Fig. 2.1
Mechanisms of erythropoiesis and iron homeostasis
Enterocyte iron absorption is an extremely complicated process whose scope goes beyond the goal of this presentation. Understanding of some basic elements are important in managing any patient with iron deficiency [17]. Mammals absorb dietary iron through the duodenal epithelium which is organized in villous structures that maximize absorptive surface area. Enterocyte precursors are present at the bases of the villous crypts and migrate up the villous axis as they differentiate. Enterocytes have a brush border that additionally amplifies the surface area for absorption. Mature enterocytes live for only 1–2 days. Iron that accumulates within them is lost from the body when senescent enterocytes are shed into the gut lumen. The crucial export step is regulated by hepcidin , a hormone produced by the hepatocytes in response to iron stores.
Intestinal iron uptake from the gut lumen requires a number of transporters and the right valence of iron. Proteins for the uptake of heme and inorganic iron reside on the brush-border membrane. A key transporter is divalent metal transporter 1 (DMT1) which is activated by hypoxia-inducible factor 2α (HIF-2α) [18]; it requires proton cotransport. Most non-heme iron in the diet is present as the Fe3+ form. DMT1 exclusively transports divalent metals, necessitating luminal conversion of Fe3+ to Fe2+. This occurs through the action of duodenal cytochrome b (Dcytb) , expressed in the intestinal mucosa by hypoxia, iron deficiency, or anemia. The intestine also absorbs heme iron from the diet; such heme absorption is a saturable, carrier-mediated process. The heme enterocyte importer, termed heme carrier protein 1 (HCP1), resembles bacterial proteins that transport metal-tetracycline complexes. Once dietary heme has entered the intestinal epithelial cell, it is cleaved by intracellular heme oxygenase 1 to release iron, the iron probably joining the same intracellular pool as non-heme iron.
Intracellular enterocyte iron is either stored in the multimeric protein ferritin or transported across the basolateral membrane of the enterocyte into the circulation. Ferroportin is the basolateral iron exporter as intestinal expression of ferroportin mRNA and protein increases in response to iron deficiency and hypoxia [19]. Selective inactivation of the murine ferroportin gene in intestinal cells has established that ferroportin is the major, if not only, intestinal iron exporter. Hepcidin binds to and internalizes this transporter.
To ensure that very little iron is free within cells, iron regulatory proteins (IRP1 and IRP2) control the posttranscriptional expression of genes modulating cellular iron uptake and storage. Cellular iron in excess of immediate needs is stored as an iron oxide solid within ferritin, a polymeric protein composed of varying ratios of heavy (H) and light (L) ferritin polypeptides [20]. The presence of IREs in DMT1 and ferroportin mRNAs during heme synthesis in erythrocytes suggests that their expression is likely controlled, at least in part, by cellular iron content. Data from animal models establish that the Tfn cycle is critical for iron uptake into erythroid cells [21].
Recycling of iron from senescent erythrocytes provides most of the iron utilized by developing red blood cells. Senescent red cells are removed from circulation by specialized macrophages in the spleen, bone marrow, and liver for the recovery of their iron. It is believed that accumulation of phosphatidylserine in the outer leaflet of the erythrocyte membrane seems to be a primary event [22] but Ca2+ flux, removal of sialic acids on the cell surface, and opsonization of red blood cells by autoantibodies are all possible signals for turnover [23]. The scavenger receptor CD36 [24] is a likely candidate for the macrophage-specific receptor. After the red cell has been internalized into an acidic phagosome, heme oxygenase liberates iron from heme; iron egress from macrophages is partly, if not entirely, mediated by ferroportin whose function is regulated by hepcidin.
Iron acquisition is thus tightly regulated by hepcidin [16]. The hepcidin homeostatic control mechanism regulates both duodenal enterocytes iron absorption (final step of transport) as well as macrophage recycling of iron (efflux of iron, mostly spleen, and bone marrow). Hepcidin functions as an acute-phase reactant that adjusts fluctuations in plasma iron levels caused by absorptive enterocytes and macrophages by binding to and inducing the degradation of ferroportin, which exports iron from cells [25]. Liver hepcidin expression increases in response to high circulating and tissue levels of iron (its physiologic signal) but it also increases during systemic inflammation or infection. Increases in hepcidin levels induced by inflammatory cytokines, especially interleukin-6, explain the iron sequestration and reduced supply of iron to the bone marrow iron that occurs in the anemia of chronic disease. On the other hand, expression and thus production of hepcidin is decreased during states of expanded erythropoiesis, in iron deficiency, and with tissue hypoxia in response to signals originating in the bone marrow (erythroferrone), the liver, and probably muscle tissue and adipocytes [26]. Hepcidin is crucial in regulating total-body iron within normal ranges, avoiding both iron deficiency and excess.
In iron deficiency, the transcription of hepcidin is suppressed. This adaptive mechanism facilitates the absorption of iron (Fig. 2.1) and the release of iron from body stores. The rapidity of iron repletion varies with the degree and rapidity with which iron deficiency develops. Although spleen and bone marrow macrophages are the primary “reservoirs” for iron storage in the body, hepatocytes do appear to be a long-term reservoir for iron. However, since hepatocytes do not have cell membrane ferroportin, the release of iron from hepatocytes is much slower than from macrophages.
Iron circulates in plasma bound to the 80-kDa serum glycoprotein transferrin (Tfn) which contains two specific high-affinity Fe3+ binding sites with an overall association constant of 1020 M−1 at pH 7.4 [27]. In humans, transferrin consists of a polypeptide chain containing 679 amino acids and two carbohydrate chains and has both alpha helices and beta sheets that form two domains [28]. The amino acids which bind iron ion to the transferrin are identical for both of the binding sites; two tyrosines, one histidine, and one aspartic acid. For the iron ion to bind, an anion is required, preferably carbonate (CO2 −3). In summary, iron-binding blood plasma glycoprotein controls the level of free iron in biological fluids. Diferric Tfn binds to a highly specific Tfn receptor (TfnR1) [29], permitting cellular uptake by receptor-mediated endocytosis and the formation of clathrin-coated pits that facilitate transferrin internalization into endocytic vesicles. As these endosomes become acidified, protein conformation changes at a pH of 5.5 cause iron to dissociate from Tfn [30]; Fe3+ is then reduced to Fe2+, for transport from the endosome to the cytoplasm by the transporter DMT1. The Tfn cycle is completed when the endosome returns to and fuses with the plasma membrane, returning apotransferrin to the circulation and TfnR1 to the plasma membrane to start the cycle again.
The transferrin iron-bound receptor is a disulfide-linked homodimer. Transferrin plays a key role in areas where erythropoiesis and active cell division occur. It is also found in mucosal tissues; by binding iron and creating and a low free iron environment, it impedes bacterial survival in a process called iron withholding. Levels of transferrin decrease (acute phase reaction) during systemic inflammation, as well as with cancers and certain other diseases [31].
Etiology
The most common causes of iron deficiency are summarized in Table 2.1.
Table 2.1
The most common causes of iron deficiency
Cause | Examples |
---|---|
Physiologic | |
Increased demand | Infancy, rapid growth in adolescence, menstrual blood loss, pregnancy, blood donation |
Dietary or environmental | Insufficient intake, resulting from poverty, malnutrition, diet (e.g., vegetarian, vegan, iron-poor) |
Pathologic | Decreased absorption: gastrectomy, duodenal bypass, bariatric surgery, Helicobacter pylori infection, celiac sprue, atrophic gastritis, inflammatory bowel diseases (ulcerative colitis, Crohn’s disease) |
Chronic blood loss: gastrointestinal tract, including esophagitis, erosive gastritis, peptic ulcer, diverticulitis, benign tumors, intestinal cancer, inflammatory bowel diseases, angiodysplasia, hemorrhoids, hookworm infestation, obscure source | |
Genitourinary system: heavy menses, menorrhagia, intravascular hemolysis (paroxysmal nocturnal hemoglobinuria, autoimmune hemolytic anemia, march hemoglobinuria, damaged heart valves, microangiopathic hemolysis (TTP/HUS) | |
Systemic bleeding: hemorrhagic telangiectasia, chronic schistosomiasis, Munchausen’s syndrome (self-induced hemorrhages) | |
Drug-associated | Glucocorticoids, salicylates, NSAIDs, proton-pump inhibitors |
Genetic | Iron-refractory iron-deficiency anemia (IRIDA) |
Iron-restricted erythropoietic | Treatment with erythropoiesis-stimulating agents (ESA), anemia of chronic disease, chronic kidney disease |
Poverty, malnutrition, and famine are the main causes for anemia from iron deficiency in developing countries, especially in children and pregnant women [11]. Cereal-based diet decreases iron bioavailability as they contain phytates that sequester iron into a poorly absorbable complex. Other common causes in developing countries include hookworm infections and schistosomiasis, which cause chronic blood loss [10]. Strict vegan and vegetarian diets, malabsorption, and chronic blood loss resulting from heavy menstrual losses are also well-known causes of iron-deficiency anemia. Chronic blood loss from the gastrointestinal tract, including occult blood, especially in male patients and elderly patients, may reveal the presence of benign lesions, angiodysplasia, or cancer. Obscure gastrointestinal blood loss, especially from the small bowel, may be seen by means of video-capsule endoscopy [32]. This technique is increasingly used when conventional workups for iron-deficiency anemia is negative [33]. Persons who donate blood regularly are also at risk for iron deficiency, and their iron levels should be monitored.
In rare forms of intravascular hemolysis as in in paroxysmal nocturnal hemoglobinuria, iron is lost in the urine, the iron deficiency then aggravating the hemolytic anemia. Anemia develops in endurance athletes, perhaps from some hemolysis, blood loss, and/or mild inflammation. Nonsteroidal anti-inflammatory drugs (NSAIDS) , anticoagulants more so than antiplatelet agents can aggravate blood loss. Proton-pump inhibitors (PPIs) by reducing acid secretion impair iron absorption (Table 2.1) [34].
Multiple causes may be operative in any given person. Low iron intake in the presence of intestinal infections with nematodes may result in severe anemia [35]. Blood losses may combine with the anemia of inflammation [5]. In end-stage kidney disease (ESRD) , iron-deficiency anemia results from dialyzer blood loss (average of 1–2 g elemental Fe per year), increased hepcidin from reduced hepcidin clearance and from inflammation, and PPIs and anticoagulant use. In elderly persons, the anemia correlates with advanced age and multiple related conditions, including iron deficiency [36] inflammatory disorders, decreased levels of erythropoietin, and cancer [7, 30]. Obesity, now reaching epidemic proportions in Western societies, is associated with mild iron deficiency [7]. The processes include subclinical inflammation, increased hepcidin levels, and decreased iron absorption [37].
In most cases, resistance to oral iron therapy is due to disorders of the gastrointestinal tract (Table 2.1). Partial or total gastrectomy or surgical procedures that bypasses the duodenum can produce such resistance. Bariatric surgery, such as laparoscopic Roux-en-Y gastric bypass, which is performed in obese patients is an emerging cause of iron deficiency (up to 45% of subjects) [38] and anemia because the procedure effectively removes an active iron absorption site from the digestive process and increases gastric pH [39]. Lifelong nutritional monitoring and iron supplementation are advisable [40].
Infection with Helicobacter pylori infection leads to decreased iron absorption (Table 2.1) as the microorganism competes with its human host for iron, reduces the bioavailability of vitamin C, and may lead to micro-erosions that cause bleeding [41]. Since it is estimated that half the world’s population is infected with H. pylori, clinicians should be aware of the possibility of infection and provide treatment in order to eradicate this acquired source of iron-resistant iron-deficiency anemia.
The prevalence of celiac disease and its atypical manifestations, which include iron-deficiency anemia, appear to be increasing worldwide [42]. Whether gluten allergy contributes to iron deficiency is unclear. The incidence among iron-replete participants for a positive anti-transglutaminase antibodies is negligible; however, gluten sensitivity by this antibody test was found in 2.5% of participants with iron deficiency and seemed to occur in Caucasians [43]. In another study of a series of patients with iron-refractory iron-deficiency anemia, 5% of participants had gluten sensitivity [44]. These findings suggest that gluten sensitivity may be associated with secondary iron-refractory iron-deficiency anemia. Similarly, autoimmune atrophic gastritis is another rare cause of iron-refractory iron-deficiency anemia, resulting from an immune reaction against gastric parietal cells and intrinsic factor, should be considered as a possible albeit unlikely cause of iron-refractory microcytic anemia [44]. In patients with inflammatory bowel disease (IBD) , anemia may be iron-resistant, but it is multifactorial from a combination of deficiencies in iron, folate, and vitamin B12, inflammation, and side effects from drug therapy.
An uncommon but important entity with respect to our understanding of iron sensing by the liver is an entity known as iron-refractory iron-deficient anemia (IRIDA) . It is a rare autosomal disorder characterized by the absence of a hematologic response (an increase of <1 g of hemoglobin) after 4–6 weeks of treatment with oral iron [45]. IRIDA is caused by a mutation in TMPRSS6 [45], the gene encoding transmembrane protease serine 6, also known as matriptase-2, which inhibits the signaling pathway [46] that activates hepcidin [47]. Loss-of-function mutations in TMPRSS6 have been reported in many families [48]. In these families, constitutively high production of hepcidin is noted. Hepcidin blocks the intestinal absorption of iron despite the presence of anemia. Anemia is variable, more severe in children, and unresponsive to treatment with oral iron. Microcytosis is striking, transferrin saturation is very low in the presence of normal or borderline-low ferritin levels and high hepcidin levels [49]. The diagnosis ultimately requires sequencing of TMPRSS6. Although IRIDA represents <1% of the cases of iron-deficiency anemia seen in medical practice, knowledge of this condition is valuable to clinicians, since it clarifies how essential the suppression of hepcidin is to the body’s response to pharmacologic iron. IRIDA points to the existence of genetic susceptibility to iron deficiency. Variants of TMPRSS6 have been associated with modulation of serum hepcidin levels in individual persons [50] as well as variation in iron levels in population studies [51].
Diagnostic Procedures
The traditional laboratory measures used to determine iron status and iron deficiency and related conditions (e.g., functional iron deficiency, iron-deficiency anemia, IRIDA, and anemia of chronic diseases) are now well established. Older studies of iron status in blood donors used various biochemical markers including serum iron, serum transferrin, transferrin saturation (TSAT), and serum ferritin levels [52]. In these studies, iron depletion was considered to be present if the ferritin concentration was below 12 μg/dL. However, this cut off failed to identify iron deficiency in over one-third of blood donor cases. Other investigators have found that a higher ferritin concentration between 22 and 40 μg/dL [53] better reflects functional iron depletion. These findings were based on more sensitive measures of iron status such as serum (soluble) transferrin receptor (sTfR) levels, which reflect the functional iron compartment and have been shown to correlate with depleted iron stores in marrow preparations [54].
Cook et al. described the use of the ratio of sTfR to ferritin (R/F) [55] for assessing body iron stores and determining iron deficiency from a small capillary blood specimen requiring no venipuncture. Analysis showed a single normal distribution of body iron stores in US men aged 20–65 years (mean ± 1 SD, 9.82 ± 2.82 mg/kg). Distribution analysis in US women aged 20–45 years indicated two populations; 93% of women had body iron stores averaging 5.5 ± 3.35 mg/kg whereas the remaining 7% of women had a mean tissue iron deficit of 3.87 ± 3.23 mg/kg. Quantitative estimates of body iron greatly enhance the evaluation of iron status and the sensitivity of iron intervention trials in populations without inflammation. Moreover, it eliminates the need for a TSAT determination.
In another study of almost 1700 male and female blood donors, studies were performed to see if better criteria could be developed [56]. Absent iron stores (AIS) was defined as plasma ferritin level of less than 12 μg/L. The logarithm of the ratio of soluble transferrin receptor to ferritin of at least 2.07 (≥97.5% in FT/RA males) was used to define iron-deficient erythropoiesis (IDE). Receiver operating characteristics analysis was performed to assess selected RBC indices (e.g., percentage of hypochromic mature RBCs, proportion of hypochromic mature RBCs [%HYPO], and hemoglobin [Hb] content of reticulocytes [CHr]) in identifying AIS and IDE; %HYPO and CHr detected IDE with comparable sensitivity, 72% vs. 69%, but differed in specificity: %HYPO 68% and CHr 53%. For detecting absolute iron deficiency, sensitivity was improved to 85% for %HYPO and 81% for CHr but specificity was reduced for both. Venous Hb at (<12.5 g/dL) had high specificity but poor sensitivity for iron deficiency, whether relative or absolute. The sensitivity of hemoglobin measurements is poor because anemia associated with nutritional iron deficiency is relatively mild, resulting in extensive overlap in Hb values between healthy and iron-deficient persons [57]. A plasma ferritin level of less than 26.7 μg/L was a good surrogate for assessing IDE. The most recent guidelines for the differential diagnosis of microcytic anemias have been published elsewhere [58]. The current clinical consensus is to use a ferritin level of <30 μg/L as the most sensitive and specific test for identification of iron deficiency. Levels are even lower in patients with iron-deficiency anemia.
However TSAT is still used by many to determine iron status [27]. A value of less than 16% indicates an iron supply that is insufficient to support normal erythropoiesis. However, in determining iron status, it is important to consider the entire picture rather than using a single result. In nephrotic syndrome, urinary loss of transferrin, along with other serum proteins can manifest as iron-resistant microcytic anemia.
Diagnosis of iron-deficiency anemia in the context of inflammation is challenging and cannot be determined on the basis of the results of a single test since many of the indicators are either positive (ferritin) or negative (transferrin) acute phase reactants. As a result, higher cutoff levels for ferritin are used to define iron-deficiency anemia accompanied by inflammation [59, 60], with the best predictor being a ferritin level of less than 100 μg/L. Higher ferritin cutoff levels are used in the diagnosis of iron deficiency in other conditions (e.g., <300 μg/L for heart failure [61] and for chronic kidney disease in the presence of a transferrin saturation level of less than 30%) [62]. The assessment of iron stores through iron staining of bone marrow specimens obtained by means of biopsy is an option that is used infrequently. ELISA kits for measuring plasma hepcidin are available [63] but there is significant variation from kit to kit. At present, C-reactive protein (CRP) level may be the most reliable screening tool to identify patients with inflammation.
The R/F ratio also has limited value in individuals with inflammation or liver disease although sensitivity/specificity of a value of 0.6 was superior in predicting response to IV iron than either TSAT <20% or ferritin <100 ng/mL, singly or used together [64]. More recently, a meta-analysis performed by the US Agency for Health Care Research (AHCR) [65] indicated that in CKD stage 3 or higher, both CHr and % hypochromic red cells have a similar or better overall test accuracy compared with classical markers (TSAT or ferritin) to predict a response to IV iron treatment (as the reference standard for iron deficiency). In addition, CHr (with cutoff values of <28 pg) and % hypochromic red cells (with cutoff values of >10%) have better sensitivities and specificities to predict iron deficiency than classical markers (TSAT <20 or ferritin <100 ng/mL). There is also evidence that sTfR has a similar test performance compared with classical markers (TSAT or ferritin) to predict a response to IV iron treatment. Across studies, a high degree of heterogeneity exists in the test comparisons, definitions for the reference standard (a response to IV iron treatment), iron status of the study populations (assessed by TSAT or ferritin), and background treatment. This heterogeneity limits final conclusions about the optimal test for iron deficiency.
The AHCR concluded that based on results from two randomized clinical trials, there is a reduction in the number of iron status tests and IV iron treatments administered to patients when CHr was used to guide iron management compared to when guided by TSAT or ferritin [65]. These results suggest that CHr may be a suitable alternative marker of iron status for guiding iron treatment, and could potentially reduce the frequency of iron testing and potential harms from IV iron treatment, particularly in those with some degree of inflammation.
Therapy
Oral Iron
The benefit of treating iron deficiency without the presence of anemia remains uncertain. Small studies show that the administration of intravenous iron (oral iron not studied) improves fatigue in women without anemia whose ferritin levels are in the iron-deficient range [66]. Some studies with limited number of participants and quite heterogeneous also suggest that oral iron supplementation benefits physical performance in women of reproductive age [67]. Patients with IDA should receive iron supplementation. However, emerging data suggest that nonabsorbed iron could be harmful by modifying the gut microbiota, increasing the concentration of intestinal pathogens [68]. Patients with severe iron-deficiency anemia producing cardiovascular symptoms, such as heart failure or angina, should receive red-cell transfusions to treat the tissue hypoxia of anemia. One unit of packed red cells also provides approximately 200 mg of iron helping to correct partially the iron deficiency.
Prevention of iron deficiency in at-risk populations is practiced in some parts of the world by fortifying foods with iron compounds [69], for instance iron sulfate (FeS) which is soluble or iron pyrophosphate (FePP) which is less soluble. In human studies, plasma ferritin is a strong negative predictor of Fe bioavailability from FeS but not from FePP. Thus, more soluble Fe compounds demonstrate better overall absorption and can be used at lower fortification levels and because their absorption is upregulated in Fe deficiency, they innately “target” Fe-deficient individuals in a population [70]. Both addition of ascorbate and Na2EDTA enhance iron absorption at molar ratios of 0.6:1 to 0.7:1 relative to fortification iron [71].
The administration of oral iron is a convenient, inexpensive, and effective means of treating stable patients. The low hepcidin levels in patients with uncomplicated iron-deficiency anemia ensure effective iron absorption and the recovery of hemoglobin levels; however, 3–6 months of treatment are often required for the repletion of iron stores and the normalization of serum ferritin levels. Mean values of absorption rates from 100 mg Fe in healthy males and females are ~5.0%, whereas in latent iron deficiency and in iron-deficiency anemia mean values of 10% and 13% are obtained, respectively. The maximum absorption rate is 20–25% [72]. With the conventional dose of 180–200 mg per day, less than 20 mg is absorbed explaining the long duration of therapy needed in most patients. Of note, studies of dosage amounts and frequency have shown that with increasing dose, fractional absorption decreases, whereas absolute absorption increases. A sixfold increase in iron dose from 40 to 240 mg only increases the amount of iron absorbed from 6.7 to 18.1 mg in non-anemic young women with plasma ferritin ≤20 μg/L. Providing lower dosages (40–80 mg Fe) and avoiding twice-daily dosing maximize fractional absorption. In fact, the hepcidin response to oral iron and its duration of the hepcidin response supports alternate day supplementation [73].
Among the myriad preparations on the market, iron sulfate is most frequently used; iron gluconate and iron fumarate are also effective. The recommended daily dose for adults with iron deficiency is 100–200 mg of elementary iron; for children, the dose is 3–6 mg per kilogram of body weight of a liquid preparation; for both groups, the supplement should be administered in divided doses without food. Addition of vitamin C is believed to increase absorption. Long-term use of oral iron is limited by side effects, including nausea, vomiting, constipation, and metallic taste; these side effects are frequent and, although not severe, are bothersome to some patients. Although oral iron may cause dark stools, it does not produce false-positive results on tests for occult blood. Failure of oral iron treatment typically results from premature termination of treatment, lack of compliance with the regimen, or discontinuation by the patient. Truly refractory response to treatment is uncommon and requires other, specific treatments, such as the eradication of infection with H. pylori or the introduction of a gluten-free diet in patients with celiac disease, may restore the capacity for iron absorption [43].
None of the markers of iron status are predictive of which patients will or will not have a response to oral iron therapy. Iron absorption studies in iron-deficient patients and healthy persons have been performed using iron radioisotopes [72], but they are no longer used in clinical practice due to radiation exposure and logistical difficulties. The oral iron challenge test in which either a low dose of 10–20 or a higher dose of 60 mg of oral iron is administered and serum iron levels are measured 1–3 h afterward is rarely used. Criteria include either the maximal increase in serum iron or the area under the curve [74, 75]. More recently, hepcidin has been used to predict the likelihood of response to oral iron in clinical studies. Those with low hepcidin levels were likely and those with high levels were unlikely to respond [76]. However, hepcidin tests are a specialty test and not routinely available for clinical use. The earliest response may be in the indices of RBC hemoglobin content in reticulocytes (CHr). A study in rheumatologic diseases and iron-deficiency anemia showed that a change in CHr and in serum levels of iron and transferrin saturation may predict the response to the administration of oral iron after 1 week of therapy [77].
One of the most frequent obstacles to oral iron repletion in daily practice is patients’ lack of adherence to what is prescribed. In this sense, individualized rather than “recommended” oral daily doses of 100–200 mg iron in divided doses might increase compliance. A dose of >120 mg per day is usually too much in that it greatly surpasses the maximum ability of the gut to absorb iron [77, 78] and it induces dose-dependent gastrointestinal, side effects that can be prevented by simply decreasing the dose. Administration of elemental iron at doses as low as 15 mg a day has been shown to correct anemia as effectively as administration of higher doses, with fewer adverse effects [79].
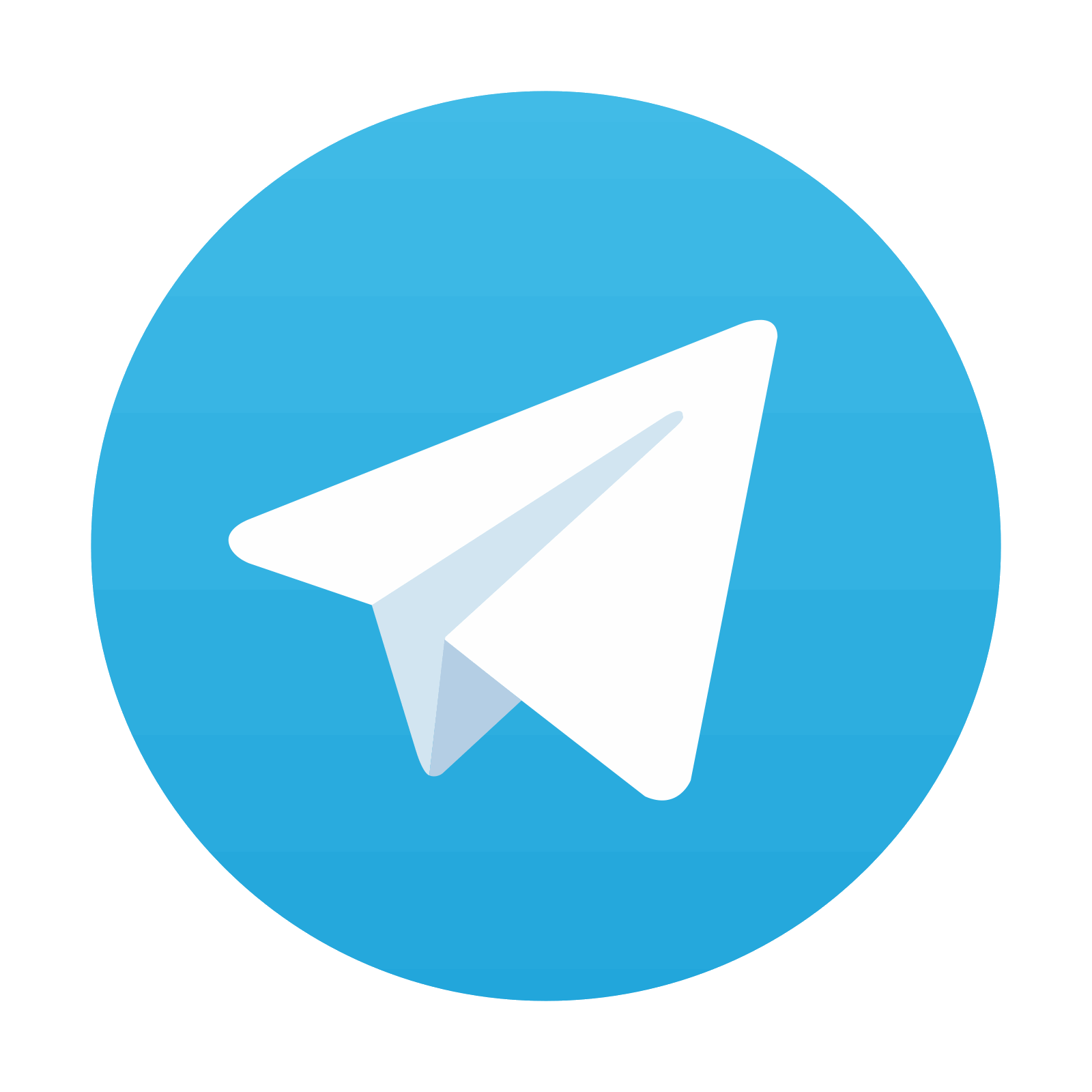
Stay updated, free articles. Join our Telegram channel
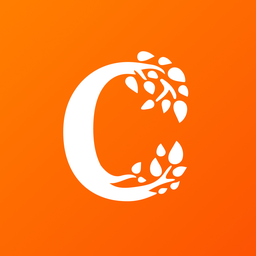
Full access? Get Clinical Tree
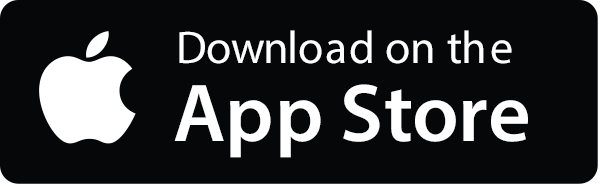
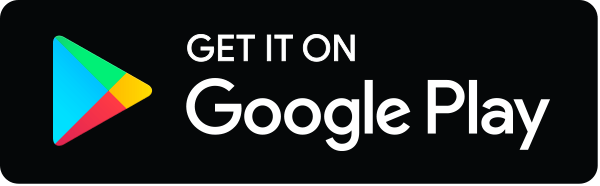