Technique
Resolutiona
Depth
Timeb
Quantitativec
Multichannel
Imaging agents
Target
Costd
Main small animal use
Clinical use
MRI
10–100 μm
No limit
Minutes to hours
Yes
No
Paramagnetic chelates, magnetic particles
Anatomical, physiological, molecular
$$$
Versatile imaging modality with high soft tissue contrast
Yes
CT
50 μm
No limit
Minutes
Yes
No
Iodinated molecules
Anatomical, physiological
$$
Imaging lungs and bone
Yes
Ultrasound
50 μm
cm
Seconds to minutes
Yes
No
Microbubbles
Anatomical, physiological
$$
Vascular and interventional imaginge
Yes
PET
1–2 mm
No limit
Minutes to hours
Yes
No
18 F-, 64Cu-, or 11C-labeled compounds
Physiological, molecular
$$$
Versatile imaging modality with many tracers
Yes
SPECT
1–2 mm
No limits
Minutes to hours
Yes
No
99mTc- or 11In-labeled compounds
Physiological, molecular
$$
Imaging labeled antibodies, proteins and peptides
Yes
Fluorescence
2–3 mm
<1 cm
Seconds to minutes
No
Yes
Photoproteins, fluorochromes
Physiological, molecular
$
Rapid screening of molecular events in surface-based disease
Yes
FMT
1 mm
<10 cm
Minutes to hours
Yes
Yes
Near-infrared fluorochromes
Physiological, molecular
$
Quantitative imaging of fluorochrome reporters
In development
Bioluminescence imaging
Several mm
cm
Minutes
No
Yes
Luciferins
Molecular
$$
Gene expression, cell and bacteria tracking
No
Intravital microscopyf
1 μm
<400–800 μm
Seconds to hours
No
Yes
Photoproteins, fluorochromes
Anatomical, physiological, molecular
$$$
All of the above at higher resolutions but limited depths and coverage
In developmentg
Although still in its infancy, molecular imaging has clinical applications ranging widely from diagnostic uses in disease detection and tumor characterization, real-time guidance in surgery [9], to clinical outcomes such as treatment response and prognosis. In this chapter, we will discuss the urologic applications of existing molecular imaging in clinical practice as well as emerging technologies currently in the preclinical setting.
Molecular Imaging Agents
While some molecular imaging techniques can visualize the intrinsic differences, such as Raman scattering and autofluorescence, between normal and diseased tissues [9, 10], an exogenous molecular imaging agent is generally required [6] and administrated systematically or topically. In general, molecular imaging agents should have high selectivity, minimal background from nonspecific binding, suitable pharmacokinetics, excellent in vivo stability, and good safety profile [6]. While for topical application, the imaging agents are delivered to target organ directly thus smaller amount of imaging agents are required, which together reduce potential toxicity and blood clearance issues from intravenous injection [10]. This is of special importance for endoscopy-based molecular imaging of urothelial malignancies where imaging agents are introduced intravesically. Translational and clinical examples of molecular imaging though topical administration can be found for imaging of gastrointestinal cancer, another epithelial malignancy [11].
An exogenous molecular imaging agent is typically comprised of a targeting molecule and a signaling conjugate, which should not interfere with the function of the targeting molecule. Targeting molecules can be categorized based on size, ranging from small molecules, peptides, engineered proteins (e.g., affibody), aptamers, and monoclonal antibodies (mAb)—each of which possesses different pharmacokinetic and binding properties (Fig. 20.1) [6].
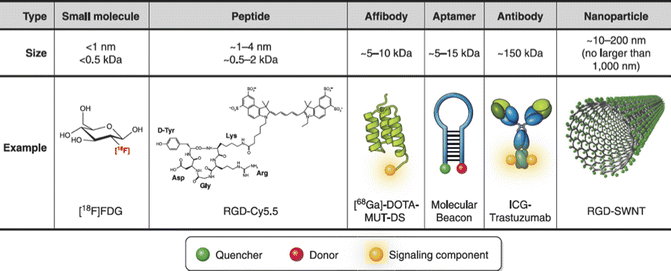
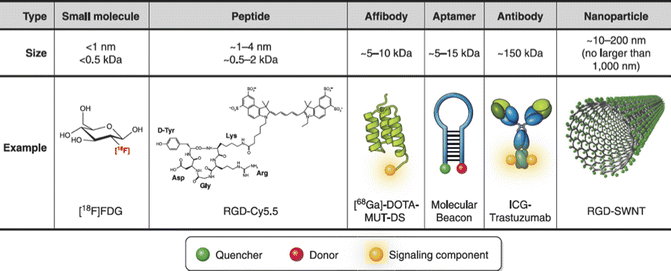
Fig. 20.1
Overview of molecular imaging agents. 18F-FDG is a small molecule PET imaging agent for visualizing activity and levels of hexokinase type II and glucose transporter I. RGD peptide labeled with cyanine 5.5 (RGD-Cy5.5) is a fluorescent peptide imaging agent for imaging alpha-v beta-3 (αvβ3) integrins. Affibody PET agent gallium-68-labeled DOTA-MUT-DS (68Ga-DOTA-MUT-DS) and fluorescent mAb agent ICG-trastuzumab are both used to image human epidermal growth factor receptor type 2 (HER2). A general representation of an aptamer molecular beacon (also known as smart/activatable probe) is shown as an example of an aptamer imaging agent. Lastly, RGD-SWNT is depicted as an example of a nanoparticle imaging agent (From James and Gambhir [6], with permission from The American Physiological Society)
Small molecules (usually <500 Da) have the smallest size among all molecular imaging agent, which allows them to access and image intracellular targets. Unlike other molecular imaging agents that enable imaging by direct binding to specific target, small molecules can also visualize alternations in protein or cellular functions including metabolism, hypoxia, enzyme activity, and protein synthesis [6]. One clinical example is fluorodeoxyglucose (FDG) that targets increased glucose metabolism found commonly in cancer cells. 18F-FDG has been the most commonly used PET molecular probe for penile, bladder, prostate, and kidney cancer [12]. On the other hand, due to the small size, there are very limited numbers of signaling conjugates, and small molecules can be attached without altering their pharmacokinetic and targeting properties [6].
Peptides (~15 amino acids) are intermediate in size between small molecules and mAb and engineered proteins. Thus, peptides provide increased flexibility in signaling conjugates and generally offer superior selectivity and specificity than small molecules. While peptides generally have lower affinity compared to mAbs, the relatively smaller size makes them less immunogenic and allows penetration to deep tissue where mAbs are not accessible. The biggest limitation of peptide as targeting molecule is the in vivo stability, and strategies such as adding prosthetic group is mostly required for radiolabeling of peptides. An example of peptide-based molecular imaging agent for SPECT is 111In-DTPA-octreotide, a somatostatin analog originally developed for scintigraphy of neuroendocrine tumors. More peptide imaging agents are under clinical trial or preclinical investigations [13]. One preclinical example of peptide-based molecular imaging is bombesin (BBN) analogs targeting the gastrin-releasing peptide receptor (GRPR) as molecular probes for PET imaging of prostate cancer. In vivo imaging results on animal models are promising [14].
Monoclonal antibodies can recognize tumor-associated antigens including adhesion molecules and cell surface proteins with ultrahigh affinity and specificity [15]. However, their utilization as molecular imaging agents are limited by high immunogenicity from murine origin, restricted tissue penetration, and slow clearance from blood associated with big size (150 kDa) [6, 16]. The immunogenicity issue can be overcome by production of chimeric and humanized mAb [15]. mAb derivatives with smaller size have also been developed to improve the pharmacokinetics of mAb while maintaining the specificity and affinity [17]. While single-chain variable fragment (scFv, ~25 kDa), which contains one antigen-binding site of mAb, clears too quickly to generate adequate accumulation on target, mAb derivatives with intermediate size such as diabodies (scFc dimer, ~55 kDa) and minibodies (scFv fused to single Fc domain, ~80 kDa) have shown promising imaging results on animal models [17]. Currently, there are at least 8 mAbs approved for SPECT molecular imaging [16]. Among them, 111In-capromabpendetide (ProstaScint) in particular is approved for molecular imaging of prostate cancer [18].
Affibodies are emerging molecular imaging agents with significant potential for clinical translation [6]. Affibodies are small non-immunoglobulin affinity ligands capable of binding to a wide range of protein targets. They are selected from combinatorial libraries based on a 58-amino acid, three-alpha-helical Z-domain scaffold [17]. Affibodies have fast clearance from the blood with adequate tumor uptake [16]. There are growing interests in using affibodies as alternative to mAb and mAb derivatives. One example is affibody molecules that target epidermal growth factor receptor 2 as molecular probe for SPECT. A clinical study on breast cancer patients with 111In- or 68Ga-labeled ABY-002 has shown great potential to localize metastatic lesions [19]. Variants of ABY-002 also have been investigated on prostate cancer xenografts [20].
Aptamers are single-stranded DNA or RNA oligonucleotides that are activated upon binding to their targets. While aptamers offer high affinity and specificity comparable to mAbs, their application as molecular imaging agent is limited by low in vivo stability and short half-life associated with their small size [6]. Molecular imaging with aptamers in general is still in its infancy and needs further investigation [6]. Recently, a novel nucleolin-targeted DNA aptamer (AS1411) has been evaluated as therapeutic drug for metastatic renal cell carcinoma in a phase II trial study [21]. Low toxicity was observed in patients, indicating AS1411 may potentially be translated for molecular imaging purpose.
Targeting molecules can be conjugated to radioisotopes, fluorescent dyes, or nanoparticles to enable visualization. Molecular imaging with targeted nanoparticles is emerging as an exciting diagnostic tool. Nanoparticles can vary largely in size, shape, and material they can be composed of, with unique surface properties and reactivates [6, 22]. The large variety offers nanoparticles great flexibility in terms of imaging techniques they are compatible with, thus enabling multimodality molecular imaging [6, 22]. Nanoparticles are designed to be intrinsically near-infrared fluorescent [e.g., single-wall carbon nanotubes (SWNTs), quantum dots (Qdots)], or have magnetic properties [e.g., superparamagnetic iron oxide nanoparticles (SPIOs)] [23]. By surface modification [24] or additional radiolabeling [25], dual-labeled nanoparticles targeting the same ligands can therefore be recognized simultaneously by two or even three imaging techniques. In general, different imaging techniques are complementary rather than competitive [23], and the multimodality capacity of nanoparticles will facilitate the cross-talk between the whole-body scan imaging techniques (e.g., PET, CT) and optical imaging techniques (e.g., photoacoustic imaging, Raman spectroscopy), opening up the opportunity of tumor staging and characterization with single-molecular imaging agent.
The flexibility of nanoparticles also enables the capacity for multiplexed imaging. Many nanoparticles [e.g., quantum dots (Qdots) and surface-enhanced Raman scattering (SERS) gold nanoparticles] are tunable for different emission wavelength or Raman spectrum by alternation of size or structure. Same type of nanoparticles with different characteristics can be functionalized for simultaneous imaging of different tumor targets. In vivo studies on small animals have demonstrated simultaneous detection and separation of ten different types of SERS particles [26]. The Raman spectroscopy feature for detecting SERS nanoparticles has been incorporated into endoscopy [27], and molecular imaging of bladder cancer with targeted SERS particles is currently under active investigation.
The large surface area-to-volume ratio of many nanoparticles allows substantial payloads of targeting molecules, contributing to their ultrahigh sensitivity [6, 28], thereby minimizing the quantity of the imaging agent required and enabling molecular imaging with insensitive conventional imaging techniques such as CT [29] and MRI [30]. Nanocrystals such as Qdots offer additional benefits including 20-fold greater brightness and 100-fold greater stability to photo-bleaching than organic fluorophores [31], which is ideal for optical imaging.
While promising, in vivo application of nanoparticles can be challenging due to general pharmacokinetic issues associated with their large size (10 ~ 200 nm). While toxicity can also be an issue for nanoparticles such as Qdots with a core made of cadmium, Qdot-based molecular imaging may still have a role in evaluation of urogenital malignancies through topical (e.g., intravesical) administration, which could potentially minimize the toxicity and other limitations from systematic use. On the other hand, gold nanoparticles (AuNP) could be a safer alternative and have been approved for therapeutic use in humans [32]. Efficacy of therapeutic gold nanoparticles has also been demonstrated for treatment of prostate cancer on mouse xenograft [33], accelerating the clinical translation of molecular imaging of urological malignancies with targeted nanoparticles.
Molecular Imaging with Conventional Imaging Modalities
Current investigations of molecular imaging platforms incorporating conventional CT and MRI have emerged in recent years, which promise to add molecular information such as with PET and SPECT. PET and SPECT are radionuclide imaging techniques that trace whole-body distribution of intravenously administrated radiolabeled imaging agents [6], delivering excellent sensitivity along with unlimited depth of penetration and quantitative capability. As biochemical changes often precede anatomical ones in disease, PET and SPECT are able to detect such changes before CT and MRI. A limitation of PET and SPECT, however, is the lack of an anatomical reference frame (addressed by combining with CT/MRI). On the other hand, US is efficient in detecting vascular structures with high resolution and sensitivity [34], with transrectal US being the current standard for real-time guidance of prostate biopsy [35]. While it is relatively cost-effective and has a good safety profile, it is limited in its ability to image bone or air-containing structures and by its limited depth of penetration [6].
CT and MRI
Currently, CT and MRI rely on iodinated and gadolinium (Gd)-based nonspecific imaging agents to provide tissue contrast [6]. While routinely used in detection of urologic malignancies [35], the application of CT and MRI in molecular imaging is constrained by low sensitivity since large amounts of imaging agents are needed to produce an adequate readout. These considerations are clinically relevant given the risks of radiation exposure and contrast nephropathy for CT scan and nephrogenic systemic fibrosis for MRI. However, multiple examples of CT and MRI molecular imaging have begun to emerge recently. Gastrin-release peptide (GRP) fragments targeting GRPR have been chelated to Gd for in vivo MRI of prostate cancer [30, 36]. GRPR, a member of the BBN receptor family, is consistently overexpressed in prostate cancer [37] but absent or very low on normal and hyperplastic prostate tissues [38].
A AuNP dual loaded with RNA aptamer targeting prostate-specific membrane antigen (PSMA) and doxorubicin has shown preferential binding to PSMA-expressing prostate cancer cells in vitro by CT scan [39]. PSMA is a type II transmembrane glycoprotein with folate hydrolase activity [40, 41] whose expression in normal tissues is highly restricted to prostate epithelium [42–44]. Given its high-level expression in all prostate cancers [43–47], PSMA has been a well-established biomarker for prostate cancer diagnosis and immunotherapy [48].
US
In recent years, an intravascular contrast agent using gas-filled microbubbles coated with lipids or biopolymers has been studied for the imaging of cancer-associated neovascularity indicative of angiogenesis. This contrast-based US technique has shown significant benefits for detecting high-grade/high-volume prostate cancer in clinical trials [49–51] and is under active investigation for diagnosis of renal masses [52–54]. The microbubbles have exhibited an acceptable safety profile and can be attached with disease-specific targeting molecules, thus adding a molecular imaging dimension to ultrasound [55, 56]. While most of molecular imaging studies with contrast-enhanced US are under preclinical development [56–60], a phase 0 clinical trial completed in 2012 in prostate cancer patient for microbubbles (BR55) targeting human vascular epidermal growth factor receptor 2 (VEGFR2) (Available online: http://www.clinicaltrials.gov/ct2/show/NCT01253213).
PET/SPECT
A panel of clinical-grade, molecular imaging agents has been clinically used with PET to image prostate cancer, among them 18F-FDG (glucose metabolism), 11C-acetate, 18F-fluoroacetate, 11C/18F-choline (lipogenesis), anti-1-amino-3-18F-fluorocyclobutane-1-carboxylic acid (anti-18F-FACBC) and 11C-methionine (amino acid transportation), 18F-fluoro-5a-dihydrotestosterone (18F-FDHT) (androgen receptor), and 64Cu-CB-TE2A-AR06 (GRPR) [12, 61–63].
While 18F-FDG has been most commonly used in imaging urological malignancies by targeting increased glucose metabolism in cancer cells, FDG PET/CT has limited applicability in detecting primary prostate cancer and local recurrence due to the generally low avidity of FDG for primary prostate cancer, uptake in cases of prostatitis and benign prostatic hypertrophy, and interference from nearby bladder excretion [12, 61, 62]. However, FDG uptake has been shown to be higher in untreated patients with advanced-stage prostate cancer [64] and aggressive poorly differentiated tumors than in well-differentiated tumors [65]. Cumulative evidences have shown FDG PET/CT may be useful in the subset of patients with greater than intermediate risk (Gleason sum score ≥7), increased serum prostate surface antigen (PSA) level at initial diagnosis [66], and restaging patients with PSA relapse after treatment for localized prostate cancer [67–69]. The most useful application of FDG PET/CT may be providing prognostic information in patients with castration-sensitive and castration-resistant metastatic disease [12, 64, 70, 71].
In addition, by using a diuretic followed by oral hydration, this strategy may facilitate the FDG PET/CT detection of recurrent or residual bladder lesions for invasive bladder cancer [72]. Overall, FDG PET/CT may indeed have a major impact on the clinical management of up to 41 % of prostate cancer patients, 33 % with kidney cancer patients, and 36 % with bladder cancer patients older than 65 years [73, 74].
111In-capromab pendetide (ProstaScint®) is the only approved radioimmunoscintigraphy agent for molecular imaging of prostate cancer. ProstaScint consists of a radiolabeled murine mAb (7E11-C5.3) to PSMA. The clinical utilities of ProstaScint scan with SPECT include: (1) detecting occult lymph node metastasis in patients with high PSA level prior to treatment [75, 76], (2) identifying early recurrence after treatment [77, 78], and (3) stratifying patients for salvage radiation therapy [79, 80]. While the recent technical advance with fusion of SPECT with CT/MRI has revolutionized ProstaScint scan with doubled diagnostic accuracy and reduced false-positive rate (Fig. 20.2) [80], ProstaScint has limited use in detecting bone metastases, and the intracellular epitope recognition of ProstaScint also remains controversial. ProstaScint binds to intracellular epitope of the transmembrane PSMA protein, raising concerns of its limited accumulation in viable cells [12]. Another mAb (J591) against PSMA that recognizes extracellular epitope of PSMA has been developed and studied in phase II trial for imaging metastatic castration-resistant prostate cancer [81]. Also, two novel small molecule PSMA inhibitors (MIP-1095 and MIP-1072) have been studied in phase I trial, in which the 123I-labeled compounds localized to metastatic lesions in both soft tissue and bone at as early as 1–4 h after intravenous administration [82]. A multifunctional nanoparticle functionalized with bladder cancer-specific ligand (PLZ4) also demonstrated both fluorescent imaging and therapeutic potential in mouse orthotopic model of dog bladder cancer [83]. Their clinical utility requires further validation in larger clinical studies.
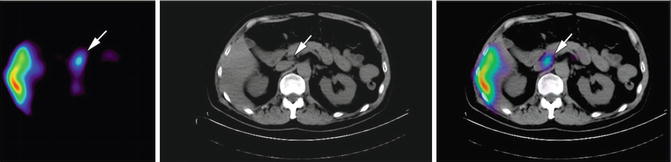
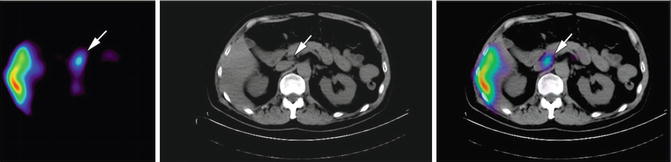
Fig. 20.2
SPECT/CT case of showing a 1.5 × 0.7 cm peripancreatic lymph node with correlative 111In-capromab pendetide uptake. Images of SPECT alone (left); CT alone (middle); SPECT/CT fusion (right). Arrows indicate where the lymph node uptake was identified (From Aparici et al. [131], with permission from e-Century Publishing Corporation)
Molecular Optical Imaging
Optical imaging utilizes visible, ultraviolet, and infrared light to interrogate tissues of interest, with its advantages including superior resolution and relatively facile integration into the operating room setting. By integrating features such as fluorescence imaging into standard white-light endoscopic and laparoscopic procedures, the visual contrast between normal/benign and tumor tissues is enhanced, enabling real-time, intraoperative visualization and characterization of tumor tissues [84]. The advent of fluorescence-guided surgery has been a novel paradigm for cancer diagnostics and treatment [9].
Several promising optical imaging modalities have entered the urologic clinical arena, ranging on a macroscopic [i.e., photodynamic diagnosis (PDD), near-infrared fluorescence (NIRF)] to microscopic [i.e., optical coherence tomography (OCT), confocal laser endomicroscopy (CLE)] scale. PDD, NIRF, and CLE all require an exogenous fluorescent imaging agent. The potential in-human application of fluorescent molecular imaging has been presented in ovarian cancer [85] and colon cancer [86], adding promise for future application to urologic malignancies.
PDD
PDD (Fig. 20.3) is considered a molecular imaging modality by detecting increased metabolism of photosensitive protoporphyrin analogs [5-aminolevulinic acid (5-ALA), or its more strongly fluorescent ester analog, hexaminolevulinate (HAL)] in tumor cells. PDD provides macroscopic examination of tissues by inducing pink-colored fluorescence from selective accumulation of 5-ALA or HLA using a blue light source (375 ~ 440 nm). PDD has been applied principally in bladder cancer through intravesical contrast administration under an integrated blue fluorescence cystoscope setting, with more limited experience in penile, prostate, and kidney cancers. This is in part due to the limited penetration depth of light [34] and established bladder accessibility with intravesical endoscopy [5]. Nevertheless, PDD with 5-ALA has been used to guide Nd:YAG laser coagulation in penile surgery for carcinoma [87], to assess margin status in laparoscopic partial nephrectomy [88], and to assess margin status during prostatectomy [89, 90]. For bladder cancer, PDD has a potential role in addressing white-light cystoscopy (WLC)’s shortcomings with incomplete resection of suspicious lesions that can subsequently lead to tumor recurrence/progression [3]. In human studies, PDD has been shown to improve initial detection of carcinoma in situ (CIS) compared to WLC [91–93].
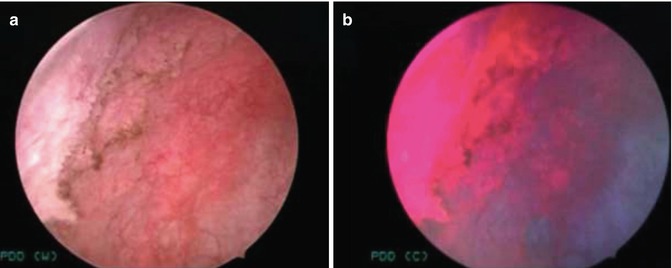
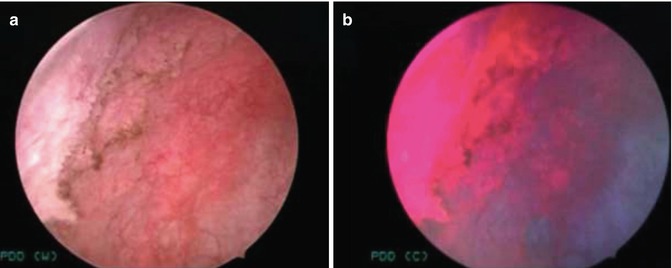
Fig. 20.3
PDD for bladder cancer. WLC (a) of the right lateral bladder wall demonstrated a diffuse area of nonpapillary tumor. Under PDD (b), pink fluorescence outlines the extent of the neoplastic region that was pathologically confirmed to be carcinoma in situ (CIS) (From Hsu et al. [24], with permission from Wolters Kluwer Health)
Despite the above benefits, PDD still suffers from up to 30 % false-positive rate, particularly in patients with prior bacillus Calmette-Guérin (BCG) treatment, the standard immunotherapy for bladder cancer [3]. Preliminary studies are ongoing with combining the PDD concept with molecular imaging agents targeting tumor-specific antigens. CD47, for example, is a marker that is widely distributed in various malignancies including bladder cancer. Antibodies to this marker have demonstrated therapeutic effect for bladder cancer in mouse xenograft [94] and may be used for molecular imaging. This provides potential in improving specificity using molecular markers to differentiate cancerous lesions as opposed to relying on metabolic differences.
NIRF
NIRF has revolutionized optical imaging by providing extended depth of penetration and minimal tissue autofluorescence at near-infrared spectrum (800 ~ 2,500 nm) [95]. As NIRF imaging systems used in conjunction with open, laparoscopic, and robotic-assisted surgery have been developed, NIRF has been used with non-tumor-specific NIRF dye [indocyanine green (ICG)] and nanoparticle (99mTc-nanocolloid) to facilitate intraoperative imaging during nephrectomy to locate the hilar vessels [96, 97] and robotic prostatectomy to highlight the sentinel lymph nodes draining the prostate [98].
Molecular imaging of prostate cancer with NIRF is currently under active preclinical investigation. An Alexa Fluor 680-conjugated bombesin (BBN) peptide targeting BBN receptors has shown 90 % accuracy in detecting metastatic prostate cancer [99]. To facilitate clinical translation, BBN peptide [100] and epithelial cell adhesion molecule (EpCAM) mAb [101] were coupled with IRDye®800CW (IR800CW), a NIRF dye with good safety profile [102]. Recently, multimodality and quantitative molecular imaging with NIRF have been demonstrated to improve prostate cancer detection and resection. Monoclonal antibody against PSMA has been dual labeled with 111In and IR800CW to enable SPECT/CT and NIFR imaging, with SPECT/CT for preoperative tumor detection and NIFR for surgery guidance [103]. A panel of EpCAM mAbs dual labeled with 64Cu and IR800CW was also evaluated by PET/CT and NIRF to enhance the sensitivity and specificity of lymph nodes metastases detection [104].
CLE
CLE allows for real-time in vivo microscopy with cellular level resolution using a miniaturized fiberoptic imaging probe with a 488 nm laser and nonspecific fluorescein as the imaging agent. The clinical system (Cellvizio, Mauna Kea Technologies) uses probes ranging from 0.85 to 2.6 mm in diameter, which fit through working channels of standard cystoscopes. In vivo application of CLE with fluorescein in urological operations has been reported in the lower urinary tract, particularly with bladder cancer [105–108]. While visualization of benign versus low- and high-grade tumors with respect to cellular organization and vascularization were shown, diagnosis with CLE is largely based on cell architecture and morphology, making real-time analysis of confocal images challenging in the operating room.
A fluorescein isothiocyanate (FITC)-labeled bladder cancer-specific molecular imaging agent may improve the clinical application of CLE. FITC has a well-approved safety profile and is capable of protein conjugation. The signaling molecule may be adapted from clinically approved therapeutic mAb or peptides to facilitate clinical translation. CLE molecular imaging agent for bladder cancer is currently under investigation. On the other hand, in-human application of targeted imaging with CLE has been demonstrated for other epithelial diseases including colonic dysplasia [109] and esophageal neoplasia [86] using FITC-labeled peptide, confirming the feasibility of molecular imaging with CLE. Future investigation may allow combined molecular imaging of CLE with macroscopic endoscopic technique such as PDD to enable simultaneous tumor detection and characterization.
Emerging Molecular Imaging Modalities
Several emerging molecular imaging modalities [i.e., photoacoustic imaging (PAI), Raman spectroscopy (RS), and multiphoton microscopy (MPM)] hold great promise in preclinical studies. PAI detects ultrasound transmission resulting from optical absorption by tissues. Imaging agents such as SWNT and AuNP emit strong photoacoustic signals via light absorption and can be used to enhance tissue contrast or functionalized with signaling molecules to enable molecular imaging with PAI [110, 111]. The advantages of PAI include superior depth of penetration (up to 5 cm) compared to optical imaging, significantly lower doses of imaging agent required (picograms to micrograms), and minimal background noise compared to traditional US [6]. The urologic application of PAI has been explored, with preliminary results of an ex vivo study on human prostate tissues suggesting that PAI can differentiate between normal, benign, and malignant tissues by detecting the intrinsic differences in photoacoustic signals [112]. In vivo visualization of prostate tumors has been shown in mouse models [113, 114], with a transrectal probe combining US and PAI under development to facilitate image-guided prostate biopsy. In a pilot study on ex vivo porcine bladders, PAI successfully visualized simulated tumors of injected biomaterials containing varying degrees of pigment, suggesting PAI can potentially visualize tumor depth in the bladder and provide complimentary staging information to diagnostic cystoscopies [115]. Photoacoustic endoscopy has been developed recently [116, 117], and PAI platforms contrasted with non-targeted non-ionizing imaging agents have been investigated for in vivo visualization of the bladder in small animals [27, 118, 119] to facilitate clinical translation. While PAI molecular imaging agents for urological malignancies are still under investigation, the feasibility of molecular imaging with PAI has been demonstrated in tumor-bearing mice for identifying breast cancer lesions using SWNT functionalized with Arg-Gly-Asp (RGD) peptide targeting tumor overexpressed integrin [120].
RS detects alternation in vibrational state of molecules in biological tissues under near-infrared light illumination (785–845 nm), which is known as a Raman shift [121]. Detection of multiple Raman peaks from the target tissue is plotted to create a spectrum of peaks, achieving a molecular “fingerprint” of the tissue examined without the need for exogenous contrast agent [121, 122]. RS’s drawbacks include time to obtain a spectrum (1–5 s), weak signals, and limited field of view. SERS gold nanoparticles have been demonstrated to augment relatively weak signals from Raman scattering to improve sensitivity. These nanoparticles, when conjugated with tumor-specific ligands, have been used for in vivo tumor detection in small animals [123]. Targeted SERS particles also have the potential to enable multiplexed targeted imaging during endoscopy [124]. The feasibility of in vivo bladder cancer diagnosis was demonstrated using a fiberoptic Raman endoscopic probe that could differentiate cancerous tissue from normal urothelium in near real time during cystoscopy [122]. Bladder cancer-specific SERS particles are currently under investigation. On the other hand, RS has also been considered for implications in prostate cancer with pathologic diagnosis, margin status, and diagnostic biomarkers [125].
MPM uses simultaneous absorption of 2–3 near-infrared photon to cause nonlinear excitation equivalent to that created by a single photon of blue light and allows for both in vivo imaging and ex vivo imaging of fresh, unprocessed, and unstained tissue via distinct intrinsic tissue emissions (ITEs). Imaging capabilities include submicron resolution and up to 0.5 mm depth of penetration. Urologic investigations using MPM have been done with periprostatic nerves in a rat model [126], visualization and quantification of renal uptake [127, 128], differentiating normal and pathologic human bladder biopsies [129], and testicular biopsies distinguishing normal from abnormal spermatogenesis in patients being worked up for nonobstructive azoospermia [130].
< div class='tao-gold-member'>
Only gold members can continue reading. Log In or Register a > to continue
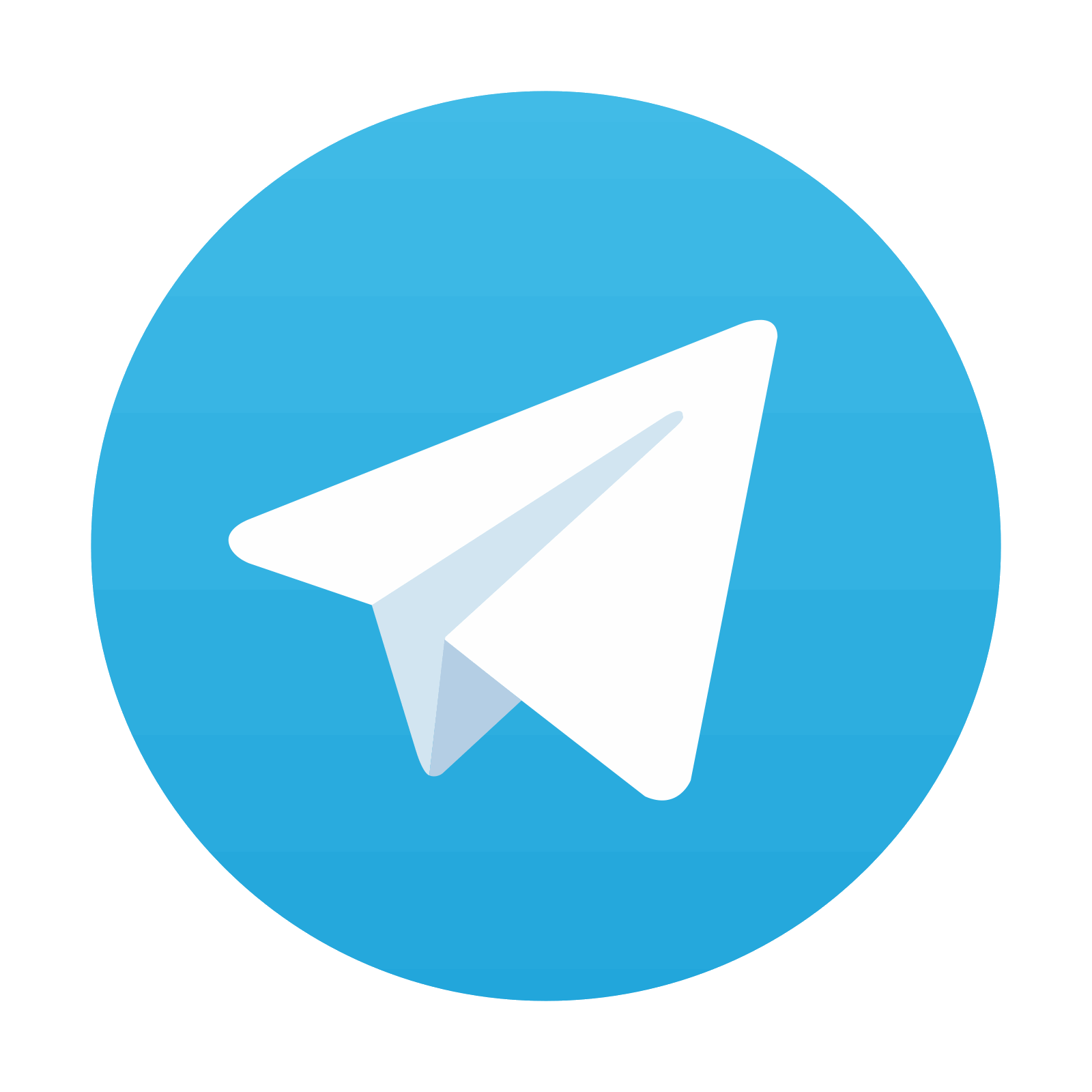
Stay updated, free articles. Join our Telegram channel
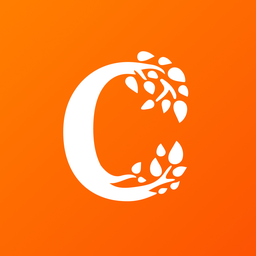
Full access? Get Clinical Tree
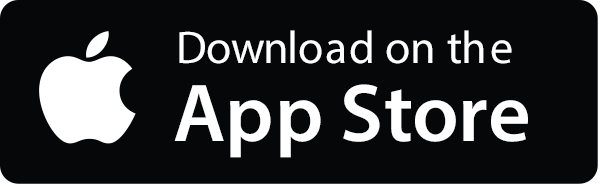
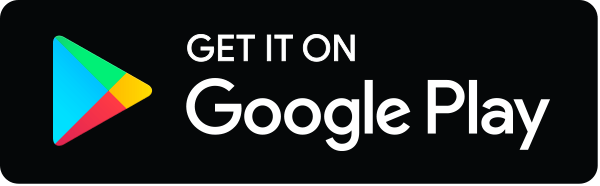