© Springer Science+Business Media New York 2015
Joseph C. Liao and Li-Ming Su (eds.)Advances in Image-Guided Urologic Surgery10.1007/978-1-4939-1450-0_88. Light Reflectance Spectroscopy and Autofluorescence (Kidney and Prostate)
(1)
Department of Urology, University of Texas Southwestern Medical Center, Dallas, TX, USA
(2)
Department of Urology, UT Southwestern Medical Center, Dallas, TX, USA
Keywords
light reflectance spectroscopyautofluorescence spectroscopykidneyprostatecancerSpectroscopy involves the study of the interaction of light and other radiation with matter, and its capabilities for diagnostic and therapeutic medical applications have been extensively investigated in recent years. As light travels through a substance, it is reflected, absorbed, transmitted, and/or scattered in a very specific pattern that is dependent on the wavelength of the incident light as well as the molecular and structural composition of the illuminated target; the study of this interaction can be exploited for the noninvasive identification and quantification of specific molecules within a sample. A plethora of spectroscopy types have been applied in medicine, including but not limited to absorption, fluorescence, visible reflectance, ultraviolet, infrared, near-infrared (NIR), Raman, and nuclear magnetic resonance.
Light reflectance spectroscopy measures the intensity of diffusely reflected light after interaction with tissue. The intensity of reflected light as a function of the wavelength defines the reflectance spectrum, which is measured for each wavelength of light produced by a broadband light source [1]. Light reflectance spectroscopy is generally applied in the study of substances located at or near the surface of samples. Light interaction with tissue also includes absorption and scatter. Tissue absorption is characterized spectroscopically by the absorption coefficient, which is directly related to the concentration of the molecule absorbing the incident light. Concentrations of molecules such as beta-carotene and hemoglobin are measurable in the visible light range, while water, adipose tissue, and collagen are measurable in the NIR range [2]. Light scattering by tissue is dependent upon the underlying cellular structure and contains information about cellular morphology as well as about processes such as cellular death and proliferation [2].
Tissue autofluorescence refers to the natural emission of fluorescent light by endogenous fluorophores when excited by light of a suitable wavelength [3]. It is an intrinsic property of cells and tissue. Within cells, endogenous fluorophores include aromatic amino acids, lipo-pigments, and flavin coenzymes that are largely contained within mitochondria and lysosomes. Within tissues, endogenous fluorophores consist primarily of collagen and elastin that compose the extracellular matrix [3]. Changes in the amount and distribution of endogenous fluorophores and/or their microenvironments due to various biological processes can be monitored by autofluorescence spectroscopy.
Light reflectance spectroscopy and fluorescence spectroscopy have been investigated for potential use in a wide variety of clinical applications, including but not limited to differentiation between benign and malignant skin lesions, early detection of premalignant lesions in the oral cavity, differentiation between benign and malignant breast lesions, differentiation between benign and malignant endobronchial lesions, early detection of cervical abnormalities, and detection of esophageal dysplasia [4–10]. In urology, these techniques have been investigated in studies involving bladder, kidney, and prostate tissue. In this chapter, we review the research to date on application of light reflectance and autofluorescence spectroscopy in the kidney and prostate.
Light Reflectance Spectroscopy in Kidneys
Conventional Reflectance Spectroscopy
Fitzgerald et al. reported the earliest uses of optical spectroscopy in kidney tissue diagnosis [11]. They described autofluorescence and NIR-reflectance spectra in ischemic kidneys and nonischemic controls in a rat model of warm and cold ischemia in pre-transplanted kidneys. Several intervals of ischemia from 0 to 120 min were studied. NIR-reflectance spectroscopy was found to achieve the best differentiation between normal and ischemic kidneys when backscattered light was captured through an 800 nm filter. The intensity ratio of injured/normal was found to consistently decrease with increasing warm ischemia time, providing a possible framework for future quantification of the duration of warm ischemia time in pretransplant allografts, an important outcome that is often “guessed” by the transplant surgeon [11].
Parekh et al. reported on the first use of optical spectroscopy for differentiating malignant from benign renal tissue [12]. Similar to Fitzgerald and colleagues, they used fluorescence and NIR reflectance spectroscopy, alone and in combination. Spectra were measured ex vivo in human kidneys removed during radical nephrectomy. The authors found that the intensity of reflectance spectra for renal cell carcinoma (RCC) increased with increasing wavelength in the 600 and 800 nm (NIR) range [12]. This was true for both clear cell and papillary RCC. One patient in the series had a benign diagnosis (cystic nephroma) and was found to have an opposite spectral trend, whereby reflectance intensity decreased with increasing wavelength in the 400–850 nm range. These findings were distinct from those observed for normal kidneys, which showed no change in reflectance intensity with increasing wavelength [12]. Hemoglobin spectra were prominent between 400 and 600 nm. When combined with fluorescence spectroscopy parameters, algorithms were developed which distinguished cancerous from noncancerous kidneys with high sensitivity and specificity [12].
Similarly, Bensaleh et al. investigated the use of reflectance spectroscopy in the NIR and visible light ranges in the differentiation of tumor-bearing from normal renal tissue in patients undergoing radical or partial nephrectomy [13]. A goal of their study was to evaluate the potential usefulness of optical spectroscopy in assessment of positive margins in partial nephrectomy specimens. To minimize the influence of hemoglobin on their data, NIR spectra were analyzed between 630 and 880 nm, while visible light spectra were analyzed between 550 and 620 nm. Hemoglobin has strong absorption peaks at 400–410 nm and secondary peaks at 550–600 nm, depending on its state of reduction/oxidation. Spectra were measured at multiple locations within the tumor as well as within normal regions of the kidney including at the resection margin in partial nephrectomy specimens. Twenty-one specimens were analyzed, 15 with malignant histology (14 clear cell and 1 papillary RCC) and 6 with benign histology (oncocytoma). There was one positive margin in the series in a patient with oncocytoma. The authors found that in the NIR region (630–880 nm), slopes for the reflectance curves were significantly different between tumor-bearing and normal renal tissue (p = 0.03). In the visible range, there were strong correlations within individual spectral measurements obtained intra-tumor and those obtained within normal kidney but poor correlations between tumor and normal [13]. In the patient with a positive margin, there was a poor correlation between measurements at the presumed positive margin site and adjacent parenchymal margin but a strong correlation between positive margin site and tumor. The authors concluded that in conjunction with NIR spectroscopy, quantifying correlations in spectra between tumor and normal kidney could be a useful technique to assess for positive margins during partial nephrectomy [13].
In a follow-up study within the same patient cohort, Bensaleh et al. used similar methodology to determine the usefulness of optical reflectance spectroscopy in differentiating between malignant and benign renal tissue [14]. Twenty-eight spectra obtained from malignant tissue were compared with 12 spectra obtained from benign tissue. The slope of the mean reflectance curve for malignant tissue significantly differed from that for benign tissue in both the visible light (p = 0.02) and NIR (p = 0.005) regions. Correlations within malignant tumor and within benign tumor were strong (r > 0.95 for each) but were poor for malignant vs. benign tumor (r−0.49) [14].
Recently, Couapel et al. investigated the ability of light reflectance spectroscopy and Raman spectroscopy to differentiate benign from malignant renal tissue as well as to differentiate between histological subtypes of RCC [15]. Spectra were collected prospectively in a cohort of 60 patients who underwent radical or partial nephrectomy for suspicion of cancer. Support vector machine (SVM) analysis was used for comparison of benign vs. malignant and also for comparison among histologic subtypes. A subset analysis was performed for small renal masses (<4 cm in size). A random integer number function was used in conjunction with the SVM where a discrepancy between the sample numbers of spectra existed. Light reflectance spectroscopy was performed using similar methodology as previously reported by Bensaleh et al. [13, 14]. The SVM for light reflectance spectroscopy was found to differentiate malignant from benign renal tissue with 88 % accuracy (sensitivity 96 % and specificity 80 %). For small renal masses, accuracy improved to 95 % (sensitivity 98 % and specificity 92 %). Accuracy for distinguishing between histological subtypes was 88–90 % overall and 89–95 % for small renal masses. Accuracy for distinguishing between chromophobe RCC and benign histology was 98–100 % [15]. To date, these findings have not been investigated in vivo and technology that would enable this is pending.
Hyperspectral Imaging
Hyperspectral imaging (HSI) is a unique imaging system developed in the early to mid-1980s that combines high-resolution remote imaging with reflectance spectroscopy, such that each image pixel carries spectroscopic information about the illuminated target [16]. It has been used in a wide variety of settings, such as in the military for identifying ground targets from airborne and satellite platforms, in identifying oil fields, in mapping of earth’s minerals, and many others. Its use in the clinical setting was first described in 2001 [17], when Zuzak et al. developed the first portable HSI system. This early prototype used a liquid crystal tunable filter (LCTF) system for recording of high-resolution spectra and a large array detector for the detection and rendering of a spectrally resolved macroscopic image of the target. The authors demonstrated the usefulness of this system in monitoring skin perfusion in real time in an experimental model of vascular dysfunction [17, 18]. Digital light processing technology (DLP®; Texas Instruments, Dallas, TX), which employs a digital micro-mirror array device to produce complex spectral illumination at extremely fast switching rates, was subsequently incorporated with HSI. This greatly reduced imaging processing times from approximately 30–40 s per image with conventional LCTF systems, to 3–4 frames per second in the “3-shot” mode [19]. The current DLP®-HSI system is calibrated for measurement of hemoglobin spectra. In this setting, the DLP® chip is incorporated with a broadband white light source, and by digitally programming the micro-mirror array of the DLP® chip, an illumination spectrum spanning the reflectance range for oxy- and deoxyhemoglobin (520–645 nm) is delivered by the light source. Reflected light from the illuminated target is captured by a focal plane array detector, digitized, and transferred to a computer for processing. A customized computer algorithm synchronizes the selective output from the light source with that from the image detector and deconvolutes the sample reflectance spectra by performing a least squared fit to determine the best linear combination of oxy- and deoxyhemoglobin at each detector pixel. The relative hemoglobin saturation at each detector pixel is calculated, and these data are color-coded using a combination of colors between blue (deoxyhemoglobin) and red (oxyhemoglobin). The color-coded image is displayed on the computer monitor as an “oxygenation map” of the kidney. Changes in relative hemoglobin saturation (% HbO2) can qualitatively and quantitatively be tracked in real time using this system.
The DLP®-HSI system has successfully been used in several preclinical studies of renal ischemia during partial nephrectomy. In a porcine model, Tracy et al. demonstrated a difference in kidney oxygenation with artery only vs. artery+vein occlusion during partial nephrectomy, with significantly higher oxygenation levels for artery only occlusion between 16 and 24 min of ischemia [20]. The authors hypothesized that the DLP®-HSI system may be useful in identifying an “ischemic window” in which artery only occlusion may provide benefit over artery+vein occlusion. Best et al. demonstrated that the DLP®-HSI system was sufficiently sensitive to detect subtle differences in renal oxygenation during partial nephrectomy when comparing 0 % vs. 10 % vs. 25 % arterial inflow during parenchymal resection [21]. In their solitary kidney model, they further demonstrated that 25 % inflow resulted in significantly less change from baseline serum creatinine postoperatively [21].
DLP®-HSI has also been used in several studies of open and minimally invasive partial nephrectomy in humans. In a cohort of 21 patients undergoing open partial nephrectomy, Holzer et al. demonstrated that relative hemoglobin saturation (%HbO2) decreased to nadir levels (20 % below baseline levels), within 10 min of hilar occlusion, with baseline levels restored within 6 min of reperfusion [22]. Best et al. demonstrated significant baseline variation in baseline %HbO2 in 26 patients undergoing open partial nephrectomy, with preservation of early postoperative estimated glomerular filtration rate (eGFR) only in patients with baseline %HbO2 >75 % [23]. Subsequently, Liu et al. investigated artery only vs. artery+vein occlusion in 37 patients undergoing open partial nephrectomy, demonstrating that in the artery+vein group, a lower mean ischemic %HbO2 was associated with significantly lower eGFR at most recent follow-up on both univariate and multivariate analysis [24]. Most recently, Olweny et al. used a novel laparoscopic DLP®-HSI system to study renal oxygenation in patients undergoing robotic-assisted laparoscopic partial nephrectomy [25]. They demonstrated a significant inverse association between baseline %HbO2 and baseline eGFR as well as between baseline %HbO2 and eGFR at most recent follow-up (p < 0.05). Furthermore, younger patients had a lower median baseline %HbO2 and a significantly higher median preoperative eGFR, perhaps reflective of age-related decline in renal oxygen consumption. No significant associations between %HbO2 and diabetes, hypertension, and smoking history were observed [25].
The promising findings of these initial studies with DLP®-HSI experimentally and in humans suggest that pending further device enhancements and output parameterization, it has the potential for development as an optimal device for the intraoperative assessment of specific aspects of renal metabolism in real time. Furthermore, calibration of the device for measurement of spectra other than those for hemoglobin can potentially expand versatility and therefore capabilities for use in a wide variety of tissue types.
Blanco et al. evaluated the use NIR-hyperspectral imaging in characterizing ex vivo kidney stones [26]. Artificial neural networks were used to analyze the unique spectra generated for each different stone type, and an algorithm that achieved a greater than 90 % probability for the correct classification of the stones was created. This may aid future rapid identification of renal stone composition, reducing latent errors in stone classification characteristic of present-day techniques [26].
Autofluorescence Spectroscopy in Kidneys
The initial study of use of optical spectroscopy kidney tissue diagnosis by Fitzgerald et al. [11] was notable for the evaluation of both light reflectance and autofluorescence spectra. Data for NIR-reflectance spectroscopy were presented above. With regard to autofluorescence, the authors similarly found that the intensity ratio of injured/normal kidney consistently decreased with increasing warm ischemia time. The best differentiation between normal and ischemic kidneys with autofluorescence was achieved with a 450 nm emission filter, following excitation with a 335 nm laser [11]. NADH, produced during oxidative metabolism, is known to have peak fluorescence at 470 nm when excited at <380 nm [27]. Although NADH could have accounted for some of the fluorescence observed, the authors postulated that a compound other than NADH was also likely, given the decrease in autofluorescence intensity ratio with increasing warm ischemia time. Alternatively, changes in pH could have altered the emission efficiency of the endogenous fluorophores [11]. In follow-up studies, the same group demonstrated the feasibility of using autofluorescence spectroscopy to monitor real-time kidney tissue changes during ischemia and reperfusion in an in vivo rat model [28–30]. A limitation of the above studies was the absence of correlation of autofluorescence spectroscopic findings with tissue histology. Tirapelli et al. addressed this limitation in studies in which grades of ischemic renal tissue injury at several intervals of ischemia were correlated with autofluorescence at 520 nm (excitation 442 nm) and 622 nm (excitation 532 nm). A strong association between tissue damage and autofluorescence intensity was demonstrated, more pronounced with excitation at 442 nm [31, 32].
< div class='tao-gold-member'>
Only gold members can continue reading. Log In or Register a > to continue
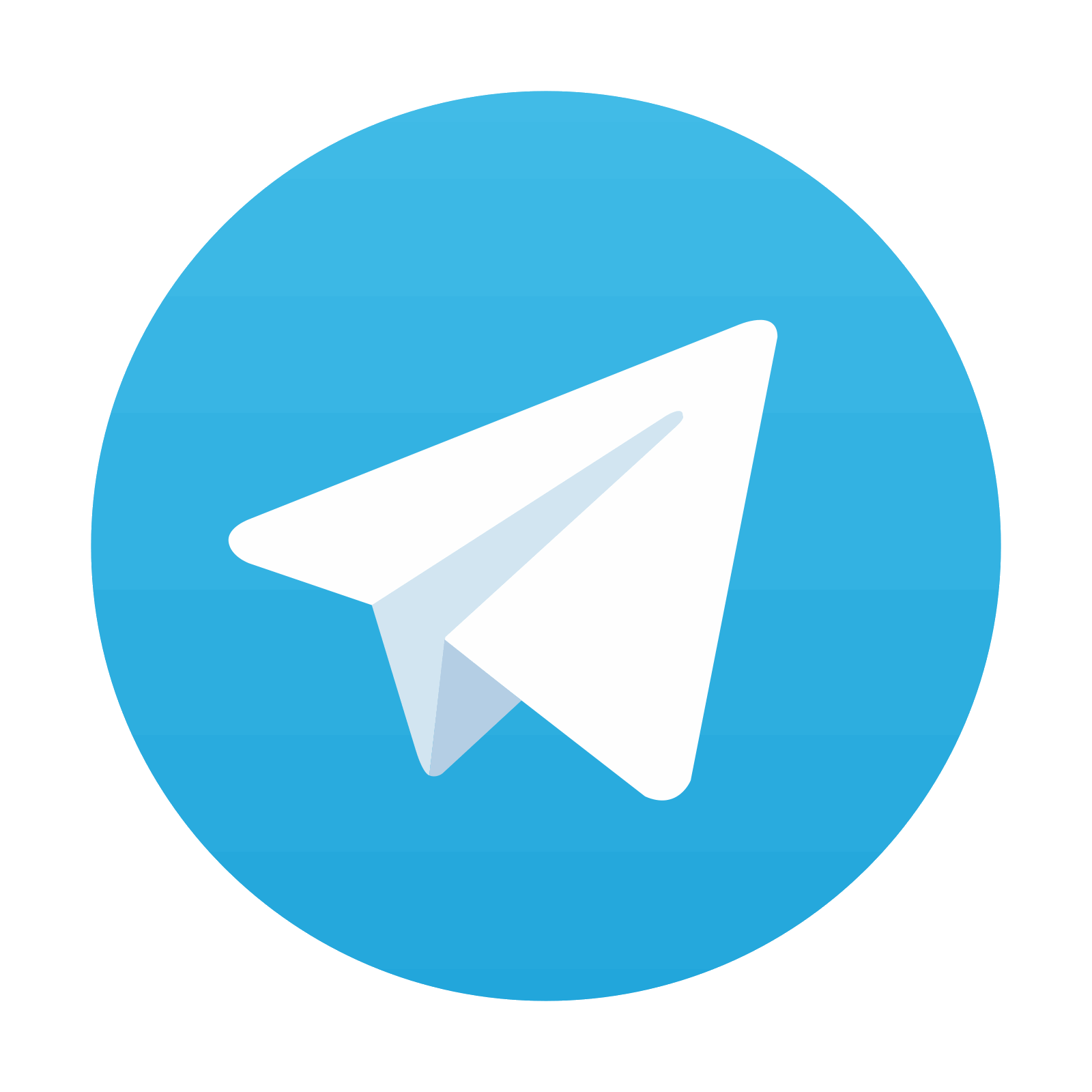
Stay updated, free articles. Join our Telegram channel
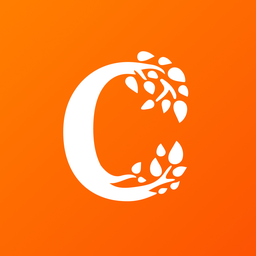
Full access? Get Clinical Tree
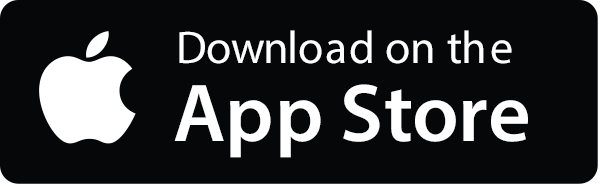
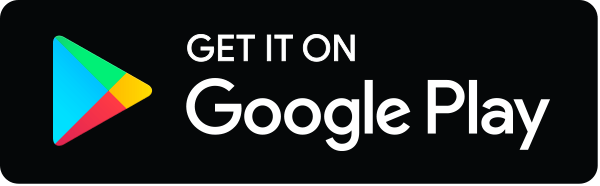