Schematic presentation for cellular events involved in the progression of chronic kidney disease
2.2 Glomerulosclerosis
Each normal glomerulus is a tuft of small blood vessels called capillaries situated within Bowman’s capsule with the kidney. The tufts are structurally supported by mesangial cells, which facilitate structural organization and integrity of capillaries of glomerulus. Endothelial cells lining capillaries uniquely have numerous pores also named fenestrated endothelial cells. The glomerular base membrane (GBM) separating fenestrated endothelial cells from podocytes is the basal lamina layer of the glomerulus. The podocyte foot processes, GBM and endothelial cells constitute the glomerular filtration barrier, a highly specialized blood filtration interface. Dysfunction of the glomerular filtration barrier and expansion or proliferation of mesangial cells are thought to contribute to the development of glomerulosclerosis.
Glomerulosclerosis is characterized by an increase in mesangial matrix accumulation and obliteration of glomerular capillaries. The underlying pathogenic mechanism of glomerulosclerosis is even more complicated and can be divided into several distinctive phases. First, under the influence of various risk factors such as hypertension, dyslipidemia, and/or deposition of immune complexes, glomerulus-resident cells are injured and activated, followed by a release of multiple cytokines and chemokines, which attract monocytes, lymphocytes, neutrophils, and other types of inflammatory cells to immigrate and accumulate at the injury site. Second, these infiltrating cells accompanied with intrinsic cells further produce multiple cytokines and growth factors including angiotensin, transforming growth factor β1 (TGF-β1), platelet-derived growth factor (PDGF), fibroblast growth factor (FGF), tumor necrosis factor (TNF), and interferon gamma (IFN-γ) to induce the capillary collapse and obliteration, loss of podocytes, and activation of parietal epithelium cells. The final process is fibrogenesis, featuring production of new ECM components to replace damaged tissue, thereby providing a scaffold for wound closure, remodeling, and repair. Thus, glomerulosclerosis mainly reflects podocyte injury, proliferation, and matrix production by mesangial cells as well as endothelial damage and dysfunction.
Podocyte injury is a key manifestation of proteinuric glomerulopathies, including focal segmental glomerulosclerosis (FSGS), minimal change disease (MCD), membranous-proliferative glomerulonephritis (MPGN), amyloid nephropathy (AN), and diabetic nephropathy (DN). Four major mechanisms cause injury to podocytes: alteration of the GBM or its interactions with the podocyte, molecular change in the slit diaphragm or interference with its structure, dysfunction of podocyte actin cytoskeleton, and changes in negative surface charge on podocytes. In response to insults, podocytes appear different adaptive changes, such as hypertrophy, transdifferentiation, dedifferentiation, detachment, and apoptosis. These adaptive responses of podocytes mainly depend on the insult severity and duration.
Mesangial cells constitute the central stalk of the glomerulus and represent continuation of the extraglomerular mesangium and the juxtaglomerular apparatus. Proliferation of mesangial cells is a prominent feature of glomerular disease including IgA nephropathy (IgAN), membranous-proliferative glomerulonephritis (MPNG) lupus nephritis (LN), and DN. Mesangial-cell proliferation and matrix accumulation promoted by many kinds of profibrotic factors including TGF-β, PDGF, and FGF2 are believed to contribute to the development of glomerulosclerosis. In response to initial injury factors, mesangial cells undergo transformation to mesangioblasts. The latter are capable of producing an excessive ECM, leading to mesangial expansion: an early sign of glomerulosclerosis. The relative contribution of podocytes, mesangial cells, and other resident glomerular cells to matrix accumulation and glomerulosclerosis may depend on the disease in question.
2.2.1 Podocyte Injury
The podocyte is one of the most important cell types for the glomerular filtration barrier. The podocyte and its slit diaphragm are the main structure of the glomerular filtration barrier. Podocytes stabilize the filtration barrier of glomerular through production of GBM molecules, maintenance of the structure of slit diaphragm, and keeping viability of endothelial cell. Podocytes injured by various risk factors undergo detachment or apoptosis, which is an irreversible loss that permanently causes inefficiency of podocytes. Because podocytes are terminally differentiated cells and can’t proliferate, the area of the GBM initially covered by podocyte becomes exposed. To the areas of the exposed GBM, parietal epithelial cells may get attached. This scenario leads to form adhesions or synechiae between GBM and parietal cells, which are recognized as some of the earliest signs of segmental glomerulosclerosis. Maladaptive interactions develop between the GBM and parietal epithelial cells. Extension of the synechiae leads to the leakage of protein into Bowman’s space, collapse and sclerosis of the associated capillary tuft, and the loss of endothelial cells, which is suggestive of the formation of crescents and sclerosis. Tracer studies have been performed to disclose that filtration of the tracer mainly accumulates extracellularly at the sites within tuft adhesions to Bowman’s capsule and related paraglomerular spaces. The accumulation of protein debris is obligatory for capillary collapse. More than that, proteinaceous fluid derived from misdirected filtration is trapped within the space between parietal epithelium and GBM, ultimately causing the progression to global sclerosis and obsolescence.
2.2.1.1 Slit-Diaphragm Destruction
The slit diaphragm is the main barrier that limits protein leakage. The slit-diaphragm system destruction can lead to foot process effacement (FPE). The slit diaphragm can be subdivided into two parts structurally. ZO-1, α-actinin-4, podocin, and CD2AP have been recognized as intracellular parts of the slit diaphragm; NEPH family members, Nephrin, FAT, and P-cadherin are known to constitute the extracellular components of the slit diaphragm. Proteinuria is an early consequence of podocyte injury and is a typical sign of kidney disease [4]. Podocyte-specific ablation of slit-diaphragm-related proteins in mice may result in severe proteinuria and sclerosis. Clinically, proteinuria is a strong independent risk factor of progressive CKD. Although podocyte injury directly results in proteinuria, it is possible that proteinuria further worsens podocyte injury. Electron-microscopic studies have shown that a large number of protein droplets in the podocyte may be related to proteinuria. After proteinuria develops, tubular cells also take in large amounts of albumin, which can cause interstitial inflammation and fibrosis.
In congenital nephrosis, it is known that neutralization of nephrin by a specific antibody and a podocyte-specific conditional knockout of a slit-diaphragm gene such as nephrin, CD2AP, or α-actinin-4 each results in severe proteinuria and sclerosis [5]. In response to injurious stimuli, podocytes undergo phenotypic alteration characterized by downregulation of slit-diaphragm-associated proteins including P-cadherin, ZO-1, and nephrin and their own molecular signatures such as Wilms tumor protein 1 (WT1), synaptopodin, and initiation of expression of mesenchymal markers, including desmin, fibroblast-specific protein 1 (FSP1), and matrix metallopeptidase 9 (MMP-9), producing interstitial matrix components, such as fibronectin and type I collagen. This phenotypic alteration also involves upregulation of the transcription factor Snail and integrin-linked kinase. Liu et al. have named this process “podocyte epithelial–mesenchymal transition (EMT),” which is similar to kidney tubular epithelial cells undergoing mesenchymal transition during kidney interstitial fibrosis [6, 7]. A number of important intracellular signal pathways, such as ILK, Wnt–β-catenin, Jagged-Notch, and Snail, often specially drive podocyte into “EMT” program in the pathogenesis of various proteinuric renal diseases. These data are suggestive of podocyte undergoing “EMT” program in response to various injuries. Eventually, impairment of podocyte filtration barrier is consequence for podocyte phenotype changes, leading to proteinuria and glomerulosclerosis.
Furthermore, slit diaphragm, the only structure participating in cell–cell junctions and communications, have important implications for stabilizing cellular polarity. Damaged podocytes lose their polarity, which causes proteins normally situated on the podocyte slit diaphragm to spread to abnormal locations. Arrangement of these proteins may interrupt the communications between podocytes and lead to disorganization of the architecture and to FPE shown in proteinuric renal diseases. The slit diaphragm may also have signal transduction functions. For example, tyrosine phosphorylation of nephrin correlates with the activation of signaling pathways in vivo and in vitro [8]. After nephrin phosphorylation, multiple downstream molecules perform critical functions to maintain podocyte polarity and regulate cell survival.
2.2.1.2 Changes in the Actin Cytoskeleton of Podocytes
It is known that podocytes function to support and maintain the structural of the glomerular tuft. Podocyte foot processes with highly dynamic performance partly depend on a rich actin cytoskeleton, which eventually establishes the podocyte’s shape. A study conducted by Ichimura et al. [9] have shown that using electron microscopy, two populations of actin cytoskeletons are included in matured podocytes. One is actin bundle running above the level of slit diaphragms and the other is the cortical actin network situated beneath the plasmalemma. FPE often has close relations with an increase in the number of microfilaments. Meanwhile, the podocyte actin system is regulated by the Rho family of guanosine triphosphatases, whose member Cdc42 mediates filopodia formation [10]. Mice with a podocyte-specific Cdc42 knockout have severe proteinuria and extensive nephrosclerosis associated with a reduction in the expression of slit-diaphragm components. Guanosine triphosphatase dynamin plays a basic role in regulating actin cytoskeleton of podocytes [11], and interference with oligomerization of actin-dependent dynamin with inhibitor effectively attenuates proteinuria and progress of renal diseases [12]. The actin cytoskeleton system is necessary not only for supporting foot processes but also for podocytes to confront the filtrate pressure. Thus, these findings suggest that protein molecules maintaining or regulating podocyte actin cytoskeleton system are extremely important to podocyte functions, and any changes in the actin or actin-related proteins may cause podocyte shape alterations and podocyte dysfunctions [13].
2.2.1.3 Loss of Podocytes
Podocyte Detachment
As mentioned above, podocyte detachment from the GBM resulting in the formation of adhesions or synechiae is the main pathophysiological mechanism of glomerulosclerosis. Podocytes are normally tethered to the GBM by integrins and dystroglycans. Integrins and dystroglycans expressed on the podocyte plasma membrane specifically bind to ligands in the GBM matrix to maintain tight cell attachment. Several mechanisms of podocyte detachment, including mechanical distension, shear forces, and/or impaired adhesion to the GBM, have been suggested. In response to mechanical forces, including intraglomerular hypertension, hyperfiltration, hypertrophy, and podocytes may undergo detachment from the GBM. Intraglomerular hypertension will contribute to (1) not only increased axial capillary wall and circumferential stress but also increased filtrate flow, causing (2) an increase in fluid shear stress on the podocytes. (3) In this setting of circumstances, podocytes are challenged by the hypertrophy of glomerular to obtain more motility and cover more areas of the GBM. (4) In the progressive phase of the disease, podocytes will be challenged by increased expansile forces and then undergo detachment.
Studies also show integrin outside-in signals regulate podocyte-GBM adhesion; inside-out signals from the podocyte cytoskeleton are also critical for podocyte adhesion to the GBM. Research suggests that there is decreased expression of integrin α3β1 on podocytes of humans and rats during diabetes; this phenomenon causes detachment of podocytes from the GBM [14]. Talin-1, a large cytoskeletal protein in the podocyte, favors inside-out signal transduction through the activating β1 integrin and mediating integrin–actin binding. Suppression of calpain-induced talin-1 cleavage with inhibitor compound alleviates proteinuria, and ablation of talin-1 in podocytes leads to severe nephrosis with FPE [15]. Clinically, podocytes and certain podocyte-specific protein products can be detected in the urine of patients with proteinuric renal disease, but not in healthy subjects or in nonpodocyte glomerular disease [13]. A study by Ye et al. has shown that podocyte present in urine is thought to be a more sensitive marker than proteinuria. The major underlying mechanism of podocyte loss is detachment of podocytes from the GBM, which contributes to the progression of CKD [16].
Podocyte Apoptosis
A second cause of the loss of podocytes is augmented apoptosis. Podocyte apoptosis leads to proteinuria and/or glomerulosclerosis. Bottinger is among the first to report augmented apoptosis of podocytes responding to TGF-β in a transgenic mouse model. The cytokine TGF-β and its receptors are upregulated in a variety of podocytopathic diseases, including membranous nephropathy (MN), DN, and FSGS. Proteinuria in turn accelerates podocyte loss or death. One study has revealed that upregulation of podocyte Notch1 in models of DN and FSGS is associated with proteinuria and glomerulosclerosis by Notch1-driven podocyte apoptosis via a p53-mediated pathway [17]. In vitro study, a Notch2 knockdown increases podocyte apoptosis. Inhibition of Notch2 by its specific antibody alleviates proteinuria in a FSGS mouse model [18]. Nevertheless, research indicates that podocyte apoptosis may not be the main cause of the loss of podocytes [19]. Many findings about podocyte apoptosis are obtained in in vitro studies; thus, whether these studies are reliable—from the point of view of proving that apoptosis is the common mechanism of podocyte loss in situ—needs further investigation.
2.2.2 Mesangial Expansion and/or Proliferation
The role of mesangial cells can be investigated by the injurious consequences of accumulation of mesangial immune complexes with subsequent complement activation and production of mediators of inflammation, such as prostanoids; platelet-activating factor (PAF); reactive oxygen species (ROS); cytokines such as IL-6, TNF-α, CSF-1; and chemokines. Immune complexes such as those involving IgA get deposited in the mesangium with simultaneous complement activation. In response to these aberrant immune complexes, mesangial cells are activated and produce several mediators of inflammation, such as chemokines, cytokines, and growth factors, such as mesangial cells bFGF, PDGF, and TGF-β. These may result in mesangial-cell proliferation and matrix expansion. The production of proinflammatory mediators by mesangial cells may also cause a deleterious circle between these mediators, altering the endothelial barrier, allowing more macromolecules to enter the mesangium, and further accelerating the production of inflammatory mediators, ultimately leading to local leukocyte adhesion, activation, and extravasation. Finally, a number of inflammatory mediators produced by mesangial cells (in response to immune complex deposition) and by the infiltrating leukocytes may also change glomerular permselectivity by affecting podocyte function, eventually leading to proteinuria.
Besides being activated by the immune complex, mesangial cells can be activated by advanced glycation end products via binding to their receptor on mesangial cells in diabetes. Furthermore, production of various growth factors, matrix components, and alteration of matrix metabolism by mesangial cells can also be caused by glomerular hypertension in the early phage of diabetes, even in the absence of systemic hypertension. The resulting appearance is early mesangial-cell hypertrophy, proliferation, and deposition of mesangial matrix. The pathological manifestation of DN is characterized by the formation of nodular glomerulosclerosis first described by Kimmelstiel and Wilson. Finally, activation of mesangial cells by hyperglycemia and the sequelae mentioned above also produce chemokines that involve in the leukocytes infiltration, thereby provoking destructive proapoptotic and profibrotic responses. Consequently, mesangial cells significantly participate in the initiation and progression of diabetic glomerulopathy. Besides, changes in mesangial cell biological functions can also be noted in other glomerular diseases, such as amyloidosis and light chain deposition diseases.
2.3 Renal Interstitial Fibrosis
- 1.
After a sustained injury (e.g., proteinuria, high concentration of glucose, or hypoxemia), kidney-resident cells are damaged and release chemotactic factors providing a signal that attracts inflammatory cells to infiltrate into the injury site.
- 2.
The infiltrating inflammatory cells producing various compounds including ROS and multiple protein factors, such as MCP-1, TNF-α, IL-1, TGF-β1, CTGF, and angiotensin II (Ang II), aggravate renal-cell injury. This series of events induces fibroblasts and other cell types, including tubular epithelial cells, pericytes, and endothelial cells, to undergo phenotypic activation or transition and to produce a great amount of ECM components.
- 3.
Activated myofibroblasts from different sources generate ECM, as well as promoting excessive ECM deposition in renal interstitium that results in renal tubular apoptosis and atrophy.
- 4.
Over-generation of extracellular matrix and their degradation defects are accountable for excessive matrix accumulation in the renal interstitium. In the early stage of kidney interstitial fibrosis, the fibrosis may be reversible due to the extracellular matrix prone to proteolysis. Nonetheless, as injuries are sustained and fibrosis progresses, cross-linking with tissue transglutaminase and lysyl oxidase is thought to modify matrix in late stage of renal fibrosis, eventually leading to make the matrix stiff and highly resistant to proteolysis [20]. Excessive deposition of the ECM results in the ultimate destruction of renal parenchyma, microvascular rarefaction, and loss of kidney function.
Due to the limitations of space, we are going to focus on the principal cell types that are responsible for the process of interstitial fibrosis.
2.3.1 Activation of Fibroblasts and Pericytes
In healthy adult kidneys, fibroblasts are located in the interstitial space between capillaries and epithelia, and interact with each other throughout the entire kidney, therefore stabilizing tissue architecture and matrix homeostasis via production of a basal amount of ECM molecules under physiological conditions [21]. In a resting quiescent state, interstitial fibroblasts express some relatively specific markers, including CD73 (also known as ecto-5′-nucleotidase), PDGF-β, and fibroblast-specific protein 1 (FSP1; also known as S100A4). None of these markers, however, is specific for fibroblasts. Profibrotic factors and mechanical stress trigger fibroblast differentiation into αSMA-expressing myofibroblasts. A study by LeBleu et al. shows that 50% of the total pool of myofibroblasts arises from local resident fibroblasts through proliferation during the process of unilateral ureteral obstruction (UUO) [22]. As in resident fibroblasts, there are no universal pericyte markers, whereas kidney pericytes express a number of typical pericyte markers, including PDGFR-β, PDGFR-α, CD248, CD146, desmin, and others [23].
Research suggests that vascular pericytes are also a major source of myofibroblasts in fibrotic kidneys [24, 25]. Pericytes are defined anatomically as cells of mesenchymal origin attached to capillaries that share a common basement membrane and form junctions with endothelial cells [23]. Following kidney injury, pericytes get detached from the endothelium, undergo migration and proliferation, and differentiate into myofibroblasts [26]. The detachment of pericytes and their differentiation into myofibroblasts not only lead to instability of the microvasculature, but also contribute to activation of myofibroblasts, which results in interstitial fibrosis. In addition, pericytes play pivotal roles in sensing of injury, the regulation of recruitment of leukocytes, and perpetuation of inflammation.
2.3.2 Epithelial–Mesenchymal Transition (EMT)
Another origin of cells producing matrix may be the tubular epithelium that involves in EMT: a phenotypic transformation program is that is characterized by the loss of epithelial markers and a gain of mesenchymal features. Similarly, capillary endothelium via endothelial–mesenchymal transition (EndoMT) may be another source of fibroblasts and/or myofibroblasts. EndoMT is recognized as a special form of EMT as endothelial cells are thought as a specialized type of epithelia. That tubular epithelial cells undergo EMT in vitro is not debated. Whether this transition occurs in vivo is at the center of a controversy. An early study has revealed that tubular epithelium contributes to over one-third of FSP1+ interstitial fibroblasts by using bone marrow chimeras and transgenic reporter mice in a UUO model [27]. Meanwhile, two studies also have revealed that EndoMT occurring in various fibrotic kidney diseases significantly participates in the formation of fibroblast and/or myofibroblasts. Nonetheless, some studies question the above experimental results and find that no or only a small number of epithelial or endothelial cells participate in EMT [26, 28].
In 2015, however, two important studies readdressed this debate and shed light on the potential impacts of tubular EMT in the initiation and development of kidney interstitial fibrosis [29, 30]. These studies clarify the issue via several new models of genetically modified mice, in which Snail or Twist1, two key transcription factors regulating the EMT program, are specifically deleted in tubules. Consequently, the EMT program is suppressed significantly in the renal tubular cells in vivo. Both studies show that suppression of the EMT program by tubular-specific deletion of Snail or Twist1 attenuates interstitial fibrosis in several CKD models, including UUO, nephrotoxic-serum-induced nephritis, and folic-acid-induced nephropathy. Thus, the EMT program is pivotal and required for provoking tubular dysfunction and promoting fibrosis progression under pathological conditions.
2.3.3 Recruitment of Circulating Fibrocytes
Fibrocytes are recognized as bone marrow-derived cells that circulate in the peripheral blood and generate matrix components such as collagens and vimentin. Fibrocytes may be identified by dual positivity for CD34 or CD45 and type I collagen or procollagen 1. A study suggests that three markers, CD45RO, 25F9, and S100A8/A9, can distinguish monocyte-derived fibrocytes from monocytes, macrophages, and fibroblasts [31]. Nevertheless, specific markers of fibrocytes have yet to be identified. It is noteworthy that fibrocytes isolated from humans and mice also express certain chemokine receptors, such as CCR2, CCR3, CCR5, CCR7, and CXCR4, thereby regulating the recruitment to sites of fibrosis. Fibrocytes migrate, infiltrate into renal tissue, and involve in the pathogenesis of kidney fibrosis following initial renal injury. Other immune cells like CD4+ T cells can modulate the Gr1+ monocytes differentiation into fibrocytes via secretion of cytokines [32]. Profibrotic cytokines IL-4 and IL-13 promote fibrocytic differentiation, whereas antifibrotic cytokines IFN-γ and IL-12 inhibit this process, suggesting that an inflamed milieu that contains a complex mixture of cytokines is a major determinant of fibrocytic differentiation.
The relative contribution of fibrocytes to renal fibrogenesis is another field full of controversy. Studies by different labs have led to different conclusions. It is a great challenge to clearly discriminate fibrocytes from monocytes/macrophages, fibroblasts, and myofibroblasts due to the fact that there is no specific marker for fibrocytes. In addition, subpopulations of fibrocytes seem to exist [31]. Until now, results of studies on the contribution of fibrocytes in the pathogenesis of kidney interstitial fibrosis are controversial [27, 32, 33].
Over the years, board agreement has been reached that myofibroblasts in renal interstitial fibrosis have a variety of origins. They accomplish tissue repair and remodeling through synthesis and organization of the ECM that causes scarring. Myofibroblasts form from fibroblastic cells (via differentiation) that have distinct biological features, thus supporting the concept of phenotypic heterogeneity of fibroblasts. A better understanding of what cell types generate a larger amount of ECM components and how they are regulated under pathological conditions may shed new light on designing effective therapeutic strategies.
2.4 Key Molecules or Events in CKD
2.4.1 RAAS
The kidney has all the components of the RAAS, and Ang II formation in kidney does not depend on the circulating RAAS. Ang II acting through AT1 receptors activates local components of the RAAS inside the kidney. Meanwhile, Ang II acting as contracting factor mediates intraglomerular hypertension that contributes to impairment of glomerular endothelial, podocytes, and mesangial cells. Moreover, aldosterone and Ang II perform a number of nonhemodynamic functions that are critical for the pathogenesis of CKD, including effects on inflammation, ECM production, endothelial dysfunction, and ROS generation.
Ang II as a main vasoconstrictor of RAAS predominantly effects on postglomerular arterioles, which leads to intraglomerular hypertension, an increase in filtration, and impairment of the size-selective function of glomerular barrier toward macromolecules, such as plasma protein. Glomerular hypertension and outflow of plasma protein may be responsible for the initiation and progression of chronic kidney diseases. The nonhemodynamic effects of Ang II, such as upregulation of cytokines, increased generation of ROS, and cell adhesion molecules, play important roles in driving progression of kidney disease. In addition, Ang II is thought to impair the integrity of glomerular filtration barrier by decreasing negative charged proteoglycans synthesis and inhibiting nephrin expression [34]. Consequently, Ang II via hemodynamic and nonhemodynamic effects—leads to proteinuria.
The RAAS participates in interstitial fibrosis mainly by promoting the proliferation of fibroblasts, enhancing EMT, increasing production of TGF-β, and by promoting the imbalance between ECM synthesis and degradation. Studies have uncovered a close link between the RAAS and profibrotic cytokine TGF-β. Ang II not only promotes TGF-β expression through several pathways, but also stimulates expression of receptors for TGF-β. Furthermore, SMADs phosphorylation can be directly regulated by Ang II without induction of TGF-β. In addition, other molecules of the RAAS, such as renin, Ang III, and aldosterone also promote activation of TGF-β system. Given that therapeutic strategies for directly targeting TGF-β system in human diseases have not been feasible, angiotensin-converting enzyme (ACE) inhibitors and AT1 receptor blockers are currently the most promising drugs for interfering with this Ang-II-induced TGF-β system activation. Additionally, Ang II can induce PAI-1 and tissue inhibitor of matrix metalloproteinases 1 (TIMP-1) via AT1 receptors. Meanwhile, the RAAS participates in EMT too. Aside from the mechanism mentioned above, Ang II activates (through AT1 and AT2 receptors) the proinflammatory transcription factor NF-κB [35], enhancing an inflammatory response and contributing to renal interstitial fibrosis. In contrast, one study conducted by Zhang et al. indicates that activation of AT1 receptors on macrophages can ameliorate kidney fibrosis by inhibiting macrophage M1 polarization and by reducing the inflammatory response. This study may remind us that the RAAS acting on different cell lineages plays various roles during interstitial fibrogenesis, and angiotensin receptor blockade is related not only to previously recognized side effects but also to patently detrimental effects of blocking angiotensin receptors on hematopoietic cells [36].
Sustained activation of the intrarenal RAAS results in renal injury and plays a pivotal part in the pathogenesis of CKD. This fascinating system is found to be increasingly complex as it is characterized, and research into new members continues. Identification and characterization of the RAAS together with novel pharmacological approaches that target its components—we hope will provide methods to sufficiently retard the progression and to induce regression of kidney disease.
2.4.2 TGF-β
TGF-β is a key mediator of implicating in the regulation of fibrogenesis, cell proliferation, apoptosis, and hypertrophy, contributing to kidney fibrosis and progressive CKD. The TGF-β superfamily consists of TGF-β1, -β2, and, -β3; activins; and bone morphogenic proteins (BMPs). Canonical and noncanonical signaling cascades can be provoked by TGF-β to exert various biological functions. SMAD signaling is thought to be a major pathway among TGF-β-driven signalings in progressive renal fibrosis. TGF-β signaling is initiated when ligand-bound type II TGF-β receptor (TβRII) binds to (and phosphorylates) type I TGF-β receptor (TβRI) in the TβRI cytoplasmic glycine and serine (GS) region. Then, SMAD2 and/or -3 are highly activated along with a common SMAD: SMAD4. These SMAD proteins form an oligomeric complex and then are translocated into the nucleus to regulate the transcription of target genes in collaboration with various coactivators and corepressors. Activation of SMAD3 is associated with the downregulation of inhibitory SMAD7 via an ubiquitin E3 ligase-dependent degradation mechanism. Additionally, TGF-β can function via SMAD-independent pathways, including p38, ERK, MAPK, Rho GTPases, Rac, Cdc42, ILK, β-catenin, and PI3K–Akt–mTOR cascades.
The cytokine TGF-β is closely associated with glomerular disease. This cytokine and its receptors are upregulated in a variety of podocyte diseases, including MN, DN, and FSGS. Increased urinary concentration of TGF-β is detected in patients with some forms of nephrotic syndrome, IgAN, and FSGS. Of note, detection of TGF-β in urine can differentiate between FSGS and the nonfibrotic process of minimal change disease (MCD). In addition to its profibrotic effects, TGF-β signaling induces apoptosis. As described above, glomerulosclerosis in animal models and humans is characterized in part by depletion of podocytes. TGF-β1 and SMAD7 synergize to induce apoptosis in podocytes in vitro. A study by Shankland’s group suggests that CDK inhibitor p21 is necessary for TGF-β-induced podocyte apoptosis [37]. In a TGF-β1 transgenic model of progressive glomerulosclerosis, the time point of peak podocyte apoptosis coincides with expression of TGF-β1 and SMAD7, and with the onset of albuminuria, but precedes mesangial expansion [38]. In addition, podocyte depletion may cause decreased expression of VEGF, which acts on endothelial cells and promotes their survival [39]. These pathogenic events mediated by TGF-β signaling may represent the pathological mechanism behind podocyte depletion and progressive glomerulosclerosis.
Additionally, TGF-β signaling mediates mesangial-cell-induced glomerulosclerosis. TGF-β1 stimulates production of type I and IV collagens and fibronectin in mesangial cells, leading to glomerular-matrix accumulation in the pathogenesis of glomerulosclerosis. In addition, TGF-β1 inhibits plasminogen activator production, and this effect stimulates PAI synthesis by normal glomeruli. Moreover, TGF-β can rapidly stimulate Ca2+ influx, without promoting a Ca2+ release, thereby further promoting cytoskeletal rearrangement and increasing incorporation of αSMA into stress fibers in mesangial cells [40].
TGF-β exhibits profibrotic effects during kidney interstitial fibrosis potentially via several mechanisms below: (1) As mentioned above, kidney-resident and -nonresident cells can transdifferentiate into myofibroblasts under the action of TGF-β. TGF-β treatment drives transdifferentiation of endothelial and epithelial cells in myofibroblast-like cells, whereas inhibiting TGF-β–SMAD signaling with antagonists or blockers ameliorates or reverses the development of EndMT or EMT [6, 41]. (2) TGF-β1 directly promotes generation of the ECM, including fibronectin and type I collagen, via dependent or independent of SMAD3 pathways. (3) TGF-β1 inhibits ECM degradation via MMPs inhibition but TIMPs induction. (4) TGF-β1 directly exerts effects on various kidney-resident cells to perform different functions; for instance, it induces fibroblast proliferation to generate more matrix or may promote tubular epithelial cells apoptosis, and this effect may cause more severe renal interstitial fibrosis and incur more damage to kidney diseases.
Not surprisingly, due to the importance of TGF-β signaling, therapeutic agents that inhibit TGF-β signaling have been shown to reduce matrix accumulation in animal models of diabetes, puromycin nephropathy, and UUO. Many potential therapeutic approaches based on inhibition of TGF-β have been tested in experimental models of CKD, such as the administration of neutralizing anti-TGF-β antibodies, a soluble TGF-β receptor, or small interfering RNAs that target TGF-β type II receptor. These therapies reduce structural renal injury and decrease renal fibrosis. In 2011, the results of a phase I clinical trial of fresolimumab, an anti-TGF-β antibody, revealed that this agent is well tolerated by patients with FSGS [42]. Phase II trials of another anti-TGF-β antibody, LY2382770, have been stopped early because of futility. Although TGF-β is generally considered a central mediator of fibrotic diseases, inhibition of TGF-β and of its downstream targets has a long way to go before adoption in the clinic.
2.4.3 Wnt Signaling Pathway
Wnt signaling has been recognized for its important functions not only in carcinogenesis but also in embryonic development and in the stem cell compartment. To date, 19 Wnt ligands and 15 receptors and coreceptors have been identified. Many Wnt ligands act through the canonical Wnt pathway in which β-catenin is a key mediator. The Wnt–β-catenin cascade is silenced in healthy adult kidneys and is reactivated in adult kidneys after injury. Aberrant activation of Wnt–β-catenin signaling is associated with proteinuria, renal function decline, and kidney fibrosis in many forms of CKD, regardless of whether the injury initially occurs in the renal tubulointerstitium or glomeruli [7, 43]. Wnt expression increases in the kidneys of animal models of CKD. Noncanonical Wnt pathways include the planar cell polarity (Wnt–PCP) and Wnt–Ca2+ signals. Due to the importance of Wnt–β-catenin signaling, we will focus on the involvement of this signaling in kidney fibrosis.
Increased Wnt activity has been observed in glomeruli of mouse models of CKD. Wnt expression increases in the podocytes of mice treated with Adriamycin, a drug that induces podocyte injury with features similar to those of FSGS [7]. Wnt expression increases in the podocytes of mice treated with adriamycin, a drug that induces podocyte injury with features similar to those of FSGS. Tail vein injection of exogenous Wnt1 into BALB/c mice exacerbates podocyte dysfunction, as evidenced by decreased protein levels of nephrin. Blocking Wnt signaling with DKK1 in adriamycin-injected mice ameliorates albuminuria and counteracts the decrease in nephrin levels [7]. Furthermore, a podocyte-specific knockout of β-catenin protects mice from adriamycin-induced podocyte injury, including albuminuria and podocyte FPE [7]. ICG-001 also can effectively attenuate proteinuria in a model of adriamycin-induced nephropathy. Wnt signaling elicits its biological effects by promoting the expression of many different target genes. Following Wnt’s binding to a cell membrane receptor and induction of a series of downstream signaling events, active β-catenin is translocated into the nucleus, binds to TCF and/or LEF transcription factors, and recruits coactivators—including CREB-binding protein (CBP) or its closely related protein p300—to form a transcriptionally active complex. The latter promotes target genes expression, such as those encoding Snail1, RAS components, MMP-7, TRPC6, FSP1, PAI-1, and fibronectin. These target genes inhibit the expression of proteins associated with the podocyte slit diaphragm, facilitate cytoskeletal organization and contraction, or promote podocyte EMT or apoptosis, thereby leading to impairment of podocyte function and perturbing slit-diaphragm integrity under pathological conditions.
The levels of many Wnt proteins and Fzd receptors are evaluated in tubular epithelium in fibrotic kidneys. In CKD, Wnt and β-catenin not only show alterations of expression, but also participate in initiation and progression of kidney fibrosis. Overexpression active form of β-catenin in tubular cells provokes dedifferentiation of tubular cells and EMT in mice. Consistent with this result, reduced Wnt/β-catenin activation closely correlates with improvement of renal fibrosis. Interventions into Wnt signaling at different levels have been studied. DKK1 is a Wnt antagonist that binds to receptor LRP5 or LRP6 and inhibits the canonical Wnt pathway. Injection of a vector encoding DKK1 into a mouse model of kidney fibrosis reduces β-catenin accumulation and fibrosis. Similarly, inhibition of Wnt–β-catenin signaling by other antagonists including sFRP4, Klotho, and the small-molecule inhibitor ICG-001 represses myofibroblast activation and reduces renal interstitial fibrosis. Emerging evidence indicates that depending on the magnitude and duration of its activation, Wnt–β-catenin signaling performs a dual function: promoting repair or regeneration or facilitating progression to CKD after acute kidney injury (AKI). Transient activation of the Wnt–β-catenin pathway is renoprotective because it promotes adaptive repair and recovery, whereas sustained activation of the same signaling cascade is detrimental and triggers maladaptive responses, leading to the onset and progression of CKD. Feng et al. have reported that Wnt–β-catenin signaling in macrophages stimulated by Wnt3a contributes to IL-4- or TGF-β-induced macrophage M2 polarization and the phosphorylation and nuclear translocation of STAT3 in vitro; by contrast, inhibition of Wnt–β-catenin signaling prevents these IL-4- or TGF-β1-induced processes. Mice with a macrophage-specific β-catenin knockout show decreased macrophage accumulation, attenuated M2 polarization, and alleviation of kidney fibrosis after UUO [44]. This evidence suggests that Wnt–β-catenin signaling in macrophages as well as in tubular cells and fibroblasts is likely to play a key role in the pathogenesis of interstitial fibrosis.
2.4.4 mTOR Signaling
mTOR, a highly conserved serine-threonine kinase, is recognized for a critical regulator of cell proliferation, cell growth, and metabolism. Mounting evidence exhibits that mTOR performs a pivotal function in the control of kidney cell homeostasis and autophagy. mTOR kinase exists in two distinct protein complexes: mTOR complex 1 (mTORC1) and mTOR complex 2 (mTORC2). mTORC1 as a “nutrient sensor” is activated by amino acids and suppressed by oxidative stress and energy depletion. mTORC1 is also regulated by growth factors and cytokines via phosphoinositide 3-kinase (PI3K) signaling pathway. Phosphatidylinositol (3, 4, 5) triphosphage (PIP3) generated by PI3K stimulates 3-phosphoinositide-dependent protein kinase 1 (PDK1), which promotes Akt (protein kinase B) activity via phosphorylating of the activation loop at threonine 308. Moreover, mTORC2-mediated phosphorylation of both the turn motif in AKT (Thr450) and hydrophobic motif in ATK (Ser473) stabilizes and activates Akt. The activation of tuberous sclerosis complex 1 (TSC1)-TSC2 negatively regulates mTORC1 and is directly inhibited by Akt-dependent phosphorylation. TSC1-TSC2 complex therefore serve as an intermediary between PI3K-Akt signaling and mTOR. TSC1 maintains but TSC2 suppresses the activity of the guanosine triphosphatase (GTPase) RAS homolog enriched in brain (Rheb) via its GTPase-activating protein activity. Rheb provokes mTORC1 activation when they are close to each other [45]. The mTORC2 kinase mainly controls many cellular parameters and processes, including protein synthesis, cell survival, rearrangement of cell cytoskeleton, and sodium homeostasis. Akt, serum, and glucocorticoid-induced kinase 1 (SGK-1), and protein kinase Cα (PKCα) are all substrates of mTORC2 [46]. Moreover, mTOCR2 can phosphorylate and sequester forkhead box proteins O1 (FOXO1) and FOXO3 via Akt [47]. The function of mTORC2 is not as clear as that of mTORC1 due to lack of specific inhibitor of mTORC2 kinase.
Rapamycin as mTOR inhibitor induces significant ultrastructural and molecular changes in podocytes including increased foot process width, decreased Nephrin and Podocin mRNA levels, which are associated with albuminuria in BALB mice with normal renal conditions. These manifestations are finally ameliorated at week 8 after rapamycin-treated groups [48]. By contrast, mice with adriamycin-induced nephropathy have significantly reduced proteinuria and preserve renal function, with only mild histological abnormalities [49]. Gene knockout approaches provide us to well understand the functions of mTORC1 and mTORC2 in podocyte. Genetic deletion of mTOCRC1 in mouse podocytes induces proteinuria and progressive glomerulosclerosis, weight loss, and increased mortality [50]. Genetic deletion of mTORC2 in mouse podocytes does not manifest significant phenotypic differences from littermate controls. This result is suggestive of a less important role of mTORC2 in podocytes. Nevertheless, abrogation of both mTORC1 and mTORC2 in podocytes precipitates an early fulminant proteinuric phenotype. This scenario may suggest that interaction between mTORC1 and mTORC2 may regulate podocyte development and homeostasis.
Conversely, podocyte-specific deletion of Tsc1 results in excessive mTORC1 activity, which can lead to severe pathological effects. Increased activation of mTORC1 causes increased pS6 expression, glomerular hypertrophy, GBM thickening, podocyte foot process broadening and effacement, and proteinuria, which can be ameliorated by rapamycin [50]. mTORC1 excessive activation in podocytes also leads to the progression of glomerular crescents, which is abrogated by rapamycin treatment. In cellular crescents from patients with crescentic glomerular diseases, mTORC1 signaling is remarkably activated too [51]. A publication by Canaud G et al. reveals that activation of Akt2 as downstream of mTORC2 is essential to maintain podocyte viability and function during chronic kidney disease [52].
In vitro, mTOR signaling pathway in fibroblasts is exclusively activated by TGF-β via the TSC2, and rapamycin abolishes TGF-β-induced upregulation of Rheb-mTORC1 signaling in interstitial myofibroblasts. Genetically modified mice with either ectopic expression of Rheb or fibroblast-specific deletion of TSC1 exhibit activated mTORC1 signaling in kidney interstitial fibroblasts and increased renal interstitial fibrosis, which can be abrogated by rapamycin as well [53]. Mounting evidence have revealed that treatment with rapamycin after renal damage, regardless of etiology, slows the subsequent progression of interstitial fibrosis caused by ischemic-reperfusion injury (IRI), transplantation, UUO, and/or glomerulopathy.
Li et al. have found that RICTOR–mTORC2 signaling can be activated in normal rat kidney cells (NRK-49F cell line) treated with TGF-β1 in a time-dependent manner, whereas a knockdown of RICTOR with small interfering RNA inhibits the fibroblast activation characterized by the expression of fibronectin and smooth muscle actin. In vivo, mice with a fibroblast-specific knockout of RICTOR show lower collagen content and fibrosis, apoptosis, and inflammatory cell infiltration in the UUO kidney as compared with control littermates [54]. Our group has also reported that RICTOR–mTORC2 signaling activation in macrophages drives macrophage M2 polarization, a release of multiple profibrotic factors and proliferation, which eventually induce kidney interstitial fibrosis [55].
2.4.5 MicroRNAs (miRNAs)
MiRNAs are endogenous single-stranded noncoding RNA molecule (containing about 22 nucleotides). MiRNAs have multiple key roles in regulating various biological functions such as cell differentiation, proliferation, development, and immune responses. Thus, dysregulation of miRNAs seems to result in disturbances of target gene networks and signal transduction culminating in disease onset and/or development. The biogenesis of miRNAs is detailed well in many reviews [56]. RNA polymerases II and III are responsible for miRNA transcription to generate precursors that is cleaved into mature miRNA. The regulatory functions of miRNAs are executed by the RNA-induced silencing complex (RISC). The latter is assembled from miRNA and other components and is thus activated to target messenger RNA (mRNA) specified by the miRNA.
MiRNAs are crucial modulators of nephron development. Dicer is an important RNase III family enzyme cleaving precursor miRNAs into RNA duplexes during the biogenesis of miRNAs. Specific deletion of Dicer in podocytes leads to formation of FPE, proteinuria, interstitial fibrosis, glomerulosclerosis, and eventually the animal dies several weeks later due to renal failure [57]. Several miRNAs (miR-23b, miR-24, miR-26a, and the miR-30 family) are thought to be closely related with abnormalities of podocyte phenotype. The miR-30 family has been shown to target several mRNA that mediate podocyte apoptosis and cytoskeletal arrangement. Drosha is another important enzyme accounting for miRNA synthesis, and specific deletion of Drosha in podocytes present similar phenotypes as in mice with Dicer deficiency [58]. Dicer deficiency in renin-expressing juxtaglomerular cells reduces cell number [59]. These results support the important roles of miRNA in maintaining biological functions of nephron. In addition to podocytes, mesangial cells are regulated by miRNAs too. MiR-34a and miR-335 potentially induce mesangial-cell senescence by inhibiting mitochondrial antioxidative enzymes superoxide dismutase 2 and thioredoxin reductase 2. Akt activation through PTEN downregulation by miRNA-216a and miRNA-217, which are regulated by upstream miR-192 and TGF-β, leading to glomerular mesangial-cell hypertrophy and survival, and playing important roles in diabetic changes in kidneys. Moreover, miR-377 overexpression in mesangial cells can trigger production of fibronectin and expansion of the mesangial matrix by downregulating serine-threonine protein kinase PAK1 and superoxide dismutase.
The regulation of miRNAs is closely associated with profibrotic cytokine TGF-β in interstitial fibrosis. MiR-382 is induced in UUO-induced obstructed kidneys as well as in cultured proximal tubular cells treated with TGF-β1. MiR-382 potentially suppresses Kallikrein 5, a crucial enzyme that mediates the degradation of ECM components. Thus, blockade of miR-382 via a locked nucleic acid inhibitor apparently attenuates kidney tubulointerstitial fibrosis [60]. Furthermore, TGF-β1 acts by causing SMAD3 to positively regulate miR-21 and miR-192 expression but negatively regulate the expression of miR-29 or miR-200 families, thereby mediating renal fibrosis [61], whereas SMAD7 can antagonize the miR-21 induction [62]. MiR-21 inhibition or deletion significantly reduces interstitial fibrosis after UUO possibly by targeting the lipid metabolic pathway regulated by peroxisome proliferator-activated receptor alpha (PPAR-α) [63]. In addition, the TGF-β cascade is responsible for the inhibition of miR-29 and miR-200 and for induction of miR-192 and miR-491-5p in the UUO model; these effects in turn promote the development of renal injury [62]. Besides, miR-192 is a major contributor to the phenotype switch of tubular cells during renal tubulointerstitial fibrosis. Additionally, it has been suggested that renal miR-433 expression induced by TGF-β promotes renal interstitial fibrosis [64]. MiR-200 and miR-141 are necessary for the development and progression of TGF-β1-dependent EMT and interstitial fibrosis in vitro and in vivo [65].
Of note, miRNAs are detectable in various body fluids such as saliva, tears, serum, and urine. And they are much more stable than mRNAs [66]. Based on this fact, clinically, miRNAs are likely to be used as biomarkers for diagnosis and prognostication of human diseases.
2.4.6 Hypoxia-Induced Factor (HIF)
HIF, known as a basic heterodimeric transcription factor, is composed of two protein subunits: HIF-α and HIF-β. In normal oxygen condition, HIF-α subunit is sensitive to oxygen and regulated by O2-dependent prolyl hydroxylation, which targets the protein for ubiquitylation by von Hippel-Lindau (VHL) tumor suppressor. Ubiquitylated HIF-α is rapidly targeted for degradation. Under hypoxic conditions or loss-of-function VHL, HIF-α does not undergo degradation and subsequently translocates into nucleus, where it binds to HIF-β to form constitutively active HIF. After binding to the cis-acting hypoxia-responsive element, HIF induces a variety of target gene expression, which are adaptive response to hypoxic conditions. More than that, HIF-regulated target genes involved in angiogenesis, erythropoiesis, and energy metabolism, also favor adaptation to oxygen depletion and oxygen delivery. As a transcription factor, HIF is involved in the regulation of many biological processes that facilitate both oxygen delivery and adaptation to oxygen deprivation by regulating genes that participate in glucose uptake, energy metabolism, angiogenesis, erythropoiesis, cell proliferation, apoptosis, cell–cell and cell–matrix interactions, and barrier function.
HIFs represent a critical initial signal for the inception of glomerular capillary morphogenesis [67]. In the settings of renal glomerular diseases, podocyte-specific activation of HIF induced by a VHL knockout induces activation and proliferation of podocytes and rapidly progressive glomerulonephritis and renal failure [68]. Likewise, HIF-α hyperstabilization induces widespread podocyte foot process broadening, GBM thickening, and ectopic deposition of collagen α1α2α1 (IV) in GBM humps beneath podocytes [69]. Endothelial cells are deeply involved in CKD. Nonetheless, the functions of HIF-1 and HIF-2 in the glomerular endothelium may be different in the different contexts of kidney diseases. In a kidney chronically injured by Ang II-induced hypertension, elevated endothelial HIF-1α amounts contribute to the initial glomerular injury, leading to hypertension and progression of renal fibrosis. Endothelial HIF-2, but not HIF-1, regulates renal inflammation likely through suppression of VCAM1 expression and protects from hypoxia-induced renal damage [70]. Studies by Kalucka et al. indicate that specific deletion of HIF-1α in endothelial cells of glomerular induces hypoxic cell death in vitro; however, a loss of HIF-1α in endothelial cells does not significantly effect on the development profile of kidneys and does not influence renal function or the expression of adhesion proteins in the pathogenesis of fibrosis after UUO [71].
Accumulating evidence highlights chronic hypoxia in the tubulointerstitium as a final common pathway to end-stage renal disease [72]. A fibrotic kidney with advanced renal disease is devoid of peritubular capillary blood supply to (and oxygenation of) the corresponding region. In this context, of note, hypoxia per se induces a fibrogenic response which would active renal microvascular endothelial cells, tubular cells, and interstitial fibroblasts. Hypoxia is also a profibrogenic stimulation for changing the ECM homeostasis of resident cells in kidney, and then leading to peritubular capillaries rarefaction. In the setting of hypoxia, tubular cells could transdifferentiate to myofibroblasts. In addition, severe and/or prolonged hypoxia can cause kidney epithelia dysfunction that accounts for energy metabolism imbalance and subsequent cell apoptosis. The reciprocal relation between hypoxia and development of renal failure may provide us new therapeutic strategies for patients with chronic kidney diseases.
2.4.7 Defective Energy Metabolism
The kidney uses a large amount of energy, most of which is dedicated to cell structure maintenance and solute reabsorption. ATP, which is called the molecular unit of intracellular energy currency, is essential for normal cellular processes. Disturbed production of ATP during energy metabolism, e.g., mitochondrial dysfunction or dysregulation of key metabolic enzymes by various insults, will cause a cellular structural abnormality, apoptosis, or differentiation.
Mitochondria supply most of ATP to the cell via their oxidative phosphorylation (OXPHOS) system. Mitochondria are highly dynamic organelles. One of the most unique features of mitochondria is fusion and fission. Frequent cycles of fusion and fission adapt the morphology of the mitochondrial compartment to metabolic needs of the cell. Once the balance between fusion and fission is lost, the cellular functions change. Meanwhile, mitochondrial biogenesis and the regulation of OXPHOS are important for satisfying the specific energy demand of specialized cells. PGC-1α itself is a master cotranscriptional regulator that induces mitochondrial biogenesis by activating various transcription factors. Besides energy supply, mitochondria contribute to calcium signaling, ROS production, and redox homeostasis.
On the other hand, β-oxidation of free fatty acids is one of the major sources of ATP production, particularly in the proximal tubule, which has high energy demand and relatively low glycolytic capacity. Several old studies indicate that ~60% of the energy in kidneys comes from burning of fatty acids. The energy production of fatty acid β-oxidation is high: on average 106 ATP equivalents per fatty acid molecule, as opposed to 36 during oxidation of carbohydrates. The high energy consumption of the proximal tubule is due to the workings of fluid and electrolyte homeostasis, active solute secretion, and hormone production. Sometimes, glycolysis contributes to energy production too, under certain circumstances. Although this metabolic pathway is a less efficient producer of ATP as compared with mitochondria, glycolysis has several advantages. One is glycolysis that can generate additional energy when mitochondria show maximum performance. Another is generation of side products, including amino acids, nucleic acids, and lipids. Nonetheless, under stress, a switch to glycolysis may influence cellular biological functions.
The regulators of energy metabolism help to balance the energy production and consumption for energy homeostasis. AMPK has been identified as the critical molecule for the regulation of metabolism in various cells. AMPK can be activated during energy stress in response to a rise in the AMP/ATP ratio. AMPK is known to be the substrate of LKB1; the latter serves as a crucial metabolic checkpoint factor and arrests cell cycle in response to low intracellular ATP concentration, e.g., during low nutrient availability. Thus, the LKB1–AMPK axis, as the key energy metabolism regulator, plays a vital role in cellular energy homeostasis. mTOR is one of the main players that controls energy-consuming cellular processes such as cell growth and proliferation. mTOR as one of the main regulators controls energy-consuming cellular processes including cell proliferation and growth. mTOR contributes to the increase in oxygen consumption, mitochondrial membrane potential, ATP-synthetic capacity, and mitochondrial content. Thus, mTOR and AMPK coordinately regulate cellular energy homeostasis. mTOR increases mitochondrial membrane potential, oxygen consumption, ATP-synthetic capacity, and mitochondrial content. Therefore, there is a complex relation between AMPK and mTOR in terms of regulating cellular energy homeostasis.
Podocytes have many highly specialized foot processes, which require high energy consumption to maintain their structure and functions. In fact, a considerable number of mitochondrial sections are observed in the narrow peripheral foot processes. Morphological changes of mitochondria are exclusively observed in podocytes among glomerular cells in patients with mitochondrial cytopathy [73]. Mitochondrial activity loss manifesting itself as a smaller energy supply may be accompanied by increased ROS production, thus causing or accelerating podocyte injury. Furthermore, studies suggest that a reduction in the amount of mitochondrial DNA (mtDNA, which is involved in mitochondrial biogenesis and function in podocytes) is associated with the pathogenesis of podocyte injury. CoQ-deficient mice with Pdss2 conditionally knocked out only in podocytes have proteinuria and podocyte FPE [74]. In these mice, mitochondrial OXPHOS capacity is impaired. Research indicates that free-fatty acid accumulation in podocytes is toxic and induces endoplasmic-reticulum stress and podocyte death. Stimulation of fatty acid oxidation by AMPK activators can abrogate the detrimental effect of free-fatty acid accumulation. In contrast, inhibition of fatty acid oxidation by CPT-1 inhibitor etomoxir increases the toxic effect of palmitic acid on podocytes [75]. Proteins involved in the transport and oxidation of fatty acids are affected in podocytes of puromycine aminonucleoside (PAN) glomeruli [76]. It is also reported that glycolysis acts as a supplier of ATP in mouse podocytes although the main supplier is mitochondria and their OXPHOS system [77]. The podocyte’s dependence on glycolysis for ATP production might change in pathological conditions.
Mitochondrial abnormality facilitates the progression of renal interstitial fibrosis, particularly due to a reduction in mtDNA copy number, a loss of mitochondrial membrane potential, and a drop of ATP production. Mitochondrial dysfunction is involved in the apoptosis and EMT of renal tubular epithelial cells and in ROS production, thereby contributing to the fibrogenic process. Stimulation of mitochondrial biogenesis by restoring cAMP levels by means of PDE4 inhibitor rolipram ameliorates UUO-induced renal fibrosis [78]. Emerging evidence suggests that defective energy metabolism—including fatty acid oxidation and glucose metabolism—is associated with CKD [79]. Impaired energy metabolism in general, and reduced fatty acid oxidation in particular, are prominent and consistent features of CKD in both human biopsy samples and several animal models. Additionally, the above study has revealed that the increased tubule-specific triglyceride concentration and higher long-chain fatty acid content after overexpression of CD36 do not induce significant renal fibrosis. These findings indicate that a defect in β-oxidation of free fatty acids is crucial for CKD development [79]. Kang et al. have reported that TGF-β1 can inhibit PPAR-α and PPARGC1A to induce EMT. In another study, the AMPK activator 5-aminoimidazole-4-carboxamide-1β riboside (AICAR) inhibited the activation of myofibroblasts both in a UUO model and in response to stimulation of NRK-49F cells by TGF-β1 [80]. Recently, Ding et al. discovered that a switch of metabolism from OXPHOS to aerobic glycolysis (Warburg effect) in renal fibroblasts is the primary feature of fibroblast activation during UUO-induced renal interstitial fibrosis [81]. In addition, suppression of renal fibroblast aerobic glycolysis significantly reduces UUO-induced interstitial fibrosis [81].
Meanwhile, an imbalance among regulators of energy metabolism may also contribute to CKD. A loss of LKB1 in the distal tubular cells causes CKD characterized by increased matrix deposition [82]. That study has revealed that energy metabolism is diminished in LKB1 renal tubular knockout mice with CKD, and this phenomenon could be retarded by treatment with either the AMPK agonist A769662 or a PPAR-α agonist, fenofibrate. In agreement with this finding, the reduction in AMPK expression both in kidney cell lines—and in AMPK-a2-null mice subjected to UUO—increases levels of markers of EMT and fibrosis. Others have also found that after various profibrotic stimuli, including TGF-β, Ang II, aldosterone, or high glucose and albumin levels, EMT is suppressed by the AMPK activators metformin and AICAR.
2.5 Conclusion

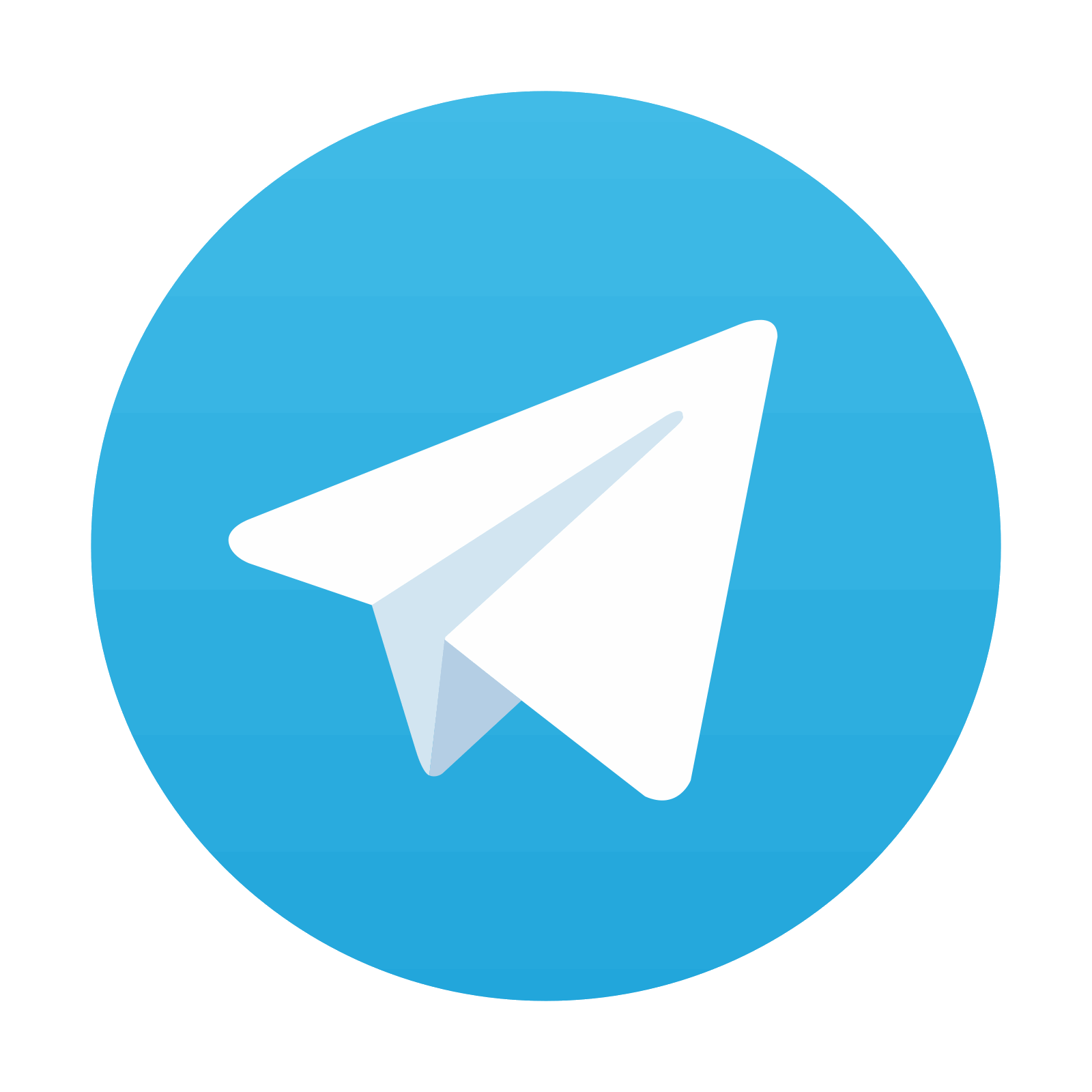
Stay updated, free articles. Join our Telegram channel
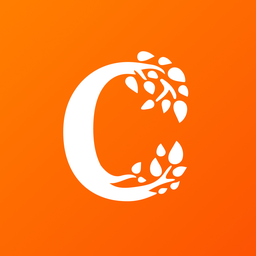
Full access? Get Clinical Tree
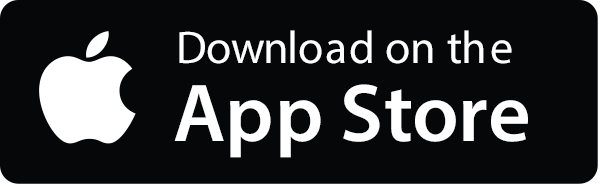
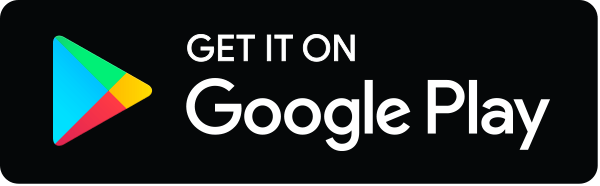