© Springer International Publishing AG Switzerland 2015
Luca Aldrighetti, Francesco Cetta and Gianfranco Ferla (eds.)Benign Tumors of the Liver10.1007/978-3-319-12985-3_22. Molecular Biology and Genetics
(1)
IRCCS MultiMedica, Milan, Italy
Hepatocellular benign tumors are characterized by benign proliferation of hepatocytes with a mass-forming presentation and mainly consist of hepatocellular adenoma (HA) and focal nodular hyperplasia (FNH) [1, 2]. HA and FNH share common features. First, a large female preponderance has been found in both diseases, with a female/male ratio = 8:1 for FNH and 9:1 for HA. In addition, FNH and HA derive from the proliferation of mature hepatocytes and arise on an otherwise normal liver. Therefore, they represent a model to study liver tumorigenesis outside the classic background of cirrhosis [2].
Has have been classified into four major molecular subgroups according to their genetic and phenotype characteristics [2–8].
2.1 HNF1A-Mutated Adenomas
This group includes 30–40 % of HAs and is defined by HNF1A (coding for hepatocyte nuclear factor 1 alpha) mutations. In most of cases, both inactivating HNF1A mutations are somatic and are thus found only in tumoral cells. However, some patients carry a germline inherited HNF1A mutation in one allele. In these cases, there is an association with maturity-onset diabetes type 3 (MODY3), a monogenic non-insulin-dependent diabetes. Adenomas that develop in patients with MODY3 have another somatic mutation inactivating the second allele of HNF1A in the tumor hepatocytes according to the 2-hit model described by Knudson for inactivation of tumor suppressor genes. In particular, patients with germline mutations of HNF1A are predisposed to develop liver adenomatosis. Genetic studies performed in mouse models suggest a necessary cooperation between HNF1A inactivation and additional genetic alterations to promote liver tumorigenesis. However, until now in humans, no additional genetic alterations have thus far been identified in HNF1A-mutated adenomas (H-HAs), and biallelic inactivating mutations of HNF1A are exclusive of CTNNB1, IL6ST, GNAS, and STAT3 mutations classically found in other HCA subtypes [2–8].
HNF1A belongs to the hepatocyte nuclear factor family and is a key transcription factor involved in liver development. In addition, HNF1A controls hepatocyte differentiation as well as glucose and lipid metabolism. Transcriptomic analysis of H-HAs revealed the dysregulation of genes involved in glucose and lipid metabolism. The main features observed included repression of gluconeogenesis, activation of glycolysis, and stimulation of aberrant fatty acid synthesis. Moreover, in human H-HA, several oncogenic factors lead to mTOR activation, angiogenesis, cell cycle activation (CCND1), and cell proliferation (PDGFRA and B, ERRB2). Interestingly, these observations were also observed on HNF1A silencing in different hepatocellular cell lines, thereby showing the specific role of HNF1A inactivation in inducing the metabolic and pro-proliferative disorders observed in H-HA [2–8].
Genotype/phenotype correlations further support these observations. Indeed, pathological analysis showed that inactivating biallelic mutations of HNF1A define a very homogeneous subgroup of adenomas characterized by a marked steatosis. However, steatosis alone is insufficient to diagnose H-HA. In fact, it can be detected in more than 35 % of inflammatory and unclassified HAs. Expression of liver fatty acid-binding protein (LFABP) is specifically downregulated in H-HAs. LFABP staining has been included in the panel of immunohistological markers used to classify H-HAs (with 100 % specificity and 100 % sensitivity). Finally, HNF1A can be considered at the crossroads between metabolism and tumorigenesis in the liver [2].
2.2 Beta-Catenin-Activated Adenomas
Mutations of beta-catenin are localized at hot spots in exon 3 of the CTNNB gene. Beta-catenin-mutated HAs constitute approximately 10–15 % of all HAs. Interestingly, beta-catenin-activated mutations are exclusive of HNF1A mutations but can be combined with gp130 or GNAS mutations, and half of the beta-catenin-activated adenomas (bHAs) are also inflammatory (bIHA) [2].
Under physiological conditions, in the absence of activating ligand, the Wnt/beta-catenin pathway is inactivated, and beta-catenin is phosphorylated by the GSKB/APC/AXIN complex. Phosphorylation of beta-catenin leads to its ubiquitination and subsequent degradation by the proteasome. In tumors, mutations of beta-catenin impair phosphorylation by the GSK3B/APC/AXIN complex and decrease its degradation; as a consequence, beta-catenin accumulates and translocates to the nucleus to act as a co-transcription factor via the association with the T-cell factor/lymphoid-enhancing complex. The Wnt/beta-catenin pathway plays a key role in amino acid metabolism, cell–cell interactions, liver regeneration, and zonation [2, 9].
Wnt is also a well-known oncogenic pathway involved in several types of cancer, namely, HCC. However, a genetically engineered mouse model harboring liver-specific expression of beta-catenin-activating mutations developed neither HA nor HCC. On the contrary, liver-specific deletion of the APC gene or co-expression of HRAS with CTNNB1 mutant leads to formation of HCC. These results show that activation of beta-catenin is not sufficient alone to induce liver carcinogenesis but can cooperate with other pathways to promote tumor development.
Genotype/phenotype correlation revealed striking hallmarks of beta-catenin-mutated HAs. First, few male subjects develop Has. However, when it occurs, it is usually a bHA. At the pathological level, these adenomas exhibit cholestasis and cell dysplasia. Importantly, beta-catenin mutations are associated with a high risk of malignant transformation. Beta-catenin-mutated adenomas show strong overexpression of GLUL (coding for glutamine synthase) and LGR5 (coding for leucine-rich repeat-containing G-protein-coupled receptor 5), which are 2 beta-catenin target genes [2].
By immunohistochemistry, diagnosis of a beta-catenin-mutated adenoma is performed using beta-catenin and glutamine synthase immunostaining. bHAs are characterized by a nuclear localization of beta-catenin and a strong homogeneous expression of glutamine synthase in the cytoplasm. This pattern is homogeneous and diffuses in bHAs and differs from the “maplike pattern” observed in FNH. Due to difficult interpretation, because beta-catenin is detectable only in a few nuclei, analysis of beta-catenin expression has to be combined with glutamine synthase staining to increase detection sensitivity for beta-catenin activation. However, although these two markers have very good specificity (approximately 100 %), they show insufficient sensitivity (75–85 %) for the diagnosis of beta-catenin mutations. Because of the high risk of malignant transformation, HAs without overexpression of beta-catenin target genes and not mutated for HNF1A (mutually exclusive with beta-catenin mutations) should be screened for beta-catenin mutations [2, 8].
2.3 Inflammatory Adenomas
The third group of HAs is composed of inflammatory adenomas and accounts for 40–50 % of all adenomas. The peculiar feature of IHAs is the activation of the JAK/STAT pathway.
At the histologic level, IHAs are characterized by polymorphic inflammatory infiltrates, dystrophic vessels, and sinusoidal dilatations. They also exhibit overexpression of serum amyloid A (SAA) and C-reactive protein, 2 proteins of the acute phase of inflammation.
Identification of IHAs reveals obesity and high alcohol intake as two new risk factors for occurrence of inflammatory adenomas but not for the other subtypes. Importantly, a subset of IHAs also harbors an activating mutation of beta-catenin and, consequently, shows an increased risk of malignant transformation [2, 11–13]. Recently, three different molecular drivers, namely, IL6ST (coding for gp130), STAT3, and GNAS, were discovered as oncogenes activated by mutations in IHCA. In tumors, each mutation is exclusive of the two others, and the three genes (IL6ST, STAT3, and GNAS) can explain more than 75 % of the overall IHAs.
In the canonical IL-6/JAK/STAT pathway, interleukin (IL)-6 binds to gp80, the IL-6 receptor (IL6R) that dimerizes with gp130, the co-receptor signal transducer (IL6ST); then, 2 IL-6/gp130/gp80 complexes associate and form an hexameric complex at the cell membrane88. This leads to the recruitment of JAK1 and JAK2, which phosphorylate in turn STAT3 and STAT1. Activated phospho-STAT3 and phospho-STAT1 homodimerize and/or heterodimerize and translocate to the nucleus to act as transcription factors. Somatic heterozygous mutations activating gp130 are found in 65 % of inflammatory adenomas. Mutations of gp130 are mainly small in-frame deletions targeting the IL-6 binding site. Gp130 mutant leads to gp130 dimerization and constitutive STAT3 activation in the absence of IL-6. Rare cases of HCC also harbor gp130-activating mutations. Interestingly, these HCCs develop on normal livers and also show beta-catenin-activating mutations, suggesting malignant transformation of HCAs [2].
The second oncogene mutated in IHCA, STAT3, is mutated in 5 % of IHAs. These mutations lead to amino acid substitutions mainly localized in the SH2 domain involved in STAT3 dimerization. STAT3 mutants are phosphorylated and activated in the absence of IL-6 and lead to an uncontrolled constitutive activation of the inflammatory pathway. The third gene, GNAS, is also found to be mutated in 5 % of IHAs. GNAS encodes the alpha subunit of the Gs protein. The Gs protein complex is composed of the alpha and beta/gamma subunits. In their inactive forms, alpha and beta/gamma subunits are associated. When a stimulus leads to G-protein-coupled receptor activation, guanosine triphosphate replaces guanosine diphosphate on the subunit. Then, the latter dissociates and activates the adenylate cyclase, thereby increasing levels of intracellular cyclic adenosine monophosphate, which acts as an intracellular second messenger. Thus, the alpha subunit is inactivated due to an intrinsic guanosine triphosphatase activity that converts guanosine triphosphate into guanosine diphosphate [2].
Activating mutations of GNAS localize at hot spots in the guanosine triphosphatase domain. Guanosine triphosphatase activity of the subunit is consequently impaired and leads to aberrant activation of adenylate cyclase and accumulation of cyclic adenosine monophosphate. GNAS is considered an oncogene recurrently mutated in benign lesions such as pituitary and thyroid adenomas, myelodysplastic syndrome, and intraductal papillary mucinous neoplasm but also in cancers such as cholangiocarcinoma. Typical GNAS-activating mutations have been identified in sporadic HCAs and in adenomas arising in patients with McCune–Albright syndrome. McCune–Albright syndrome is a rare disease defined by “café au lait” skin macula, fibrous dysplasia of bone, and high risk of pituitary and thyroid adenomas. McCune–Albright syndrome is caused by somatic mosaic-activating mutations of GNAS genes and is a condition that promotes occurrence of HCAs. GNAS-activating mutations lead to mild STAT3 activation in hepatocellular cell lines, which underlines a cross talk between the cyclic adenosine monophosphate and JAK/STAT pathways. Finally, in approximately 25 % of IHAs, it was not possible to identify any gene defect that could explain the phenotype [2].
This suggests that other driver genes leading to JAK/STAT3 activation remain to be identified. Mutations in IL6ST/gp130, STAT3, or GNAS act by a similar mechanism called “oncogene-induced inflammation” that leads to constitutive STAT3 activation. Uncontrolled activation of the JAK/STAT pathway in tumor hepatocytes explains the inflammatory phenotype observed at both the histologic and clinical levels. Cytokine and chemokine induced by STAT3 and secreted by hepatocytes act as chemoattractants that promote tumor infiltration by lymphocytes. The origin of dystrophic artery and sinusoidal dilatation observed in the tumor is unknown, but a direct or indirect role of the release of inflammatory proteins by the mutated hepatocytes could be hypothesized.
All of these data highlight that hepatocytes are major inflammatory cells and that inflammation is a central mechanism of liver tumorigenesis. Animal models would be very useful to dissect the roles of the inflammatory pathway in benign tumorigenesis, to understand the cooperation between the IL-6/JAK/STAT and beta-catenin pathways in malignant transformation, and to test for new biotherapies. However, liver-specific animal models are lacking [2].
Unclassified adenomas include approximately 10 % of all HCAs. Different genetic and environmental factors can predispose to development of HCAs. First, several rare genetic syndromes are strongly associated with the occurrence of adenomas. Some patients with MODY3 (monoallelic germline mutations of HNF1A) develop liver adenomatosis with biallelic H-HCA. Following this mechanism, HNF1A meets the criteria of a classic tumor suppressor gene with a transmitted predisposition to develop tumors as defined by Knudson. To our knowledge, currently all familial transmission of liver adenomatosis identified worldwide is associated with germline HNF1A mutations. In these patients, familial genetic counseling is required to propose screening for diabetes and HCA [2].
However, liver adenomatosis could also be related to other molecular subtypes. Other genetic disorders associated with the occurrence of adenomas are glycogen storage diseases and particularly type 1a glycogenosis. Glycogenosis 1a is a rare recessive metabolic disease characterized by germline mutations inactivating glucose-6-phosphatase [14–16]. More than 50 % of these patients develop HAs during follow-up [14–16]. As a mirror of the human disease, mice with a liver-specific deletion of glucose-6-phosphatase harbored the same metabolic disorder and developed HCA. A recent study showed that gene therapy using adenovirus could correct the metabolic disorder and prevent development of HCA in this mouse model. Two studies have recently shown that all HAs observed in patients with glycogenosis were bHCAs, IHCAs, or unclassified, whereas no HNF1A mutations were identified. Furthermore, metabolic disorders induced by HNF1A and G6PC inactivation appear to be very similar, at least partly explaining the exclusive occurrence of these genetic defects in patients. Finally, McCune–Albright syndrome, an orphan disease characterized by mosaic somatic GNAS-activating mutations, predisposes patients to the development of IHA. Strikingly, these genetic diseases predispose to the development of specific molecular subtypes: H-HA in patients with MODY3 [10], IHA in patients with McCune–Albright syndrome, and absence of H-HA in glycogenosis [14–17]. However, whereas genetic diseases constitute strong risk factors, penetrance of the HCA phenotype is incomplete and particularly rare in patients with MODY3. Thus, additional modifier genes or environmental factors remain to be identified to fully understand which patients will develop HA.
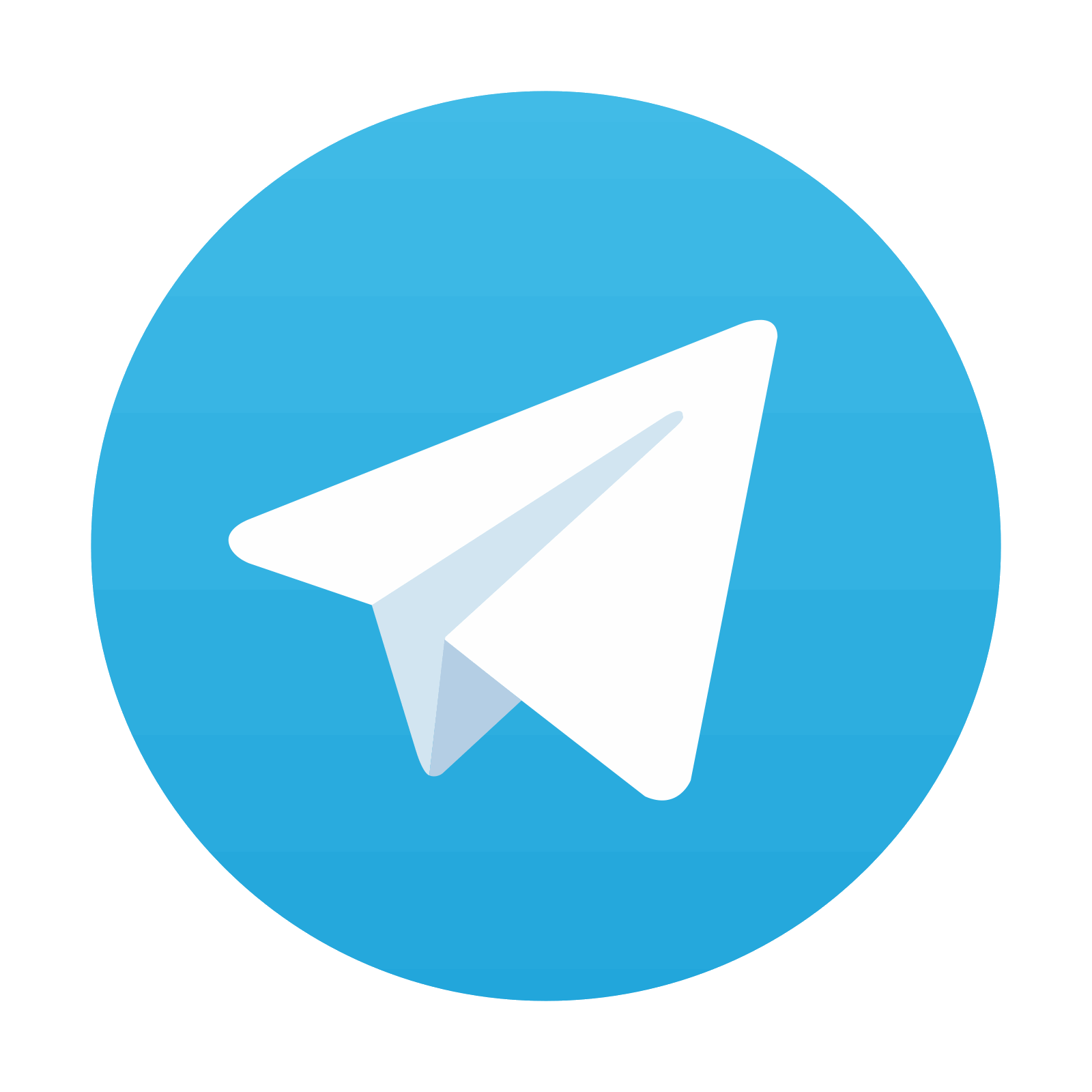
Stay updated, free articles. Join our Telegram channel
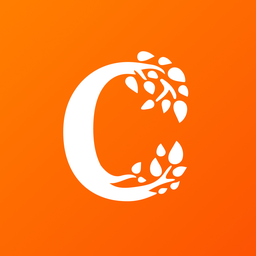
Full access? Get Clinical Tree
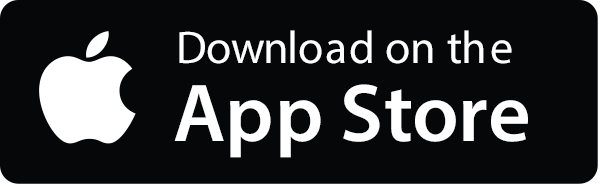
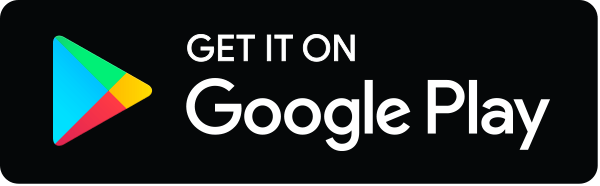