Fig. 18.1
Rendered liver in intraoperative display. In a fully realized abdominal image-guided system such as the one shown here, the relative position of a surgical tool (shown in green) can be interactively displayed along with anatomical structures (red arteries, blue veins, and gray liver parenchyma); lesions (shown in brown) and preoperative planning such as the gold preplanned resection plane (Courtesy of Pathfinder Technologies, Nashville, TN, USA)
Such displays are compelling, they are easy to understand, they can be rotated to the surgeon’s desired or optimal viewpoint, and structures can be displayed or not as required by the case. In addition, they not only provide location but orientation information and depth information via shading. However, such displays are dependent on the validity of the segmentation algorithm used to define the outline of the structures and thus can provide both potential benefit and risk.
Correcting Positional Display Errors due to Perioperative Deformation
In the past, the translation of image-guided surgery techniques to the abdominal environment has been limited due to the presence of perioperative deformation. As a result, the most widely used intraoperative guidance approaches for abdominal have been active imaging with the use of ultrasound (US) or visual laparoscopic imaging. The integration of preoperative imaging and planning data for active intraoperative guidance has only recently begun to be commercialized.
In recent reports, soft tissue deformation during liver resection has been documented with intraoperative computed tomography (iCT) and has demonstrated significant effects [27]. While intraoperative magnetic resonance (iMR) and iCT are available, these approaches are cumbersome, incur radiation dose in the latter, and are not economically scalable for many medical centers. A CT-to-ultrasound (US) vessel-based nonrigid registration system for providing the link between image and physical space has been described [28]. While successful, the OR workflow would seem to be a challenge as it requires the identification of as many vascular targets as possible with tracked ultrasound and then determination of corresponding targets within the CT. While the subsurface information would be valuable for nonrigid deformation correction, there is a significant likelihood of misidentification in highly vascularized organs such as the liver and kidney, and the encumbrance of the technique may challenge adoption.
Given the nature of abdominal procedures, the need to compensate for deformation is evident and the requirements for compensation need to be balanced with workflow and accuracy needs. As an example, presentation for open kidney and liver surgery (and even laparoscopic to a degree) involves significant organ distortion prior to the ability to resect or even collect geometric data. However, considerable exposure of the organ (as opposed to intracranial neurosurgery) is afforded for understanding surgical presentation. In partial nephrectomy, the renal artery and potentially the renal vein are clamped to prevent excessive blood loss during resection. This creates a state of turgor within the organ that is different than the preoperative image counterpart. Upon resection, significant drainage from the cortex and medulla regions can ensue and cause significant shape changes. In both of these examples, the surgical characteristics serve as constraints to data acquisition and guidance procedure execution. As the field of image guidance moves forward, it will be continually evolving to solve new challenges in nonrigid registration.
Over the past several years, approaches to deformation correction for abdominal procedures are being achieved that use sparse intraoperative surface data followed by controlled extrapolative predictions based on computer models [29].
The basic approaches begin with an initial rigid body registration usually performed using traditional [30] or weighted surface registration methods [23]. Once achieved, early correction methods focused at calculating a correspondence between surfaces acquired intraoperatively and their CT/MRI segmented counterparts, and these would be used as boundary conditions in a finite element model derived from the segmented organ in image space [22, 31]. The result could then be used to modify the shape of the CT/MRI organ to match the intraoperative state. While this does provide some improvement, in the “boundary” regions that immediately flank the intraoperative surface, the deformations often look somewhat distorted. In more recent work, efforts to extrapolative methods have been investigated involving iteratively fitting an average shape model to the intraoperatively deformed organ, a shape-based method called the iterative closest atlas (ICAt) technique, and systematically fit a constructed shape by extracting a weighted combination of precomputed shapes [32]. This method precomputes the shapes associated with deformation using a finite element model which allowed for rapid registration intraoperatively. While preliminary results were encouraging, the atlas shape models were challenging to generate for surgical data. There are still powerful aspects to this work we are investigating and such methods proposed must be thoughtfully designed with respect to surgical workflow and speed of translation. Solutions that do not accommodate workflow and are overly encumbered require too much attention from the surgeon and as a result are not easily adopted.
One of the noted drawbacks to an ICP-driven surface registration is that they are sensitive to rotational symmetries and biology tends to create smoothly curved surfaces. Thus, an ICP-driven surface registration can “slide” to incorrect location reducing the robustness of the registration methodology. To address this issue we elected to capture rough designations of important and identifiable “salient” features such as the renal hilum. In the clinical open liver surgery, “salient features” were weighted with the surface to add information to the registration process. Mean error dropped from 24 to 3.6 mm and the standard deviation from 23 to 1.0 mm [23].
Intraoperative Image-Guided Kidney Partial Nephrectomy
Bringing image guidance to the kidney poses some specific challenges. Recent studies have demonstrated that a partial nephrectomy, either open or MIS, is an effective procedure for renal cell carcinoma and is especially applicable for tumors less than 4–7 cm. In addition to providing equivalent oncologic outcomes, improved patient morbidity and mortality, as compared to complete kidney removal, have been noted. Nephron-sparing procedures are imperative when the contralateral kidney is functionally impaired or has been surgically removed in many cases.
Unlike any previous image-guided surgery target, the kidney is covered in perirenal fat, which hinders access to the surface of the kidney and to localization of specific anatomical surface targets. The fat also results in mechanical coupling to the abdomen and diaphragm resulting in significant movement with respiration. Since a significant fraction of kidney tumors protrude from the kidney, “locating” the tumor is often not the challenge. Rather it is the localization of the parenchymal deep margin point of the tumor while maintaining a clear surgical field, avoiding excessive blood loss, and performing meticulous repair that is the surgical challenge. Hopefully, by interactively showing the surgeon the location of his or her tools during the surgery in relationship to the critical deep tumor, vascular and collecting system image guidance could minimize excess nephron removal and risk of positive margin and aid in identification of critical structure for avoidance if possible or repair if needed.
Preliminary Data
We have conducted a number of preliminary studies using a porcine animal model. Swine were chosen as their kidneys closely approximate the size and structure of human kidneys. The first experiment assessed the effect of the loss of perfusion associated with standard kidney vessel clamping and a body force similar to the CO2 insufflation pressure in an MIS application.
The kidneys were obtained from anesthetized or newly euthanized pigs under an IACUC-approved protocol. Heparin was administered intravenously to prevent blood clotting, and the renal artery and vein were closed to retain turgor before resection. Between 15 and 20 glass beads with 2 mm radii and holes through the center were sutured onto the kidney surface in a roughly even distribution over the entire kidney. CT scans of the kidney (160 or 300 mAs, 90 keV, 0.8 mm slice spacing, Philips human CT scanner) were taken before and after the renal artery and vein were cut and the kidney decompressed. We then assessed the kidney changes due to fluid loss by comparison of the CT scans and by tracked fiducial location (Fig. 18.2).
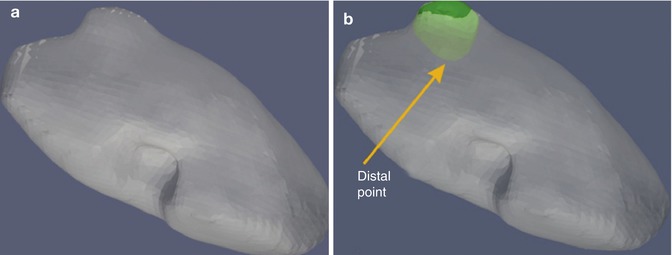
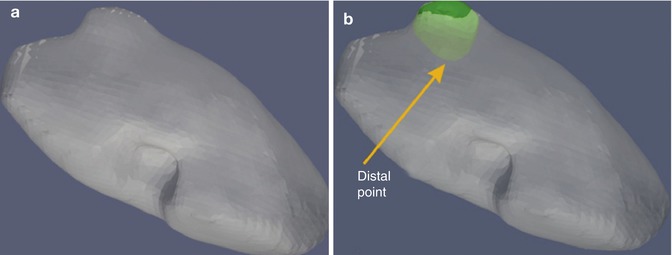
Fig. 18.2
(a) External segmented kidney surface from CT scan – note bulge lateral of tumor from surface. (b) External segmented kidney surface showing colored segmentation of tumor margin (green) and internal deep margin location of tumor from the surface (yellow arrow) (From Galloway et al. [26], with permission)
We performed similar experiments incorporating the effect of turgor loss due to an incision. In Fig. 18.3, the incision can be seen. We placed 30 fiducials distributed across the surface of the kidney. Fiducial motion ranged from near zero to 1.1 cm. After applying a correction algorithm, the errors drop dramatically showing a maximum of 0.8 cm at the site of incision but a mean of less than 2 mm. It should be noted at this point that the model is not designed to deal with incisions; therefore the residual error at the opening is to be expected.
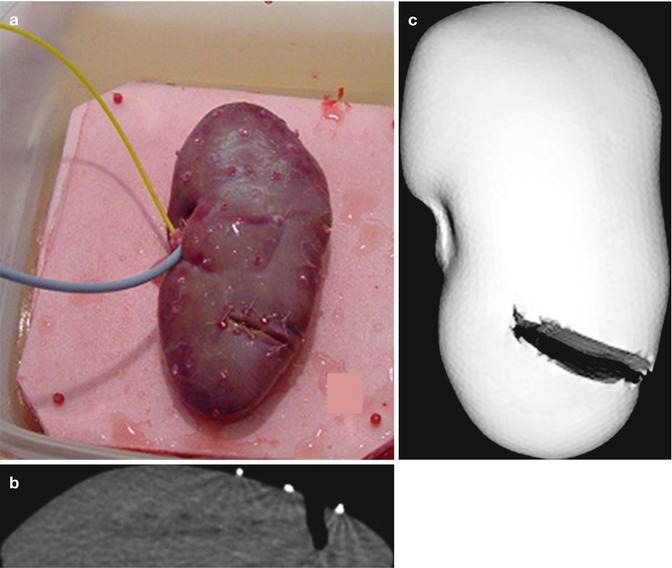
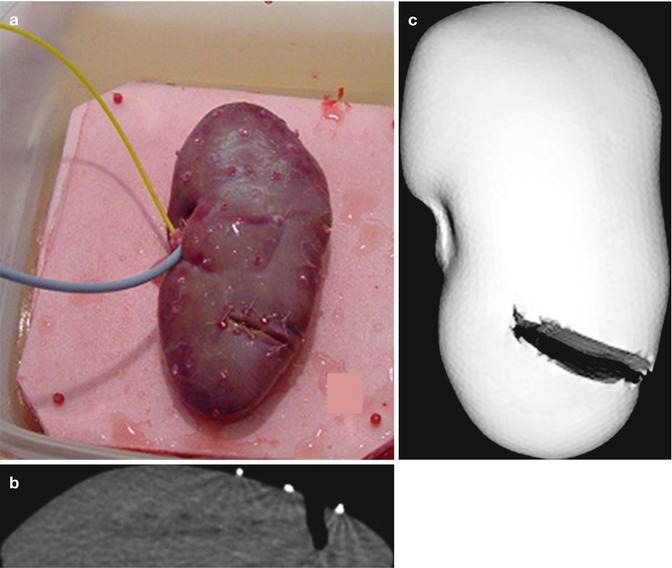
Fig. 18.3
Measuring deformation in the incised kidney. Kidney turgor is maintained by saline perfusion and drainage via catheters. (a) Resected and saline perfused kidney and the fiducial positions (red beads sutured on surface). (b) Subtraction CT scan showing the depth of the incision and bead fiducials (white). (c) CT rendering of the surface and incision from the full CT scan (From Galloway et al. [26], with permission)
Human Studies: Preliminary Data
We have progressed to human studies for kidney guidance. In the human while we get the additional challenge of the perirenal fat, we gain an advantage in that a significant number of human kidney tumors are exophytic. This provides a change in surface curvature (a salient feature) that is ideal for surface registrations (Fig. 18.4).
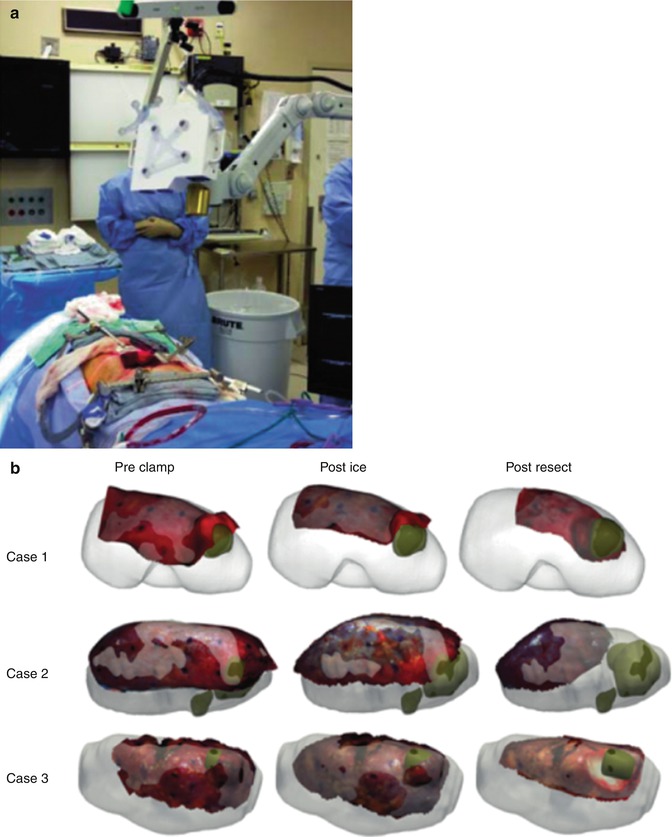
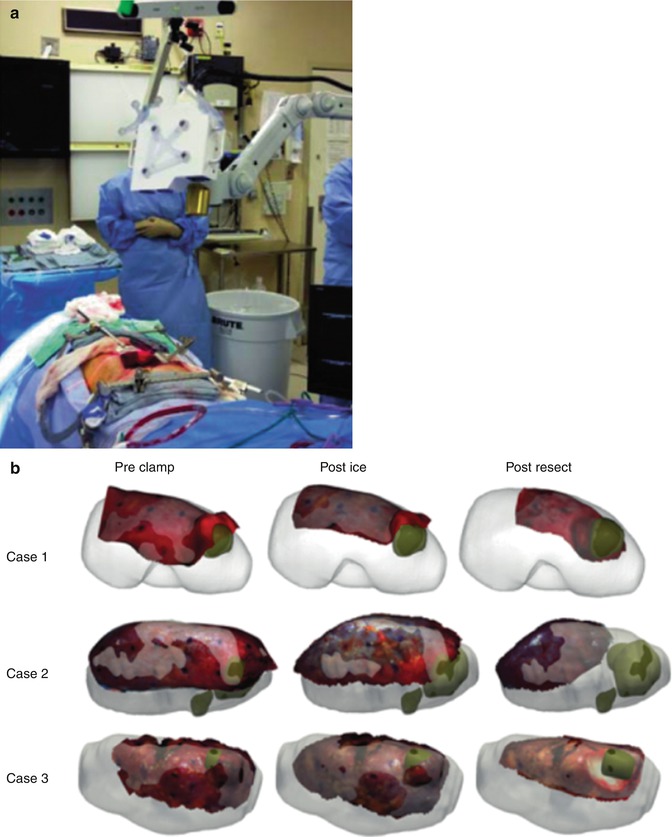
Fig. 18.4
Laser range scanner capturing kidney surface during open partial nephrectomy case. (a) Laser range scanner unit with optical tracking deployed for IRB-approved intraoperative data gathering (no active navigation) in OR during partial nephrectomy cases. (b) Post hoc registered laser range scanner surfaces (red) shown over corresponding preoperative segmented computed tomography surfaces (white), tumors (green). Scans were obtained before clamping and were registered via surface-based methods, while subsequent scans were registered to preclamp by “virtual fiducial” point-based methodology
In our preliminary human studies, we obtained LRS surfaces of a kidney during open nephron-sparing surgical procedures. Since we cannot place extrinsic objects in the kidney prior to surgery, we implemented a unique new solution. Once the kidney was exposed, six surface marker dots were placed on the kidney using a surgical marker. We then performed an LRS scan of the kidney. As the LRS obtains a color image which can be mapped onto the 3D surface, the dots can be localized as “virtual fiducials.” The kidney was clamped and iced as a standard step, and after 10 min a second LRS scan was obtained. Finally, the surgery proceeded and the tumor was resected. A final LRS scan was obtained. Our results were encouraging. After clamping and icing the mean TRE for the virtual fiducials is 0.95 mm (max = 1.33 mm). After the resection, the surface had a significant resection crater which impeded the surface registration (mean TRE 7.33 and 9.53 mm max). However, we know that an accurate registration could still be performed even after the resection due to the use of the virtual fiducials in a point-based registration (Fig. 18.5).
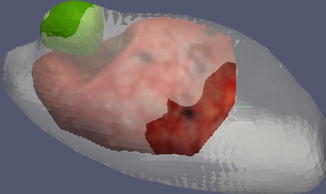
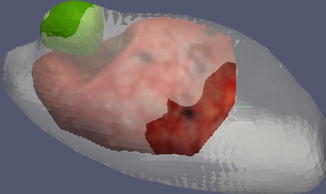
Fig. 18.5
Postresection display showing former tumor location (green), the LRS surface (red), and the preoperative CT (gray)
Robotic Image-Guided Surgery (RIGS): Vanderbilt University Experience
The current robotic da Vinci technology platform (Intuitive Surgical, Sunnyvale, CA, USA) functions as an “enabling” technology, allowing multiple arm/camera control and intricate maneuvers such as suturing, thus allowing many laparoscopic naïve and even advanced MIS surgeons to perform more complex operative interventions. Starting in 2003, we sought to incorporate the potential benefits of IGS as an “augmenting” technology in combination with the robot’s spatial accuracy, tracking, steadiness, and informational data input. We selected the partial nephrectomy procedure with its challenges (hemostasis, ischemia limits, oncologic margins, deformation, etc.) and potential benefits (high-resolution preoperative CT imaging, need for renal functional preservation).
Initially we analyzed the potential for using both intrinsic and extrinsic tracking and a variety of localizers after characterizing the kinematic chain and tracking capabilities of the da Vinci [33]. The desire to place multiple arms in the coordinate space resulted in a hybrid tracking approach incorporating optical tracking (Polaris Spectra, Northern Digital Inc., Waterloo, Canada) and intrinsic localizers in the “robot” [10]. Analysis showed acceptable error (<2 mm).
The RIGS system was then validated in a simulated surgical task of subsurface lesion resection from a gel phantom using both standard visual and IGS augmented guidance, showing benefit in improved resection metrics [9].
Intraoperative performance of surface acquisition with da Vinci during IRB-approved bystander study revealed acceptable registration error estimations (mean closest point distance 1.4 mm) [25]. While surface capture through a tracked tool is feasible, error and the lack of rapid ability to recapture and reregister have led us to explore alternatives (Fig. 18.6).
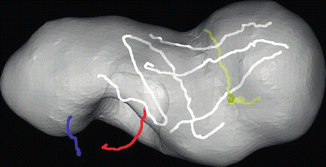
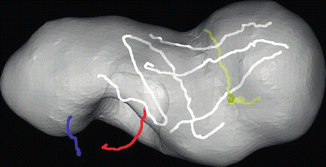
Fig. 18.6
Surface capture of the kidney registered to segmented computed tomography of kidney and mass. White lines represent surface tracking done with tracked da Vinci tool tip obtained actively during partial nephrectomy case; blue and red represent hilar structures. Gray surface model represents segmented kidney surface from preoperative computed tomography scans including large lower pole tumor (right side of figure). Gold line represents held out data for registration accuracy. Mean closest point distance 1.2 mm
A common method of noncontact scanning is the use of a laser range scanner (LRS). LRS systems work by triangulating the location of a projected laser point. With the knowledge of the direction of projected laser light and an observation of where it appears in a camera image, one can determine the location of the illuminated point in 3D space. Rapidly panning the laser allows the LRS to acquire a large number of points without moving the sensor physically. LRS has been applied to intraoperative surgical registration during open approaches, but is not compatible or available for an MIS environment at present.
We have incorporated the LRS into complex open partial nephrectomies and a new composite rapid point registration “virtual fiducials” methodology has been developed. Data regarding “real-case” operative deformations is being gathered and analyzed for predictive modeling [20].
We are investigating and characterizing technology known as conoscopic holography to provide rapid and accurate surface capture and registration (Fig. 18.7) [34]. The conoprobe tracks using an optical tracking system and provides distance measurements to points on the tissue. As the laser point is traced over the organ surface by appropriate manual manipulation, a cloud of surface points on the organ is produced. The principles by which conoscopic holography measures distance were proposed by Sirat and coworkers [35]. The system illuminates an area of interest with a collimated light source. The cone of light returning from the illuminated area is filtered and then enters a birefringent crystal. Inside the crystal, constructive and destructive interference occurs, which results in a Fresnel pattern from which distance can be deduced. Conoscopic holography is currently used for distance measurement in industrial profilometers, where it is combined with a motorized X–Y stage. This enables highly accurate measurements of dimensions on machined components and is useful for manufacturing process control.
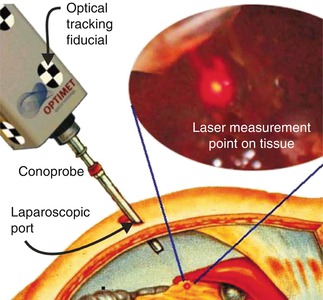
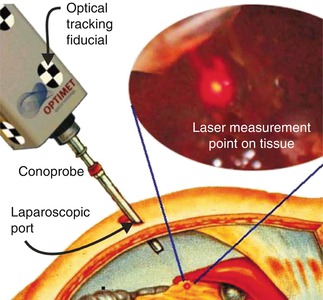
Fig. 18.7
Conceptual art of MIS access scanning of solid intra-abdominal organ via conoscopic holography. The tracked conoprobe returns distance measurements, which are converted to a point cloud that defines the shape of the tissue surface (From Lathrop et al. [34], with permission)
Working with us in our engineering school, Lathrop and Webster have described experiments exploring the feasibility of using a conoscopic holography-based scanner as a method for obtaining minimally invasive surface scans for soft tissue registration [34]. A scanning system based on conoscopic holography promises the ability to scan through a laparoscopic port without requiring wide exposure of the organ of interest. The facts that conoscopic holography is a proven technique for high-precision distance measurements, the underlying device is readily available commercially in inexpensive packages, can be adopted for use in a minimally invasive sterile manner, and can deliver high-quality distance measurements to biological tissues, making it a compelling technology for laparoscopic surface scanning.
We recently conducted experiments examining the potential use of a conoscopic holography unit adapted for use in the OR. Moving this technique from concept to clinical use requires a rigorous accuracy evaluation, which is the purpose of our paper [36]. We adapted recent nonhomogeneous and anisotropic point-based registration results to provide a theoretical framework for predicting the accuracy of tracked distance measurement systems. Experiments conducted were complex objects of defined geometry, an anthropomorphic kidney phantom, and a human cadaver kidney (Fig. 18.8). Experiments agree with model predictions, producing point RMS errors consistently of <1 mm, surface-based registration with mean closest point error of <1 mm in the phantom, and an RMS target registration error of 0.8 mm in the human cadaver kidney. Tracked conoscopic holography is clinically viable; it enables minimally invasive surface scan accuracy comparable to current clinical methods that require open surgery.
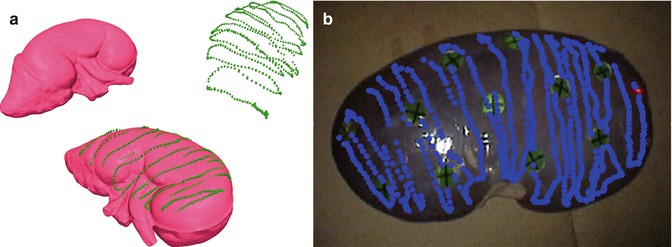
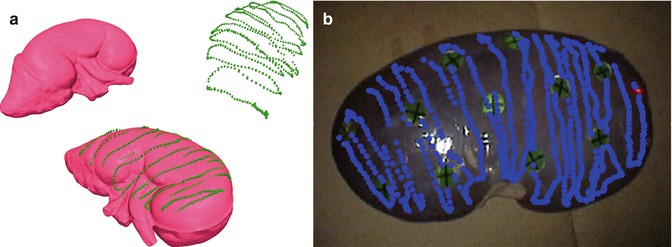
Fig. 18.8
(a) Surface model of the anthropomorphic kidney phantom in the image coordinate system I (left) and the conoscopic surface measurement acquired in the patient coordinate system P (right). After successful surface-based registration, the measured point can be transformed into the image coordinate system using (bottom) (b) scanned conoprobe points (blue dots) taken from an excised human kidney. The circles with crosses serve as assessment fiducials (a, From Burgner et al. [36], with permission)
Our work provided a comprehensive accuracy evaluation and experiments with an optically tracked conoscopic holography sensor for application in image-guided surgery. The system acquires 3D surface measurements of objects of medical interest, which can be used to register preoperative images to anatomy. Our accuracy evaluation experiments show RMS errors that are consistently <1 mm for point measurements. Surface-based registrations performed using the system show a mean closest point error of <1 mm after registration. The average RMSTRE in our human ex vivo cadaver kidney trials was 0.8 mm. These results suggest that conoprobe-enabled surface scans can be useful in extending surface-based registration techniques to the MIS setting for image-guided surgery; they enable accuracy comparable to the existing methods used in open surgery such as LRS. We are currently investigating surface acquisition with the conoprobe in place of the laser range scanner in our deformation correction framework. Thus, this approach has the potential to bring the advantages of image guidance to minimally invasive human surgeries in the near-term future.
< div class='tao-gold-member'>
Only gold members can continue reading. Log In or Register a > to continue
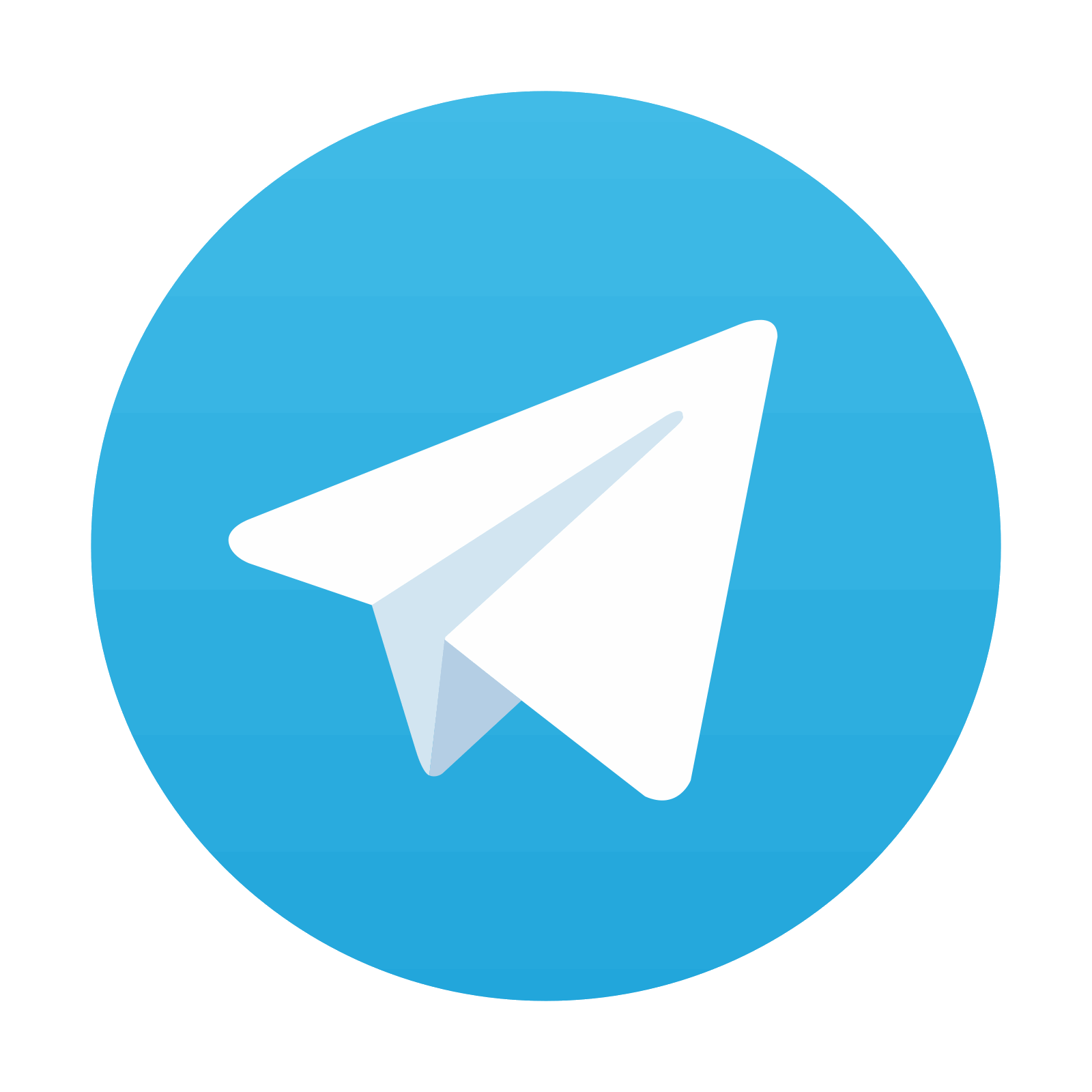
Stay updated, free articles. Join our Telegram channel
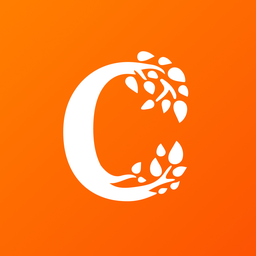
Full access? Get Clinical Tree
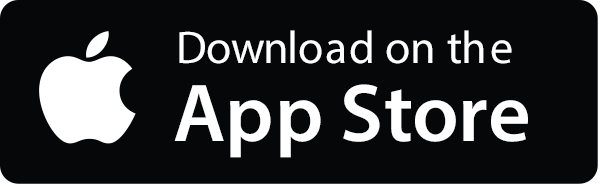
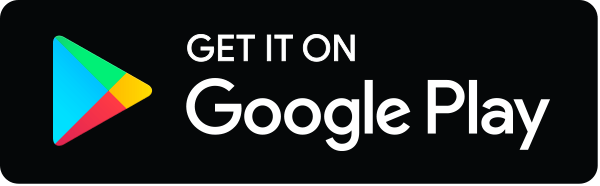