Hepatocellular Carcinoma: Molecular Biology and Genetics
Michael C. Kew
Introduction
Hepatocellular carcinoma (HCC) is the most common primary malignant tumor of the liver and is regarded as one of the major malignant diseases in the world today. Among the reasons for this view are its high incidence, limited treatment options, and grave prognosis. HCC is either the most common tumor or among the three most common tumors in many of the most populous regions of the world, and it is fifth in overall frequency (1). It ranks third in annual cancer mortality rates (1), being responsible for >500,000 deaths each year. Attesting to the poor prognosis of HCC is the observation that in Black African and Chinese populations, which have the highest incidences of the tumor, the annual mortality rate from HCC is virtually the same as its annual incidence (2). Another reason for the importance of the tumor is that, unlike most types of malignant disease in humans, the majority of the risk factors for HCC have been identified, allowing substantial progress to be made in its primary prevention.
The incidence of HCC differs considerably in different geographic regions (1,2,3), as do the major causes of the tumor. In industrialized Western countries, usually with a low but sometimes an intermediate incidence of HCC, the major etiologic associations are alcoholic cirrhosis and chronic hepatitis C virus (HCV) infection; in Japan, with a high incidence of the tumor, chronic HCV infection is the predominant risk factor; and in eastern and Southeast Asia and sub-Saharan Africa, regions that have the highest incidences of HCC, chronic hepatitis B virus (HBV) infection, sometimes in association with heavy dietary exposure to the mycotoxin, aflatoxin B1 (AFB1), is responsible for approximately 80% of HCCs. HBV and HCV, between them, account for 70% to 85% of global HCC. Less frequent causes of the tumor, such as obesity/diabetes/nonalcoholic steatohepatitis (NASH), some of the inherited metabolic diseases, dietary iron overload in the African, and membranous obstruction of the inferior vena cava, may also occur predominantly in certain populations or regions.
HCC is proving to be heterogenous in its molecular genesis, with appreciable differences depending on the cause of the tumor. Moreover, the multiple and complex pathogenetic mechanisms involved, how they interact, and the sequence in which they occur are still poorly understood. The absence of a unifying explanation for hepatocarcinogenesis at a molecular level is limiting attempts both at designing effective treatments and at secondary and tertiary prevention of the tumor.
Regardless of the etiologic agent or agents involved, hepatocarcinogenesis is a complicated multistep process with the essential components being DNA mutations or damage and dysregulated hepatocyte proliferation in the face of reduced cell death. As a result of the linear and progressive process, increasingly more aberrant monoclonal populations of hepatocytes evolve and progress ultimately to a fully malignant phenotype. Several oncogenic mechanisms are involved, including loss of tumor-suppressor gene function, protooncogene activation, direct and indirect viral effects, changes in DNA methylation, failed DNA repair, telomerase activation, and angiogenesis. These events interact with host factors, such as immune responses and the hormonal milieu, in initiating and furthering the transition from normal to malignant hepatocytes. Local invasion and metastasis are related events.
Current knowledge of the molecular genesis of HCC is reviewed in relation to the more common or important causal associations of the tumor.
Molecular Genesis of Hepatocellular Carcinoma
Chronic Necroinflammatory Hepatic Disease
With almost all known causes of HCC—namely, chronic hepatitis virus infections, habitual alcohol consumption, diabetes/obesity/NASH, hereditary hemochromatosis and African dietary iron overload, α-1-antitrypsin deficiency, glycogen storage disease, hereditary tyrosinemia, hypercitrillinemia, Wilson disease, primary biliary cirrhosis, and membranous obstruction of the inferior vena cava—and in most geographic regions, the majority of the tumors occur in association with chronic necroinflammatory hepatic disease, commonly in the form of cirrhosis (4,5), occasionally chronic hepatitis (4,5), and rarely reversed hepatic lobulation complicating hepatic venous outflow obstruction (6). Moreover, all forms of cirrhosis, whatever their cause, may be complicated by the development of HCC (4,5,7). The tumor is also the major cause of liver-related deaths in patients with compensated cirrhosis (4,8). Chronic necroinflammatory hepatic disease is therefore the most common etiologic association of HCC worldwide.
Although chronic necroinflammatory hepatic disease may act alone in hepatocarcinogenesis, far more often it acts in concert with other known or not yet identified causes of the tumor, as shown by the observation that the frequency with which
HCC complicates cirrhosis ranges from as high as 30% with chronic HCV infection to as low as 4% with Wilson disease and primary biliary cirrhosis (4). Apart from the additional risk factors’ inherent potential to induce malignant transformation, they are usually also the cause of the chronic hepatic inflammation and necrosis.
HCC complicates cirrhosis ranges from as high as 30% with chronic HCV infection to as low as 4% with Wilson disease and primary biliary cirrhosis (4). Apart from the additional risk factors’ inherent potential to induce malignant transformation, they are usually also the cause of the chronic hepatic inflammation and necrosis.
Constitutive (unrestrained) proliferation of hepatocytes is one of two essential components of hepatocellular carcinogenesis (9), the other being a series of mutations or changes at the genetic or epigenetic level. Cell cycle activation in the genesis of the tumor can be directly triggered by overexpression of a single gene or combinations of genes, or be initiated indirectly by compensatory proliferation of hepatocytes in response to injury (9). Hepatocytes are normally in a quiescent state, with a very low turnover rate (the estimated half-life of hepatocytes is 6 months [10]), but they react to loss of liver cells with an extraordinarily vigorous proliferative response (11,12). This response is normally short lived and tightly controlled, and does not lead to malignant transformation. Proliferation of hepatocytes is regulated by a number of factors, including nuclear factor-κB (NF-κB), transforming growth factor-α (TGF-α), insulinlike growth factor-2 (IGF-2), hepatocyte growth factor (HGF), and HGF receptor met (13,14,15). Transcriptional activation of these factors by proinflammatory mediators such as tumor necrosis factor-α (TNF-α), chemokines, and interleukins released during the inflammatory process regulates proliferation (13,14) and has, in addition, an antiapoptotic effect through upregulation of antiapoptotic target genes (15). With continuous hepatocyte proliferation, regardless of the initiating cause, the regulated proliferation, at some point and for reasons as yet poorly understood, may become unrestrained (9). A proliferative response with a change in the hepatocyte from a quiescent to a constitutively replicating cell is obligatory for the initiating activity in hepatocarcinogenesis (9,16).
Chronic necroinflammatory hepatic disease, irrespective of its cause, is characterized by continuous or intermittent necrosis of hepatocytes followed by regenerative proliferation that can, in certain circumstances, trigger the conversion of regulated proliferation to unconstrained proliferation. Rates of proliferation always exceed rates of apoptosis (13) so constitutive hepatocyte proliferation, in association with the accumulation over time of a number of DNA mutations or changes, results in the formation of hyperplastic hepatocyte nodules that progress to dysplastic nodules and finally to HCC (9). The exact timing of the original genetic or epigenetic change in relation to the onset of constitutive hepatocyte proliferation is uncertain, but thereafter the accumulation of successive mutations or damage occurs during the ongoing proliferation.
Existing quiescent hepatocytes are the cells mainly responsible for proliferation (9,12). Indeed, these cells have a proliferative potential that may exceed that of stem cells. They also appear to escape the senescence expected after several rounds of replication (17). Only uncommonly do oval cells (bipotential liver cells able to differentiate into either hepatocytes or bile duct epithelium cells) directly give rise to tumor cells, although it is possible that hepatocytes originating from oval cells could be at greater risk of oncogenic transformation than other hepatocytes (9).
By increasing the hepatocyte turnover rate, chronic necroinflammatory hepatic disease greatly enhances the risk of a cell being initiated and progressing to a malignant phenotype. At least three putative mechanisms are involved. Resting hepatocytes do not undergo mutation (18). However, spontaneous mutation or exogenously induced DNA damage or mutation may occur when quiescent hepatocytes enter the cell cycle (18) and are therefore prone to occur during hepatocyte proliferation. A variety of DNA repair mechanisms normally protect cells from mutations and damage (19). However, an accelerated rate of hepatocyte turnover allows less time for mutated, damaged, or rearranged DNA to be repaired before the cell divides again, thereby “fixing” the abnormal DNA in the daughter cells. For this and a number of other reasons, the failure of DNA repair processes facilitates the accumulation over time of a series of mutations. Progressive accumulation of mutations, rather than a single critical mutation, is an essential prerequisite for cancer formation (20,21). If cells harboring these mutations escape elimination by apoptosis or the host’s immune response, they may over time become fully transformed. Unconstrained hepatocyte proliferation also provides an opportunity for the selective growth advantage of initiated cells to be exercised and allows clonal expansion of these cells, a crucial step in tumor promotion and progression (20,21).
Another contributor to the persistence and growth advantage of initiated cells is reactivation of telomerase, a ribonucleoprotein enzyme that prevents the shortening of telomeres (22). During progression of chronic hepatitis to cirrhosis, progressive shortening of telomeres occurs as a result of multiple cycles of cell injury, death, and regeneration, leading to premature senescence of hepatocytes (22). Telomere shortening beyond a critical length causes a proliferative block, manifesting as chromosomal instability, end-to-end fusions, and cell death (22). Hepatocarcinogenesis is characterized by the evolution of clones of hepatocytes with increased telomerase expression and an immortalized phenotype (22). Thus, almost all HCCs show reactivation of telomerase activity.
Concurrently with these various oncogenic mechanisms, the development of hepatic fibrosis distorts the lobular architecture of the liver, modifying normal cell-to-cell and cell-to-extracellular matrix interactions, which may contribute to loss of cell growth control (23). Furthermore, changes in the microcirculation of the liver, secondary to the disturbed architecture, may impair DNA repair processes and channel oncogenic substances to selected groups of hepatocytes (24,25).
In addition to providing the stimulus to constitutive proliferation of hepatocytes, chronic necroinflammatory hepatic disease can cause the mutations and DNA damage that are also required for oncogenesis. Oxidative stress induced by overproduction of reactive oxygen species (ROS) and reactive nitrogen species, in tandem with an unregulated increased hepatocyte turnover rate, plays an important role in the initiation and progression of carcinogenesis (26,27). Chronic necroinflammatory hepatic disease generates ROS (26,27). Putative mechanisms of free radical–induced hepatocyte damage and malignant transformation are the mutagenic properties of the radicals and their effect on lipid peroxidation. Different forms of oxidative damage cause different mutations, including chromosomal translocation, and modify gene expression in different ways. DNA strand breaks may also be induced (28). Deoxyguanosine residues in DNA are hydroxylated at the C8 position by hydroxyl radical or singlet oxygen to form 8-hydroxy-2-deoxyguanosine (8-OH-dG). Misreading of 8-OH-dG results in guanine (G) to thymine (T) transversions that may be important in carcinogenesis (29). Peroxidative decomposition of membrane polyunsaturated fatty acids releases reactive aldehyde metabolites, such as malondialdehyde (MDA), which may impair cellular functions, including nucleotide and protein synthesis, and damage intracellular organelles (30,31). The proteins affected include transcriptional regulators, such as fos, jun, and NF-κB, as well as components required for the activation of the antioxidant response element. Another biogenic aldehyde derivative, 4-hydroxy-2′-nonenol (4-HNE), interacts with DNA to form exocyclic guanine products, as shown by the significantly increased 4-HNE-deoxyguanine product in hepatic tissue in HCC (32).
The other recognized etiologic associations of HCC may either act in association with chronic necroinflammatory hepatic disease or independently.
Chronic Hepatitis B Virus Infection
HBV was one of the first viruses to be causally linked to a human tumor, and it is now believed to be, with tobacco, the most important environmental carcinogen to which humans are exposed. Of the estimated 350 million carriers of this virus in the world today (about 5% of the global population) or as many as one-fourth, will develop HCC. HBV infection acquired early in life is likely to become chronic and is implicated in the development of the great majority of HCCs that occur with high frequency in Chinese and Black African populations. However, the virus plays a far lesser causal role in industrialized countries. HBV is estimated to be responsible for approximately 53% of global HCC (1). The different genotypes of the virus have different hepatocarcinogenic potentials. In Far Eastern countries, where genotypes B and C predominate, a greater risk of tumor formation has been found with genotype C, and in southern Africa, where genotype A constitutes approximately 75% of the isolates, the risk of HCC development is 4.2 times higher with this genotype (33).
An effective and safe vaccine against HBV has been available for some years, and universal immunization of newborns in those countries in which the virus is endemic has already resulted in striking decreases in the carrier rates. Furthermore, in those endemic countries where universal immunization has been practiced for the longest times, HCC rates among children have already declined by as much as 70%. HBV vaccine can legitimately be regarded as the first “anticancer vaccine.”
Many, perhaps even most, HBV-related HCCs coexist with cirrhosis and a few with chronic hepatitis, indicating that chronic necroinflammatory hepatic disease resulting from the host’s immune response to the ongoing presence of the virus plays a key role in the pathogenesis of the tumor. HCC develops in 2% to 7% of patients with HBV-induced cirrhosis each year, but less often in those without cirrhosis. Given the seminal role of constitutive proliferation of hepatocytes in hepatocarcinogenesis, even in those patients with normal or near normal livers at the time of presentation of the tumor, a period of ongoing hepatocyte necrosis and regeneration may have occurred earlier, initiating the unconstrained proliferation of those cells that later became transformed. The necroinflammation could subsequently have resolved with little or no residual histologic changes. Chronic necroinflammatory hepatic disease is thus often an integral component of the hepatocarcinogenic potential of HBV.
Support for this belief is provided by the consequences of introducing HBV preS/S gene into transgenic mice. These mice overproduce large envelope (preS1) protein, which accumulates in the endoplasmic reticulum (ER) of hepatocytes, resulting in severe injury to the cells and initiating a response characterized by inflammation, oxidative DNA damage, regenerative hyperplasia, transcriptional deregulation and aneuploidy, and progressing ultimately to neoplasia (34). These findings imply that severe and prolonged hepatocyte injury, caused by the presence of the viral protein, ultimately lead to malignant transformation. The same series of events could be triggered by a relatively inefficient immune response by the host to the presence of HBV, resulting in some infected hepatocytes undergoing necrosis without downregulating overall HBV gene expression (35,36).
Possible pathogenetic mechanisms of HCC developing in a cirrhotic liver have already been mentioned, but additional mechanisms peculiar to HBV-associated HCC exist. HBV DNA does not integrate into cellular DNA in resting hepatocytes (34). However, the frequent cell divisions that occur in chronic necroinflammatory disease create the opportunity for HBV DNA to be inserted into host DNA. Moreover, oxidative DNA damage caused by chronic virally induced inflammation induces both DNA strand breaks and DNA instability that further increase the likelihood of integration events (37). In addition, enhanced intracellular activity of topoisomerase 1, a nicking/closing enzyme that reduces tension between the strands of DNA in supercoiled DNA resulting from hepatocyte proliferation, may predispose to insertion of HBV DNA by cleaving the viral genome at specific motifs, linearizing the circular DNA, and promoting its integration into chromosomal DNA (38).
Substantial evidence has accumulated that HBV has inherent potential to induce malignant transformation in addition to its ability to cause hepatic inflammation and necrosis. Observations that both woodchucks chronically infected with woodchuck hepatitis virus (WHV), which shares with HBV membership of the Hepadnaviridae, and transgenic mice into which the HBV x (HBx) gene with its regulatory sequences had been introduced develop HCC in the absence of cirrhosis support an inherent carcinogenic potential of the virus (39,40). Multiple factors appear to contribute to this potential, but the role of each and how they interact remains uncertain. The finding that HBV DNA was integrated into chromosomal DNA in the great majority of HBV-related HCCs (41,42) pointed to a number of putative mechanisms. HBV does not contain a recognized oncogene, but the presence of HBV DNA integrants in cellular DNA is consistent with the mechanism described for nonacutely transforming viruses, namely, insertional mutagenesis. This mechanism may take a number of forms.
Deletion, translocation, duplication, or amplification of chromosomal DNA in the sequences flanking integrated viral DNA occur far more often in HBV-related HCC than in HCC attributable to other risk factors (43,44). These changes may result in increased genomic instability, as well as in loss of tumor-suppressor genes, changes in the physical relation between protooncogenes or tumor-suppressor genes and their regulatory sequences that may perturb the expression of these genes in such a way as to contribute to malignant transformation, or the translocation of a protooncogene into a constitutively expressed gene.
HBV DNA integration precedes the development of HCC (45). Insertion occurs at one or, far more often, multiple sites. Viral DNA may integrate as a single linear sequence (almost always with some nucleotides missing from one or both ends of the insert [42]), but more usually it comprises contiguous fragments of viral sequences rearranged as a result of the oxidative damage caused by the chronic necroinflammatory hepatic disease (37,40,42). Insertion into host DNA appears to take place at random sites (although repeat Alu or satellite III DNA sequences are often targeted). However, allelic losses occur more often in certain chromosomes (1p,4q,6q,8p,9p,13q,16p,16q,17q), and essentially the same chromosomes harbor changes in DNA copy numbers (46), suggesting that these regions contain genes often involved in hepatocarcinogenesis. Early data suggested that insertion of HBV DNA into chromosomal DNA at a site that may directly induce transformation (i.e., cis activation) was rare (47). However, more recent studies have shown HBV DNA to be integrated not infrequently in genes encoding for proteins that are important in the control of cell signaling, proliferation, and viability (48,49). Moreover, the telomerase gene has been shown to be targeted, and this might also be true of genes regulating calcium homeostasis and mitogen-activated protein kinase (MAPK)–dependent signaling, some of which have not previously been known to be involved in malignant transformation (48).
A cis-acting mechanism does, however, appear to be important in WHV-induced HCC in woodchucks. In 50% of infected animals, integration of WHV DNA occurs in the vicinity of N-myc-2 retroposon or MYC, considerably enhancing transcriptional activity of the promoters of these genes (50). Moreover, transgenic mice in which the WHV MYC gene, in tandem with
upstream WHV DNA, has been incorporated into the germline, develop HCC (51).
upstream WHV DNA, has been incorporated into the germline, develop HCC (51).
The most common sites of HBV DNA insertion in the virus are in the cohesive overlap region between direct repeat sequences1 and 2 (DR1 and DR2) (52). This clustering suggests that intermediates of HBV replication serve as the substrates for integration (53). Strand invasion of chromosomal DNA by single-stranded linear HBV DNA is believed to initiate recombination.
Trans-activation by HBV proteins of cellular genes remote from the site of integration, thereby influencing cellular proliferation and differentiation, or apoptosis, is a more frequent mechanism of inherent oncogenesis (47,50,54). This effect could be mediated through signal conduction pathways. Two HBV genes, HBx and the preS2/S when 3′ truncated during or after integration, have been shown to have transactivating capability (54).
Several lines of evidence support a role in the transformation process for deregulated HBx protein expression from integrated fragments of HBV DNA (55). Because the gene is close to the preferred insertion sites of HBV, it is the region of the genome most often included in integrants (56), and a selective accumulation of HBx gene transcripts has been reported in HBV-related HCC. Moreover, integrated HBx, even when truncated, frequently encodes functionally active transactivator proteins and may overexpress these proteins (56). Viral sequences encompassing the RNA encapsidation signal (which overlaps HBx) might also have intrinsic recombinogenic activity by virtue of binding to a putative recombinogenic cellular protein (57). The possible hepatocarcinogenic role for HBx protein is supported by a number of in vitro and animal model studies (51).
HBx protein does not contain any structural motifs that indicate a capacity to bind DNA directly. The 17-kDa protein is mainly a cytoplasmic protein, although there is also evidence for nuclear localization. HBx protein constitutively activates transcription from HBV enhancers and promoters, as well as from promoters of a number of cellular genes involved in the control of cell growth and proliferation, including protooncogenes, growth factors, and cytokines (58,59,60,61). Activation of transcription is mediated by modulating cell signaling pathways within the cytoplasm. The pathways activated include MAPK, the Janus family tyrosine kinase (JAK)/signal transducer and activators of transcription (STAT), Src, protein kinase C (PKC), activator protein-2 (AP-2), and jun-N-terminal kinase (58,59). Activation of JAK/STAT and Src signaling, as well as IGF-2 and TGF-α promoters, has been associated with increased hepatocyte proliferation (13,51,62). There is also evidence for transcriptional activation of the ras/raf signaling pathway leading to the activation of several oncogenes, including FOS, MYC, and JUN. Transcriptional activation of other genes, including those encoding for adhesion (intracellular adhesion molecule-1 [ICAM-1]) and HLAII molecules, is mediated by NF-κB, activator protein-1 (AP-1), and cAMP response element-binding (CREB) protein (60,61). In addition, HBx has been shown to inhibit the activity of some serine protease inhibitors and components of the proteasome complex (63), and might thus modulate the degradation and turnover of some cellular proteins involved in transcription or regulation of cell cycle progression, or both. HBx protein also binds directly to a number of transcription factors in the nucleus, such as activating transcription factor-2 (ATF-2), CREB, and p53 (58). In addition, HBx protein interacts at this site with transcription factors and elements of the basal transcription machinery transcription factor IIB, TATA box-binding protein (TBP), and RNA polymerase II subunit retinol-binding protein 5 (RBP5) (64,65).
Among the growth regulatory proteins whose functions are known to be perturbed by the HBx protein are p53, damage-specific DNA-binding protein 1 (DDB1), and the signal-mediated nuclear export receptor Crm1. The p53 tumor-suppressor gene maintains chromosomal integrity by arresting the cell cycle in G1 and regulating the DNA damage-control response, thereby permitting repair of damaged DNA (66). If repair is not possible, apoptosis is induced. HBx protein binds to specific sequences in the C-terminal end of p53 protein, preventing its entry into the nucleus and abrogating its sequence-specific DNA binding and transcriptional activity (67,68). p53 Protein transcriptionally activates a tumor-suppressor gene product PTEN (phosphatase and tensin homology deleted on chromosome 10). Inactivation of p53 and PTEN proteins by HBx protein results in increased levels of hypoxia-induced factor-1α (HIF-1α) and vascular endothelial growth factor (VEGF), both of which are important for the survival and neovascularization of early stage tumors (69).
Inactivation of p53 protein may interfere with p53-dependent DNA repair (70,71,72,73). HBx protein may also compromise p53 protein function and DNA repair in other ways, thereby leading to the accumulation of potentially transforming mutations and microsatellite instability (19,70,71,72,73,74,75,76). The protein inhibits binding of the DNA repair proteins, XBP and XPD, to p53 protein, thereby compromising the efficiency of the DNA repair. It also directly interferes with DNA repair by complexing with the DNA repair protein, hepatitis Bx–associated protein, XAP-1, which normally binds to damaged DNA in the first step of nucleotide excision repair, as well as binding to damaged DNA.
The nuclear protein encoded by the p53 gene modulates transcription of a number of genes by binding to specific DNA sequences and to other cellular factors such as mouse double mutant 2 (MDM2), TBP, and Wilm tumor-1 (WT-1) (66). Missense mutations are common in the p53 gene, and these can cause loss of tumor-suppressor function or gain in oncogenic function (66). There is no evidence that the transversion of guanine (G) to thymine (T) at codon 249 of p53 (249serp53), induced by heavy dietary exposure to AFB1, specifically interacts with the HBx protein in oncogenesis.
In addition to its inhibitory effect on p53-induced apoptosis (74,76), HBx protein inhibits caspase-3–dependent apoptosis (75). The presence of the protein may also sensitize cells to programmed cell death induced by TNF-α, an effect mediated by prolonged stimulation by N-myc transcription and the stress-mediated MAPK pathway (74). HBx protein transactivates the retinoblastoma (Rb) gene promoter, increasing expression of Rb protein, thereby resulting in cell cycle progression (77) and suppression of apoptosis (78). Another oncogenic pathway deregulated in HCC is the Wnt/β-catenin pathway. HBx protein transcriptionally upregulates the expression of β-catenin and may cause mutations of this gene (79).
Deletions in the C-terminal portion of HBx gene have been identified in HCC cells (80). Such deletions may enhance HBx protein-transforming capacity.
The PreS/S gene is also frequently included in HBV DNA integrants and might contribute to oncogenesis (55). PreS/S protein is exclusively cytoplasmic in location, and its transactivating effects are mediated by modulating PKC signal transduction; interaction with several transcription factors, such as NF-κB and AP1; and sequence-specific binding to DNA (81,82,83). Target genes for inactivation include MYC, FOS, HRAS oncogenes and the inflammation-associated cytokine IL-6 (84,85). Mutations in the transactivator region of presS/S gene may also contribute to escape of the virus from the host’s defence mechanisms. In addition, PreS mutants may result in the accumulation of abnormal S proteins in the ER and induce ER stress, possibly contributing to hepatocarcinogenesis (86). These mutants also upregulate cyclin A expression and induce hepatocyte proliferation (87).
TGF-α is overexpressed in malignant hepatocytes in which HBsAg or HBV DNA is detected (88). Some of these liver cells have a morphology consistent with that of oval cells, implying that this growth factor, driven by HBV, might favor expansion of progenitor cells. PreS1 protein may be responsible for transactivation of TGF-α and another growth factor, IGF-2.
Chronic Hepatitis C Virus Infection
In approximately 80% of individuals acutely infected with HCV, the infection persists, and about 30% ultimately develop cirrhosis. Around 170 million people worldwide are estimated to be chronically infected with this virus. Those who develop HCC have usually been infected for 20 to 40 years and almost invariably have underlying cirrhosis, with most of the remainder having advanced hepatic fibrosis or chronic hepatitis. The risk of developing HCC in those with HCV-induced cirrhosis is 1% to 7% per annum, with a lower risk in those with chronic hepatitis. HCV is the predominant cause of the tumor in Japan, Italy, and Spain, and in other industrialized countries, alcoholic cirrhosis and HCV are the main risk factors. HCV-related HCC is increasing in frequency in a number of countries, including Japan and the United States. The reservoir of HCV infection in the general population of industrialized Western countries raises concerns that there is likely to be an increasing incidence of cirrhosis and HCC in these countries in the coming decades. HCV and HBV, between them, are responsible for 70% to 85% of global HCC. Successful treatment of chronic HCV infection with antiviral agents decreases the risk of tumor development. A vaccine against HCV is not yet available and is unlikely to become available in the near future.
Relatively little is known about the molecular genesis of HCV-induced HCC. This is explained, in part, by the almost invariable association between HCV-induced cirrhosis and the tumor that led to the early assumption that the virus caused chronic necroinflammatory hepatic disease, which in turn was responsible for the malignant transformation. In addition, the lack of a reliable tissue culture system or a small animal model of HCV infection made it difficult to investigate possible mechanisms of HCV hepatocarcinogenicity. Evidence is, however, accumulating that the virus also has inherent carcinogenic potential. HCV differs from HBV in that its replicative intermediates do not integrate into cellular DNA. In common with HBV, the genome of HCV does not contain a known oncogene. The virus has, however, been shown to replicate in HCC tissue (89), and the tumor develops in transgenic mice carrying the HCV core gene alone or the complete HCV genome (90). The evidence available suggests that HCV, like HBV, promotes tumorigenesis by upregulating genes that promote hepatocyte growth and survival and downregulating genes that act as tumor suppressors and negative growth-regulatory molecules (47). The core and NS5A genes have thus far been shown to be involved in hepatocarcinogenesis. Moreover, some similarities in the oncogenic mechanisms attributed to HBx protein and the HCV core and, to a lesser extent, NS5A proteins are beginning to emerge.
A close association exists between chronic HCV infection and oxidative stress (91,92). ROS are produced by the virally induced hepatic inflammation and by expression of the core and NS5A proteins (91,93). The ROS generated are highly reactive and attack biomolecules such as DNA, lipids, and proteins. In transfection studies in tissue culture, HCV core protein has been localized to the mitochondria, where it appears to induce production of ROS (94). Mitochondrial DNA is far more susceptible to mutation caused by ROS than is nuclear DNA (95). NS5A protein also generates oxidative stress, as well as activating NF-κB and STAT-3 (93). In the presence of hepatic steatosis, a feature of HCV-induced chronic liver disease, production of ROS is further enhanced as a result, in part, of viral gene expression.
Oxidative stress may exert synergistic effects with altered intracellular signaling pathways, including indirect activation of TNF-α, raf-1-kinase, and NF-κB pathways or B-cell lymphoma-2 (Bcl-2) expression, resulting in inhibition of TNF-α– and fas-mediated apoptosis (96,97). HCV core protein is specifically incriminated in this effect, but NS5A may also be involved. Induction of nitric oxide (NO) by inducible NO synthase by viral DNA may contribute to hepatocyte injury in chronic HCV infection (91,98). Nitric oxide also reduces the frequency of apoptosis by inhibiting caspases (99).
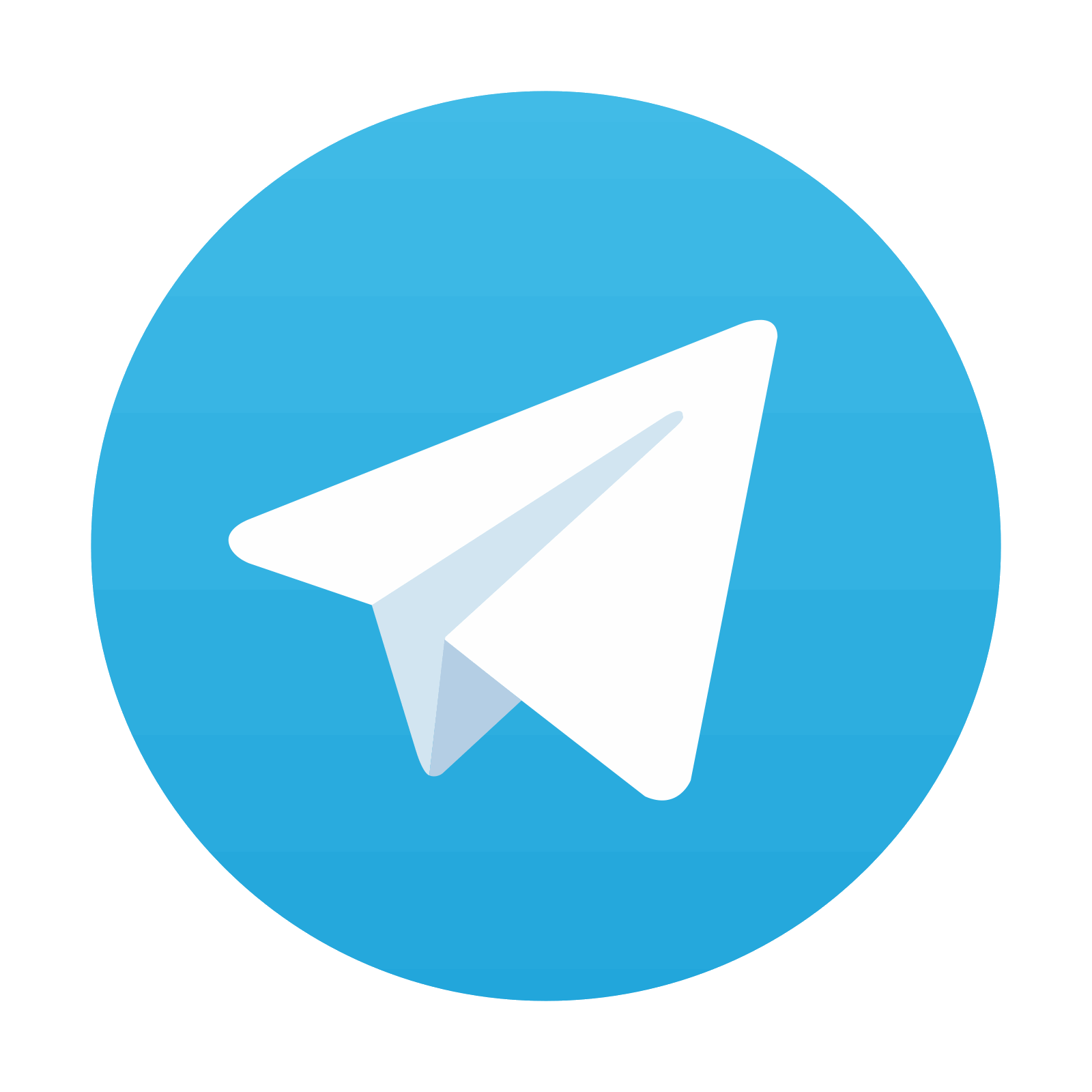
Stay updated, free articles. Join our Telegram channel
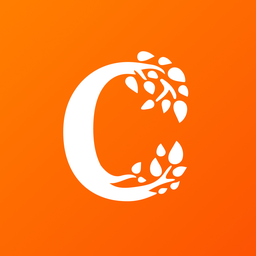
Full access? Get Clinical Tree
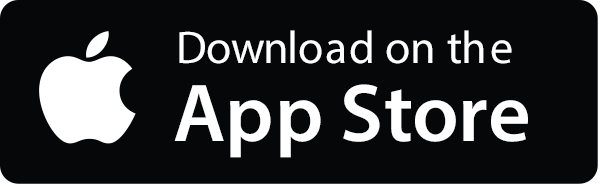
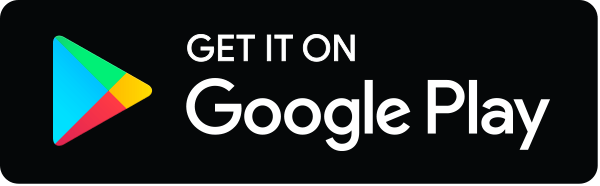