the PiZ α1-antitrypsin leads to the deposition of globules of an amorphous material within the hepatocyte, particularly in the periportal areas. These globules, which have been shown to enlarge as the infant matures, are seen by positive PAS staining after treatment of the liver biopsy specimen with diastase. Formed secondary to the accumulation of the mutated α1-antitrypsin molecule, these globules occur in the endoplasmic reticulum. Studies have suggested that the proper folding or assembly of the polypeptides is a prerequisite for their exit from the endoplasmic reticulum. Misfolding of the α1-antitrypsin variants allows the protein to be retained. The substitution of glutamine 342 for lysine in the α1-antitrypsin Z variant results in reducing the stability of the molecule in its monomeric form to a polymeric form by way of a mechanism termed loop-sheet insertion.
The mutation in the PiZ α1-antitrypsin is located at the head of the strand 5A and at the base of the mobile reactive loop. It seems that the mutation will open the β-sheet A between strands 3 and 5 allowing the incorporation of the reactive loop from a second α1-antitrypsin molecule to produce the dimer. The demonstration of spontaneous polymerization of the PiZ α1-antitrypsin at 37 degrees, whereas the normal M variant remains in its native confirmation, supported the observations by Lomas group. Thus, within the hepatocyte, the PiZ α1-antitrypsin is degraded by both proteasome-dependent and proteasome-independent pathways, and it appears that the clearing mechanism in those patients is inefficient.
Indeed, this polymerization of the α1-antitrypsin is not unique, as it does occur in other members of the serpin family, such as antithrombin, C1 inhibitor, and α 1 -antichymotrypsin, to cause deficiency in the plasma level of these molecules, resulting in thrombosis, angioedema, and emphysema, respectively. These serpin family disorders have been called conformational diseases.
Not all patients with α1-antitrypsin deficiency will develop liver disease. Therefore, other factors must be involved in the pathogenesis of the disease including increased production or decreased degradation. A possible mechanism is the serpin-enzyme complex, which is activated by α1-antitrypsin–elastase complex or other inflammatory mediators. Defects in hepatic proteasome action or other mechanisms involved in removing abnormal protein from the endoplasmic reticulum may lead to excessive accumulation of abnormal α1-antitrypsin in the hepatocytes. A possible mechanism is that susceptible individuals may have delayed intracellular degradation of the mutant protein, resulting from abnormalities in calnexin, a protein that interacts with the mutant α1-antitrypsin protein in the endoplasmic reticulum. Mannosidase 1, another endoplasmic reticulum protein, may have a role similar to that of calnexin in directing mutant α1-antitrypsin to the proteasome for degradation. Yet another important pathway to remove accumulated α1-antitrypsin polymers relates to autophagy. Indeed, rapamycin and carbamazepine have been shown to be effective in animal models of α1-antitrypsin Z. New therapeutic modalities are aimed at targeting these mechanisms such as the administration of chemical chaperones.
Although liver injury results from retained α1-antitrypsin glycoprotein in the endoplasmic reticulum, the mechanism of lung injury is different. Lung damage occurs as a result of uninhibited proteolytic changes of the connective tissue secondary to low concentration of circulating α1-antitrypsin.
Pathology of Liver Disease in α1-Antitrypsin Deficiency
The hallmark of α1-antitrypsin deficiency is the distinctive accumulation of PAS-positive, diastase-resistant globules in the endoplasmic reticulum in the periportal hepatocytes. These globules enlarge with increasing age. With the hematoxylin and eosin staining, they appear as eosinophilic deposits in the cytoplasm of the hepatocytes. These globules have also been observed in heterozygous individuals.
The absence of liver disease in the few Pi null individuals who have no deposits of these globules indicates that the deposition of the abnormal α1-antitrypsin in the liver has a significant role in the pathogenesis of liver disease. Electronmicroscopy studies show that these amorphous deposits are primarily within the dilated rough endoplasmic reticulum. Liver biopsies in neonates with α1-antitrypsin deficiency have shown three morphologic patterns of hepatic alteration, including hepatocellular damage with a picture compatible with neonatal hepatitis portal fibrosis with biliary duct proliferation and biliary duct hypoplasia.
Diagnosis of α1-Antitrypsin Deficiency
Low serum level of α1-antitrypsin does not indicate the presence of α1-antitrypsin deficiency, since this level could be low secondary to losses of α1-antitrypsin in the gastrointestinal tract or in the lung. Therefore, determination of the α1-antitrypsin phenotype by isoelectric focusing or by agarose electrophoresis at acid pH is indicated. α1-Antitrypsin deficiency should be suspected in neonates with a picture of neonatal hepatitis or in children and adults with unexplained chronic liver disease. Liver biopsies should be stained with PAS following diastase treatment to determine the presence of α1-antitrypsin globules in the periportal hepatocytes.
Treatment of α1-Antitrypsin Deficiency
The two major clinical problems in α1-antitrypsin deficiency are emphysema and liver disease resulting in cirrhosis. Therefore, avoidance of smoking is the most important preventive step in the development of emphysema, because smoking accelerates the destructive lung disease. Once the patient develops cirrhosis, liver transplantation is the only viable option for these patients. Replacement therapy of α1-antitrypsin deficiency has been done in emphysema patients, and found to be effective in raising the concentration of α1-antitrypsin in the serum and in the lung. The α1-antitrypsin in the lung has been shown to be active in neutralizing the neutrophil elastases. A recombinant α1-antitrypsin has been produced in Escherichia coli and in yeast, and was noted to be functional as an elastase inhibitor. However, because the recombinant α1-antitrypsin lacks the carbohydrate side chain, this product is unstable with a short half-life.
Efforts to induce α1-antitrypsin using danazol (isoxazole derivative of 17-ethinyl testosterone) have been shown to increase serum levels of α1-antitrypsin in only about 50% of deficient patients. However, the potential hepatotoxicity has hampered the efforts to utilize danazol as a potential therapeutic agent. Moreover, although increasing the level of α1-antitrypsin in the serum has an effect on lung disease, it has no effect on liver disease. Novel therapies to inhibit the polymerization of the α1-antitrypsin molecule or enhance its secretion and gene therapy utilizing adeno-associated virus as vector are potential therapeutic targets.
Concordant results of prospective cohort studies suggest that augmentation therapy has efficacy in slowing the rate of decline of lung function in patients with moderate airflow obstruction and severe deficiency of α1-antitrypsin. Although augmentation therapy is well tolerated, it is not cost-effective. New approaches have been aimed at blocking intracellular polymerization and increasing degradation utilizing drugs such as rapamycin and carbamazepine, which increase autophagic process. Stem cell technology offers promise for the future.
Few patients with metabolic defects of liver function have been treated with hepatocyte transplantation including patients with urea-cycle disorder, ornithine transcarbamoylase deficiency, Crigler-Najjar syndrome, and α1-antitrypsin deficiency. Hepatocyte transplantation is still considered an experimental procedure but may prove to be an effective therapeutic modality for the treatment of patients with metabolic liver disease or as a bridge for those waiting for liver transplantation.
Tyrosinemia
Several genetic and acquired hepatic disorders can result in impaired degradation of the aromatic amino acid tyrosine ( Box 72-1 ). The degradation of tyrosine ( Figure 72-1 ) is catalyzed by a series of five enzymatic reactions yielding acetoacetate as well as the Krebs cycle intermediate fumarate. The complete pathway is expressed in only two cells: the hepatocytes and renal proximal tubules, which contain enough quantities of the five enzymes required for tyrosine metabolism.
- 1.
Transient tyrosinemia of the newborn
- 2.
Hepatocellular dysfunction
- 3.
Enzyme deficiencies in tyrosine metabolism
Hepatorenal tyrosinemia (HTI)
Oculocutaneous tyrosinemia (HT2)
4-OH-Phenylpyruvate dioxygenase deficiency (HT3)
- 4.
Hyperthyroidism
- 5.
Scurvy

The most common cause of hypertyrosinemia is transient tyrosinemia of the newborn. The condition results from immaturity of the liver and the enzymes involved in the degradation process. Transient tyrosinemia of the newborn, which used to be a common finding, is now a much more rare condition due to advanced neonatal management. Hypertyrosinemia and increased urinary excretion of tyrosine metabolites can result from hepatic dysfunction of any cause.
Although autosomal-recessive enzyme deficiencies have been reported for four of the five degradation reactions, elevated blood tyrosine level is not seen in all of these conditions and liver disease is seen in only one of these disorders: hereditary tyrosinemia type I.
Hepatorenal Tyrosinemia (Hereditary Tyrosinemia Type I)
Hepatorenal tyrosinemia, which is also referred to as hereditary tyrosinemia type I (HTI), was first reported by Sakai and Kitawaga in 1957 in a patient who developed progressive liver disease complicated by bleeding and coma, and resulting in death at the age of 3 years. The patient also had rickets, which was resistant to vitamin D supplementation. In a report of seven patients, Gentz et al. described the renal component of the disorder. Neurologic crises similar to porphyria led to the recognition that δ-aminolevulinic (δ-ALA) acid was excreted in large amounts. Succinylacetone (SA) found in the urine of patients with tyrosinemia is an inhibitor of the synthesis of porphobilinogen from δ-ALA.
Pathogenesis
The pathway for tyrosine metabolism is shown in Figure 72-1 . Fumarylacetoacetate hydrolase (FAH), the last enzyme in the pathway, was identified by Lindblad et al. as the defect leading to tyrosinemia. FAH deficiency leads to the accumulation of fumarylacetoacetate and maleylacetoacetate and their derivatives succinylacetoacetone (SAA) and SA. Because fumarylacetoacetate and maleylacetoacetate usually initiate hepatocyte injury, maleylacetoacetate is more likely to lead to renal tubular dysfunction. SA is an inhibitor of the condensation of δ-ALA to porphobilinogen in heme synthesis, leading to accumulation of δ-ALA, which is neurotoxic.
Clinical Features
Acute and chronic forms of tyrosinemia are recognized, and both forms can be seen in the same family. Patients with the acute form present with hepatic dysfunction early in life, which is usually fatal during the first 2 years. Symptoms may include vomiting, diarrhea, hepatosplenomegaly, edema, ascites, bleeding diathesis, irritability, and jaundice. In a multicenter study, van Spronsen et al. showed that 25% of the patients presented during the first 2 years and that their 1- and 2-year survival rate was 38% and 29%, respectively. The earlier the onset, the worse the prognosis. The usual causes of death (35 of 47 deaths) were recurrent bleeding and liver failure. Hepatocellular carcinoma and neurologic crisis were also major factors. On the other hand, the chronic form may be a continuation of the acute presentation or may start later during infancy or childhood with manifestations of chronic liver disease and renal tubular defects.
Neurologic crises of pain and paresthesia are a result of peripheral neuropathy, and are usually seen in patients presenting with the chronic form of the disease. The crises, which are usually precipitated by an infection, can be mistaken for porphyria. Symptoms may also include hypertonia, vomiting, and weakness. Paralysis may develop and progress to complete flaccid paraplegia requiring mechanical ventilation. The onset of neurologic crisis is believed to be the result of the accumulation of SA blocking further metabolism of δ-ALA and is not necessarily associated with deterioration of hepatic function.
Rarely, patients may present with nonhepatic manifestations such as renal dysfunction, porphyria-like illness, or cardiomyopathy. Hepatocellular carcinoma (HCC) can be seen in up to 37% of patients. The youngest reported patient was 33 months of age. Detection of nodules with ultrasound and computed tomography (CT) scan appears to be reliable as demonstrated by histologic examination of liver biopsies of patients who underwent liver transplantation; carcinoma was not found in those in whom nodules were not detected. A significant increase in α-fetoprotein may herald the development of HCC. However, cancer can be seen in the face of normal levels. Patients should be monitored by ultrasound, MRI or CT scan, and α-fetoprotein on a regular basis.
Diagnosis
The diagnosis of tyrosinemia should be considered in any infant with neonatal liver disease and, later in life, in any child with undiagnosed liver disease or rickets. It is important that the diagnosis be made promptly in order to initiate medical treatment and prevent the evolution of hepatic fibrosis and cirrhosis. Patients with acute tyrosinemia often have evidence of impairment of hepatic synthetic function disproportionate to biochemical indices of liver injury. This is evidenced by hypoalbuminemia and marked coagulopathy, with only a mild-to-moderate rise in aminotransferase values. Hemolytic anemia and hypoglycemia may be present with a variable rise in total and direct bilirubin. The renal tubular dysfunction consists of Fanconi’s syndrome with hyperphosphaturia, glucosuria, proteinuria, and aminoaciduria. Marked elevation of serum tyrosine is usually seen at levels higher than those seen in other liver disorders. Other features include hypermethioninemia, urinary excretion of phenolic acid by-products of tyrosine ( p -hydroxyphenyl lactic acid, p -hydroxyphenyl pyruvic acid, and p -hydroxyphenyl acetic acid), which can be screened for with nitrosonaphthol; succinylacetone and succinyl acetoacetate in urine; and increased urinary excretion of δ-ALA. Patients with the acute disease may have generalized aminoacidemia with a disproportionate elevation of serum levels of tyrosine and methionine, whereas later in life, aminoacidemia is usually limited to tyrosine, but aminoacidemia is generalized, with tyrosine predominating.
The presence of greatly increased amounts of α-fetoprotein in the presence of normal levels of tyrosine in cord blood of affected infants, suggests that hypertyrosinemia may develop. In the acute form, there is fatty infiltration of the liver; iron deposition; varying degrees of liver cell necrosis, which may be extreme; and fine diffuse fibrosis with formation of pseudoacini. Older children may exhibit gross multilobular cirrhosis, regenerative nodules, and bile duct proliferation.
Genetics and Prenatal Diagnosis
HTI is an uncommon inborn error of metabolism. The incidence of the disease is 1:100,000 to 1:120,000. However, the incidence is much greater in certain areas such as Northern Europe and Quebec, Canada. The frequency of heterozygotes in the population of the Lac-St. Jean region in Quebec has been estimated to be about 7%.
The human FAH cDNA has been cloned and mapped to chromosome 15. The common mutations responsible for the clustering of cases in certain areas of the world have been identified. The IVS12+5G>A → a splice mutation, which is found in most cases of acute tyrosinemia in the HTI French Canadian cluster, also occurs in the chronic phenotype. Thus, that both clinical forms of HTI can result from the same mutation and genetic heterogeneity is not a sufficient explanation for the clinical heterogeneity of the disorder.
Prenatal diagnosis can be made by screening amniotic fluid for succinylacetone. However, false-negative results have been reported. FAH can be also screened in amniotic cells. In addition, diagnosis can be made by direct mutation detection and linkage analysis, taking advantage of the presence of several intragenic polymorphic markers. Newborn screening is undertaken in areas with high prevalence of the disorder such as the Canadian province of Quebec.
A mosaic pattern of immunoreactive FAH protein found in livers removed from patients with HTI for transplantation was reported by Kvittingen et al. Analysis for the tyrosinemia-causing mutations revealed that the immunonegative liver tissue contained the FAH mutations demonstrated in fibroblasts of the patients, while in the immunopositive nodules of regenerating liver tissue, mutation reversion was seen. The genetic mosaicism may explain the variability in the clinical manifestations of the disease, even within the same family. It is possible that patients with a larger mass of reverted hepatocytes will have the mild form of the disease. However, this remains to be further defined.
Treatment
Dietary restriction of phenylalanine and tyrosine has been the traditional treatment for patients with tyrosinemia. Dietary management has been shown to be effective for renal tubular disease and the metabolic bone disease. However, there is no evidence that it prevents the development of cirrhosis or HCC.
Nitisinone (NTBC)—2-(2-nitro-4-trifluoromethylbenzoyl)-1.3-cyclohexamedione—belongs to a class of compounds developed in the 1980s as bleaching herbicidal. During animal studies, it was noted that rats treated with NTBC developed corneal ulceration, a hallmark of elevated tyrosine level in rats and humans. It was then discovered that NTBC is an inhibitor of 4-hydroxyphenylpyruvate dioxygenase (HPD). In 1992, Lindstedt et al. proposed that the inhibition of HPD by NTBC can prevent the accumulation of maleylacetoacetate and fumarylacetoacetate and their derivatives.
Recently, the results of treating more than 300 patients with NTBC were reviewed. Marked hepatic improvement was seen in more than 95% of the patients. Renal function also improved and Fanconi syndrome was avoided. A large number of patients have been taking the drug for more than 5 years (up to 9 years) without significant side effects. NTBC appears to be safe during pregnancy. Typically, NTBC is started at an oral dose of 1 mg/kg per day, and the dose can be adjusted depending on clinical response and biochemical parameters. It is important that patients taking NTBC remain on a protein-restricted diet that is low in tyrosine and phenylalanine, since NTBC will increase the blood tyrosine level. Biochemical monitoring of plasma amino acids, blood, and urinary succinylacetone and liver functions is mandatory during therapy with NTBC. Recurrent ophthalmologic evaluation and hepatic imaging are also important. Measurement of α-fetoprotein is particularly important, since few late-treated patients developed HCC despite NTBC therapy. Unfortunately, impaired cognitive functioning has been reported in patients with tyrosemia type I who are receiving nitisinone. Further follow-up is needed to assess the long-term effects and safety of NTBC therapy, taking into consideration that some patients developed cancer despite therapy and that patients with HPD deficiency develop neurologic problems, and mental retardation is seen in 50% of patients with tyrosinemia type II.
The mainstay treatment for patients with tyrosinemia with end-stage liver disease is orthotopic liver transplantation (OLT), normalizing FAH activity, and correcting the metabolic derangement and hepatic function. OLT liver transplantation is an effective treatment for TTI with good quality of life. The current indications of OLT in TTI are nonresponse to NTBC, risk of malignancy, and poor quality of life related to dietary restriction and frequency of blood sampling. OLT has a marked beneficial effect on the renal function with improvement in the tubular dysfunction. However, patients with severe renal disease prior to OLT may continue to have borderline renal function and poor growth even after transplantation.
Urea-cycle Defects
Catabolism of amino acids produces ammonia, a potent neurotoxin unless inactivated by the liver. Animal studies have shown that hyperammonemia is toxic to the immature nervous system, producing alterations in the level of consciousness. The neuropathologic changes involve the astrocytes and not the neurons, which suggests that the changes may be reversible. However, repeated prolonged episodes of hyperammonemia can lead to permanent neurologic impairment.
Detoxification of ammonia occurs through a series of reactions known as the Krebs-Henseleit or urea cycle ( Figure 72-2 ). In addition to converting ammonia into urea, the cycle produces arginine, which becomes an essential amino acid in all urea-cycle defects except arginase deficiency. Five enzymes are involved in the formation of urea: carbamyl phosphate synthetase (CPS), ornithine transcarbamylase transferase (OTC), argininosuccinate synthetase (AS), argininosuccinate lyase (AL), and arginase. In addition, a sixth enzyme, N -acetylglutamate synthetase, is also needed for the formation of N -acetylglutamate. Four enzymes operate in a cyclic manner using ornithine as a substrate, which is regenerated. On the other hand, CPS, which is produced by a series of enzymatic reactions, enters the cycle by combining with ornithine. The other nitrogen atom of urea is derived from aspartate, which combines with citrulline.

Clinical Features
Symptoms may start early in life or be delayed until late childhood or adulthood. Newborns may have rapid clinical deterioration resembling sepsis, usually after a few days of protein feedings. Symptoms include refusal to eat, vomiting, tachypnea, seizure, and lethargy progressing to coma. Increased intracranial pressure may be evident. Later in life, hyperammonemia may present with vomiting and multiple neurologic abnormalities including irritability, mental confusion, ataxia, and combativeness alternating with periods of drowsiness and coma. Delayed physical growth as well as development may be seen and the patients may elect to consume a low-protein diet to avoid the symptoms.
Diagnosis
Hyperammonemia is the characteristic feature of urea-cycle defect. The degree of elevation in plasma ammonia varies according to the severity of the disorder and protein intake. Values up to 20 to 30 times normal may be seen during the neonatal period. Patients with late onset may have plasma ammonia levels around twice the normal values. A direct relation exists between the duration of the hyperammonemia and subsequent intellectual ability of the child. However, there is no relation between the severity of hyperammonemia and later intelligence or neurologic development.
Patients with CPS and OTC deficiencies do have specific abnormalities of plasma amino acids in addition to elevated alanine, glutamine, and aspartic acid secondary to hyperammonemia. Plasma citrulline level may serve as an initial screening tool in patients with urea-cycle defects. Plasma citrulline, which is very low in OTC and CPS deficiencies, exceeds 1,000 µmol/L in AS deficiency (citrullinemia) and is usually in the range of 100-300 µmol/L in AL deficiency. Urinary orotic acid level may help to differentiate CPS and N -acetylglutamate synthetase (NAGS) deficiencies, which are characterized by low orotic acid levels from OTC deficiency, which is associated with high urinary orotic acid levels.
Serum transaminase levels are elevated during the acute exacerbations and may remain high between episodes. Prothrombin time may be markedly prolonged. Respiratory alkalosis is transiently present in acute attacks. Hepatic histology is usually nonspecific and unremarkable. Histopathologic changes in livers from neonates with urea-cycle defects are nonspecific. Older patients with urea-cycle defects seem to show variable hepatic fibrosis compared to patients who died earlier in the course of the disease. Some of these patients also show features of glycogen storage disorder and cirrhosis characterized by slow progression.
Fibrosis has also been noted in patients with several disorders of the urea cycle. Heterozygote OTC deficiency in females may exhibit several histologic abnormalities including steatosis, focal necrosis, and portal fibrosis.
After the clinical condition stabilizes, the diagnosis should be confirmed by measurement of enzyme activity in an appropriate tissue ( Table 72-2 ). In addition to the liver, OTC is expressed in the intestinal mucosa and therefore the diagnosis can be made by enzyme assays in duodenal and rectal biopsies. However, the gold standard is measuring the enzyme activity in liver tissue in males with OTC deficiency, as the enzyme activity may be virtually absent. Males with partial variants may have enzyme activity that ranges from 5% to 25% of normal. In symptomatic heterozygous females, levels of activity range from 4% to 25% of normal. Heterozygous mothers of affected children may have levels as high as 97% of normal activity. It has to be noted that the in vitro measurement of the enzyme activity may not accurately reflect the in vivo activity of the enzyme.
Disorder | Tissue Diagnosis | Urine Orotic Acid |
---|---|---|
Carbamyl phosphate synthetase (CPS) deficiency | Liver | Normal |
Ornithine transcarbamylase (OTC) deficiency | Liver | Very High |
Argininosuccinate synthetase deficiency | Fibroblasts | High |
Argininosuccinate lyase deficiency | Erythrocytes | High |
Arginase deficiency | Erythrocytes | High |
N -Acetylglutamate synthetase (NAGS) deficiency | Liver | Low or Normal |
Differential Diagnosis
The differential diagnosis of hyperammonemia is summarized in Box 72-2 . In the neonatal period it is important to differentiate transient hyperammonemia (THA) of the newborn (THN) from inborn errors of metabolism, namely urea cycle defects and organic academies. Differentiation may be made using an algorithm suggested by Hudak and coworkers. Neonates with THA were found to have lower weights and earlier gestational ages. A rapid neurologic deterioration is seen in patients with THN compared to those with urea-cycle defects in whom coma is seldom seen before the third day of life. THA is an ill-understood disorder. It has been suggested that decreased hepatic flow secondary to ductus venosus shunting or transient platelet activation may contribute to hyperammonemia.
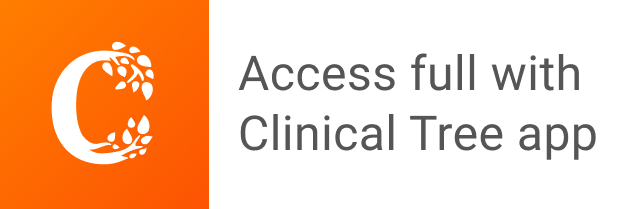