Intercellular junctions are relatively static regions of cell–cell contact found between epithelial, endothelial and mesothelial cells that have various functions. The morphology of the junctional complex in epithelia was defined by Farquhar and Palade in 1963, Farquhar MG, Palade GE. Junctional complexes in various epithelia. J Cell Biol . May 1963;17:375–412. We now know that it consists of four major components: the tight junction or zonula occludens , adherens junction, gap junction and desmosome. In this chapter we will review the cell biology and physiology of epithelial junctions, focusing on their role in the function of the renal tubule and the juxtaglomerular apparatus and potential involvement in kidney diseases. Although the slit diaphragm of the mature glomerular podocyte is a specialized form of intercellular junction, the reader is referred to Chapter 23 for a detailed discussion of this structure.
Keywords
Junctions, cell adhesion, ion channel, renal tubule.
Tight Junctions
Structure
General Structure
The tight junction is the most apical component of the junctional complex ( Figure 12.1 ). It is located at the boundary between the apical and lateral membranes, and encircles the cells to form a continuous belt-like attachment. By transmission electron microscopy, it appears morphologically as a series of appositions or “kisses” between the lateral membranes of adjacent cells ( Figure 12.2a ). The outer leaflet of the lipid bilayers approach each other so closely that they appear to be fused. In the technique of freeze-fracture electron microscopy, the hydrophobic phospholipid interior of the lipid bilayer is cleaved, allowing the tight junctions to be visualized within the plane of the lateral membrane. By this means, tight junctions appear as a series of continuous, anastomosing intramembranous strands on the protoplasmic or P-face, with complementary grooves on the exoplasmic or E-face ( Figure 12.5 ). It is believed that these strands associate in pairs from neighboring cells and obliterate the intercellular space, thus accounting for the kissing points seen by transmission electron microscopy ( Figure 12.2b ).


Early models of the tight junction posited that it was composed purely of lipid organized into inverted cylindrical micelles, that constituted the tight junction strands. There now exist three lines of evidence that refute this. First, tight junction strands were found to be resistant to deoyxcholate, an ionic detergent that should solubilize lipids. Second, the lipid model predicts that the outer leaflets of the bilayers of adjacent cells are continuous, yet no transfer of glycolipid or a fluorescent probe could be demonstrated. Finally, the discovery of integral membrane proteins within the tight junction now supports a model in which tight junction strands are composed of proteins embedded in the lipid membrane (see below).
Regional Differences in Structure
The structure of the tight junction varies considerably in different regions of the kidney. This is summarized briefly below. For further details, the reader is referred to comprehensive surveys by Orci, Brown, and colleagues.
Glomerulus
The parietal epithelium of Bowman’s capsule is constituted of squamous cells whose extensions overlap obliquely ( Figure 12.3 ). Their tight junctions consist of networks of two to four anastomosing strands with many discontinuities. The mature visceral epithelial cell (podocyte) forms a specialized intercellular junction, the slit diaphragm ( Chapter 23 ). Short elements of incomplete tight junctions can sometimes also be seen between the foot processes of adjacent podocytes. True tight junctions do form between immature podocytes in the fetal glomerulus and are of uncertain functional significance. These disappear during development, but can reappear during nephrotic states, coincident with effacement of the foot processes and obliteration of the slit pore ( Figure 12.4 ).


Renal Tubule
Tight junctions are found between the epithelial cells of all tubules, but they vary considerably in their morphology in different tubule segments ( Figure 12.5 and Table 12.1 ). In general, the more proximal segments tend to have simpler junctions, with fewer strands and more discontinuities, correlating with a leaky paracellular pathway, as judged by transepithelial resistance (TER). By contrast, more distal segments tend to have greater numbers of strands, with more continuity and complex anastomosing patterns, loosely correlating with higher TER. Indeed, Claude proposed a model in which TER is dependent primarily on strand number. However, as discussed below, we now know that the TER is also highly dependent on the composition of the tight junction.

Tubular segment | Transepithelial resistance (Ώ.cm 2 ) b | Number of junctional strands (typical range) |
---|---|---|
Proximal | 5–7 | 1–2 |
Thin descending limb of Henle | 700 c | 1 |
Thick ascending limb of Henle | 10–35 | 5–6 d |
Distal convoluted tubule | 350 | |
Collecting duct | 867 | 8–9 |
a Table adapted with permission from Pricam et al.
c Values measured in the rabbit.
d Values from both thick ascending limb and distal convoluted tubule.
Physiological Functions
Gate Function
The gate function refers to the ability to regulate the paracellular permeability. This determines the rate of passive diffusion of extracellular solutes and water between the apical and basolateral aqueous compartments. Early studies, using extracellular macromolecules and lanthanum as electron-dense markers, clearly established the tight junction as the site of the paracellular gate.
In epithelia that mediate active, uphill transcellular transport, such as the collecting duct of the kidney, this gate must act predominantly as a barrier to prevent backleak of solute gradients that have been generated. To do so, these so-called “tight” epithelia must have very low paracellular permeability and conductance (typical transepithelial resistances (TER) range from 1–2 kΏ.cm 2 for the renal collecting duct to ~8 kΏ.cm 2 for amphibian skin ).
In contrast, in epithelia that mediate bulk transport of solutes via the paracellular pathway, the gate is predominantly open. For example, in the late proximal tubule of the kidney about one-third of NaCl reabsorption occurs by the paracellular route, and in the thick ascending limb of Henle, Ca 2+ and Mg 2+ are exclusively reabsorbed via the paracellular pathway. Characterization of the properties of leaky epithelia have indicated that there must be paracellular aqueous pores. Fromter and Diamond were the first to prove that the paracellular leak is physically located at the intercellular junction. Thus, the tight junction is not always a complete barrier, but must also have pores that pass through it.
Fence Function
In epithelia, the lipid and protein components of the plasma membrane are separated into distinct apical and basolateral domains at the tight junction, and this polarity is critical for functions such as vectorial transport. Apical–basolateral polarity is initially established by the Par/aPKC, Crumbs, and Scribble complexes, while the tight junction is primarily responsible for maintenance of this polarity. Specifically, the tight junction behaves like a fence, preventing diffusion within the plane of the lipid bilayer. Interestingly, markers that are confined to the exocytoplasmic leaflet cannot pass across the tight junction, but markers that can flip-flop across the bilayer freely diffuse across it, suggesting that the fence is located in the exocytoplasmic half of the plasma membrane. Several manipulations can dissociate the barrier and fence functions of the tight junction. Transient ATP depletion and depolymerization of the cortical actin network disrupt the paracellular barrier while preserving fence function. This suggests that the fence function of the tight junction is mechanistically independent of its gate function.
Biochemical Composition
The first tight junction-associated protein to be identified was ZO-1, in 1986. Since then, it has become clear that the tight junction is actually a complex of many different proteins. These fall into one of four categories: transmembrane proteins; scaffolding proteins; signaling molecules; and transcriptional regulators. These molecules are associated, through multiple protein–protein interactions, with the scaffolding proteins serving to anchor the complex to the cortical actin cytoskeleton. Table 12.2 lists the known molecules in each category. Here, we focus solely on the transmembrane proteins. These are important because their transmembrane domains traverse the lipid bilayer, while their extracellular domains protrude into the paracellular space. Thus, tight junction membrane proteins are the leading candidates to mediate both gate and fence functions.
Transmembrane proteins | Scaffolding proteins | Signaling proteins | Transcriptional regulators |
---|---|---|---|
Occludin | ZO-1, 2, 3 | aPKC | ZONAB |
Claudins | Par-3, 6 | GEF-H1 | Symplekin |
Tricellulin | Pals1 | Gαi-2, αo, α12 | AP-1 |
MarvelD3 | PATJ | Rab13, 3B | |
JAM | MUPP1 | c-Yes | |
CAR | MAGI-2, 3 | WNK3, 4 | |
JACOP/paracinguilin | CDK4 | ||
Cingulin | |||
AF-6 |
Occludin
Occludin was the first integral tight junction membrane protein to be identified. It has four transmembrane domains with intracellular N- and C-termini. Occludin is ubiquitously expressed in epithelial cells, localizing predominantly at bicellular junctions (junctions where two cells meet). Its role is poorly understood. Overexpression of a C-terminally truncated occludin protein increases TER, but paradoxically increases mannitol flux, and also disrupts tight junction fence function. However, after knockdown or knockout of occludin, cells can still form normally appearing tight junction strands. In fibroblasts that do not have tight junctions, occludin cannot reform tight junction networks, but it does co-polymerize into strands with claudin-1. Knockdown of occludin in MDCK cells causes only a slight increase in permeability to small inorganic and organic cations. Interestingly, occludin null mice have assorted unexplained abnormalities, including retarded postnatal growth, male sterility, and chronic inflammation and epithelial hyperplasia in the gastric mucosa. Importantly, though, epithelial barrier function is normal in these mice. Thus, occludin is neither necessary nor sufficient to form tight junction strands and perform barrier, pore or fence functions. However, occludin can incorporate into claudin-based strands, in which case dominant-negative occludin constructs are presumably able to disrupt normal tight junction function.
Tricellulin
Tricellulin is a homolog of occludin that is localized to tricellular junctions (junctions at the confluence of three cells. It is clearly important for barrier function, because knockdown of tricellulin increases paracellular conductance and permeability to uncharged macromolecules, and its overexpression reduces macromolecular permeability. Mutations in tricellulin cause a form of non-syndromic hereditary deafness, indicating that it plays a critical role in inner ear function, but its role in the kidney has not been explored.
Claudins
Claudins were discovered by Tsukita and colleagues. They are members of a multigene family of ~20–24 kDa tight junction proteins with four predicted transmembrane segments and two extracellular domains (see refs for several recent reviews). When expressed in claudin-deficient cells such as fibroblasts, claudins can form tight junction-like networks of strands, indicating that they polymerize in cis (within the same cell membrane), and can mediate intercellular adhesion, indicating the ability to interact in trans (across two lateral membranes of adjacent cells). Thus, claudins have all the properties expected for a structural component of the paracellular barrier. In 1999, Lifton’s group identified the culprit gene mutated in familial hypercalciuric hypomagnesemia, an inherited disorder thought to be due to failure of paracellular reabsorption of divalent cations in the thick ascending limb of the renal tubule. This gene, which was initially named paracellin, turned out to be a claudin (claudin-16), and its involvement in this disease strongly suggested that claudins play a role in regulating paracellular transport. This is now supported by numerous studies demonstrating that overexpressing or ablating expression of various claudin isoforms in cultured cell lines or in mice affects both the degree of paracellular permeability, and its selectivity (reviewed in ref ). Moreover, Colegio et al. have convincingly shown that the net charge on residues in the first extracellular domain of claudins determines the charge selectivity of paracellular conductance. This suggests fairly strongly that the first extracellular domain of claudins actually forms the lining of the paracellular pore. The permeability properties of different claudin isoforms, as inferred from in vitro overexpression and knockdown studies, are summarized in Table 12.3 .
Pore-forming claudins b | Refs | Barrier-forming claudins | Refs |
---|---|---|---|
Cation-selective pore | Cation barrier | ||
Claudin-2 | Claudin-1 | ||
Claudin-10b | Claudin-3 | ||
Claudin-16 | Claudin-4 c | ||
Anion-selective pore | Claudin-5 | ||
Claudin-4 c | Claudin-6 d | ||
Claudin-10a | Claudin-8 | ||
Claudin-11 c | Claudin-9 d | ||
Claudin-17 | Claudin-11 c | ||
Claudin-14 | |||
Claudin-18 | |||
Anion barrier | |||
Claudin-15 | |||
Claudin-19 e |
a Based on in vitro overexpression or knockdown studies in cultured cell lines. We assume that permeability and selectivity are properties intrinsic to individual claudin isoforms, and ignore the possible confounding effect of heteromeric interactions between isoforms. Claudin-7 is omitted because conflicting data exist suggesting that it is either a Cl-barrier or a Na-barrier/Cl-pore.
b Pore-forming claudins refer to those that predominantly decrease TER or increase solute permeability, while barrier-forming claudins refer to those that predominantly increase TER or decrease solute permeability. The distinction is somewhat arbitrary, since most claudins probably have some finite permeability to most solutes, and the observable phenotype is highly dependent on the properties of the host cell line.
c Acts as a Na barrier in MDCK II cells, but as a Cl pore in LLC-PK1 cells.
d Net flux studies suggest that claudin-6 and -9 may also act as Cl-barriers.
e Conflicting data also exists suggesting claudin-19 can act as a Na barrier.
Functional Domains of Claudin
The functions of several of the domains within the claudin protein have been determined ( Figure 12.6 ). The first extracellular loop of claudin forms the lining of the paracellular pore. Chimera studies have shown that exchanging the first extracellular loop between different claudin isoforms confers different pore selectivity characteristics. Furthermore, point mutations that reverse the charge on residues in the first extracellular loop alter the charge selectivity of the paracellular pore, as would be expected if this domain faced the pore lumen. In claudin-2, a cation-selective pore, this loop specifically contains an aspartate residue (Asp-65) that acts as a Na + -binding site. Several other residues in this domain have also been mapped by cysteine-scanning mutagenesis.

The second extracellular loop of claudin is postulated to participate in trans -intermolecular interactions, acting as the adhesion receptor between neighboring cells. This is based on the finding that mutations of selected residues in the second extracellular loop of claudin-5 abolish its ability to concentrate at cell contact sites when expressed in HEK cells.
Claudins all have a long cytoplasmic C-terminal tail that appears to be involved in protein trafficking and degradation. Deletion of this tail in claudin-5 and -6 leads to accumulation of the protein in the cytoplasm. This domain also affects protein stability, since swapping it between different claudins confers different protein half-lives. The terminal dipeptide motif in claudins (tyrosine-valine in the majority) binds to PDZ domains on the scaffolding proteins, ZO-1, 2, and 3, and MUPP1. However, deletion of this motif does not seem to affect normal trafficking of claudins to the tight junction.
The cytoplasmic C-terminal tail in all claudin isoforms also contains multiple potential phosphorylation sites for a variety of protein kinases (see ref for a comprehensive review). The most common outcome of phosphorylation by protein kinases in this domain is increased assembly at the tight junction, but there are examples of phosphorylation leading to decreased assembly at the tight junction, and also to changes in paracellular permeability.
Finally, all claudins have two pairs of conserved cysteines immediately following the second and fourth transmembrane domains. In claudin-14, these have been shown to be palmitoylated, and this seems to be required for efficient localization to the tight junction.
Physiology of the Tight Junction in Different Nephron Segments
Different nephron segments exhibit different tight junction permeability characteristics that are important for their specific function. These paracellular permeability characteristics are likely a function both of the general structure of the tight junction (discussed above), and of the properties of the specific claudin isoforms expressed in that segment. The nephron segment localization of different claudin isoforms is summarized in Figure 12.7 .
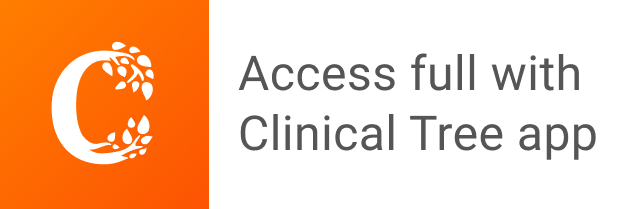