Outcomes after traumatic injury, perhaps more than in any other surgical disease, have improved through standardization of care. Nevertheless and despite seemingly ideal care, unexpected outcomes occur. Why do patients with similar injuries or severity of acute illness, despite receiving comparable and appropriate treatment, often follow different paths? While one patient recovers uneventfully from massive transfusion for hemorrhagic shock after a motor vehicle collision, another follows a prolonged course complicated by nosocomial pneumonia and organ failure. Consider also, the seemingly more straightforward task of preventing or treating deep venous thromboembolic disease. Despite our understanding of the biology of coagulation and pharmacotherapeutic strategies, venous thromboses and pulmonary emboli still occur, often with fatal consequences.
Numerous clinical, environmental, and genetic factors contribute to variability in the inflammatory response, variable rates of drug metabolism and risk for venous thromboembolic disease. The completion of the Human Genome Project and technological advances in sequencing and small molecule identification have provided the foundation on which to build knowledge of genetic variation in both humans and animal models.1 This, in turn, has been used to establish genotype–phenotype associations for common polygenic diseases, such as diabetes mellitus, cancer, and hypertension. Using this genetic variability to understand disease biology and to better direct therapy (such as drug selection and dosing) are the goals of the field of “genomic medicine.”2 Understanding the information contained in an individual’s genetic fingerprint has the potential for further decreasing the disability and mortality secondary to traumatic injury.
At the molecular level, differences in DNA sequence can alter RNA transcription and protein translation, which thereby alter clinical phenotypes. For instance, drug absorption, metabolism, and excretion are all affected by genetic variation and are the focus of an emerging field of study called pharmacogenomics. The fields of proteomics and metabolomics are additional pieces of systems biology that are promising new discoveries and improvements in patient care. Taken together these form the foundation of personalized medicine. This chapter focuses on select aspects of these fields and their potential application to the care of critically ill and injured surgical patients.
Since the discovery and publication of the molecular structure of nucleic acids by Watson and Crick in 1954, the genetic basis for many conditions has been determined; however, misconceptions remain regarding the role of genetics and genomics in clinical medicine.3 Despite the commonly held notion that genetics had little influence on clinical medicine in the past, genetics has, in fact, played an important role in understanding disease, but only for a small number of conditions and patients. As a result of these, we have entered a period of tremendous growth in our knowledge of genomics that will eventually influence care for all patients.1 In order for clinicians to understand and participate in these advances, we must become “literate” in the language of genomic medicine. Box 53-1 includes some important definitions of genetic concepts for clinicians, some of which will be more completely developed below.
Box 53-1: Definitions
Allele: One of two or more versions of a genetic sequence at a particular location in the genome.
Base pair (bp): Two nitrogenous bases paired together in double-stranded DNA by weak bonds; specific pairing of these bases (adenine with thymine and guanine with cytosine) facilitates accurate DNA replication; when quantified (eg, 8 bp), bp refers to the physical length of a sequence of nucleotides.
Complex condition: A condition caused by the interaction of multiple genes and environmental factors. Examples of complex conditions, which are also called multifactorial diseases, are sepsis, cancer and heart disease.
DNA: Deoxyribonucleic acid, the molecules inside cells that carry genetic information and pass it from one generation to the next.
Exon: The portion of a gene that encodes amino acids.
Gene: The fundamental physical and functional unit of heredity. A gene is an ordered sequence of nucleotides located in a particular position on a particular chromosome that encodes a specific functional product (ie, a protein or an RNA molecule).
Gene chip: A solid substrate, usually silicon, onto which a microscopic matrix of nucleotides is attached. Gene chips, which can take a wide variety of forms, are frequently used to measure variations in the amount or sequence of nucleic acids in a sample.
Genome: The entire set of genetic instructions found in a cell. In humans, the genome consists of 23 pairs of chromosomes, found in the nucleus, as well as a small chromosome found in the cells’ mitochondria.
Genomewide association study (GWAS): An approach used in genetics research to look for associations between many (typically hundreds of thousands) specific genetic variations (most commonly, single-nucleotide polymorphisms) and particular diseases.
Genotype: A person’s complete collection of genes. The term can also refer to the two alleles inherited for a particular gene.
Haplotype: A set of DNA variations, or polymorphisms, that tends to be inherited together. A haplotype can refer to a combination of alleles or to a set of single-nucleotide polymorphisms found on the same chromosome.
HapMap: The nickname of the International HapMap (short for “haplotype map”) Project, an international venture that seeks to map variations in human DNA sequences to facilitate the discovery of genetic variants associated with health. The HapMap describes common patterns of genetic variation among people.
Human Genome Project: An international project completed in 2003 that mapped and sequenced the entire human genome.
Microarray: A technology used to study many genes at once. Thousands of gene sequences are placed in known locations on a glass slide. A sample containing DNA or RNA is deposited on the slide, now referred to as a gene chip. The binding of complementary base pairs from the sample and the gene sequences on the chip can be measured with the use of fluorescence to detect the presence and determine the amount of specific sequences in the sample.
Mutation: A change in a DNA sequence. Germ-line mutations occur in the eggs and sperm and can be passed on to offspring, whereas somatic mutations occur in body cells and are not passed on.
Pharmacogenomics: The branch of pharmacology which deals with the influence of genetic variation on drug response in patients by correlating gene expression or single-nucleotide polymorphisms with a drug’s efficacy or toxicity. By doing so, pharmacogenomics aims to develop rational means to optimize drug therapy, with respect to the patients’ genotype, to ensure maximum efficacy with minimal adverse effects.
Phenotype: The observable traits of an individual person, such as height, eye color, and blood type. Some traits are largely determined by genotype, whereas others are largely determined by environmental factors.
Point mutation: An alteration in DNA sequence caused by a single-nucleotide base change, insertion, or deletion.
Ribonucleic acid (RNA): The molecule synthesized from the DNA template; contains the sugar ribose instead of deoxyribose, which is present in DNA; three types of RNA exist, messenger RNA (mRNA), transfer RNA (tRNA), and ribosomal RNA (rRNA).
Single-nucleotide polymorphism (SNP): A single-nucleotide variation in a genetic sequence; a common form of variation in the human genome.
Systems biology: Research that takes a holistic rather than reductionist approach to understanding organism functions.
Transcription: The synthesis of an RNA copy from a sequence of DNA (a gene); a first step in gene expression.
Translation: During protein synthesis, the process through which the sequence of bases in a molecule of messenger RNA is read in order to create a sequence of amino acids.
Much is known about the human genome, including the fact that the minority of the 3 gigabases of DNA sequence codes for proteins. It is estimated that only 2% of our DNA sequence includes the codes for approximately 20,000 protein-coding genes. The function of the remaining 98% of DNA is perhaps the most fascinating aspect of genomics. Figure 53-1 illustrates the structure of a typical gene. The 5′ control region, often termed the “promoter,” includes DNA sequences that recognize and bind to proteins called transcription factors, whose function is to modify gene expression by controlling transcription. The “start codon” is a series of nucleotides that are recognized by the transcriptional machinery, which initiates the generation of messenger RNA (mRNA) from DNA. Not all of this mRNA sequence encodes for protein. Exons are the regions of DNA that are transcribed into RNA to make amino acids. In most genes, multiple exons are separated by introns, which are removed from mRNA prior to translation into protein. The end of transcription is signaled by a series of nucleotides at the end of the coding sequence, referred to as a “stop codon.” The DNA sequence after this stop codon, termed the 3′ control region, can influence the rate of gene transcription and may affect the stability of the mRNA sequence and its subsequent translation into protein, also.
Despite our limited knowledge of the function of much of the genome, our knowledge of the genetic basis for disease is extensive. Basic research and clinical observation have elucidated the inheritance of single gene, Mendelian disorders (transmitted according to Mendel’s laws of inheritance). Most of these are uncommon and, taken together, the most prevalent (eg, cystic fibrosis and hemochromatosis), affect no more than one in several hundred people; however, when the genetic variant is present, its effect on individual patients is substantial. Furthermore, understanding the mechanisms underlying many monogenic disorders has provided pathophysiological information about related, more common disorders. For instance, we have learned a great deal about the pathophysiology of cardiovascular disease from discoveries related to familial hypercholesterolemia, a rare genetic disorder leading to premature atherosclerosis.
The most common type of DNA sequence variation is single base substitutions termed single nucleotide polymorphisms (SNPs). As naturally occurring, SNPs have emerged as important genetic markers for studying multifactorial diseases.4 Polymorphisms occur in all individuals and, by strict definition, SNPs exist with a frequency of greater than 1%.5 Therefore, the term SNP does not include numerous other single base substitutions in which the least common allele is present at a frequency of less than equal to 1% (such as “personal mutations” that may be limited to one family), nor does the term encompass other variations such as insertion/deletion polymorphisms. Importantly, SNPs should be distinguished from disease “causing” mutations, which are generally much less common, but have higher penetrance (eg, sickle cell anemia). SNPs typically do not cause disease, but, in aggregate, may influence the risk for developing a disease (eg, diabetes mellitus, asthma) or the outcome from a disease or condition (eg, death from sepsis, rate of atherosclerosis in recipients of cardiac transplants).6,7,8 SNPs that exist in mRNA-coding regions (exons) of DNA can lead to amino acid substitutions (termed a missense mutation) and, therefore, may change the structure and function of the resultant protein. This type of variant potentially has the most profound impact on protein function; however, this type of SNP is the least common.9 SNPs in the regulatory (promoter) region of a gene may influence (increase or, more often, decrease) transcription of that gene, and may influence the amount of protein available.10 Yet other SNPs may not directly alter protein abundance or function, but may be important markers for other (unidentified) functional variants—a concept known as linkage.9 Similarly, haplotypes can add complexity to this concept. A haplotype is a group of genes that are commonly inherited together, for instance, a group of SNPs on the same chromosome. Within a haplotype, a nonfunctional SNP may mark the presence of one or a group of other polymorphisms that directly alter a protein either in amount or function.
The analysis of SNPs has been facilitated by the two related following developments: (1) the establishment of large data repositories of SNPs and (2) the availability of relatively affordable “high-throughput” methods for genotyping. Recent techniques have identified at least 10 million SNPs interspersed throughout the human genome, at a frequency of one SNP per ~300 basepairs.11 Their role in critical illness, both as risk factors and in determining treatment response, has been investigated for several candidate genes.12 It is hoped that these studies will contribute to the understanding of the biology of critical illness and toward characterizing individual patient risk, treatment response and outcome.13
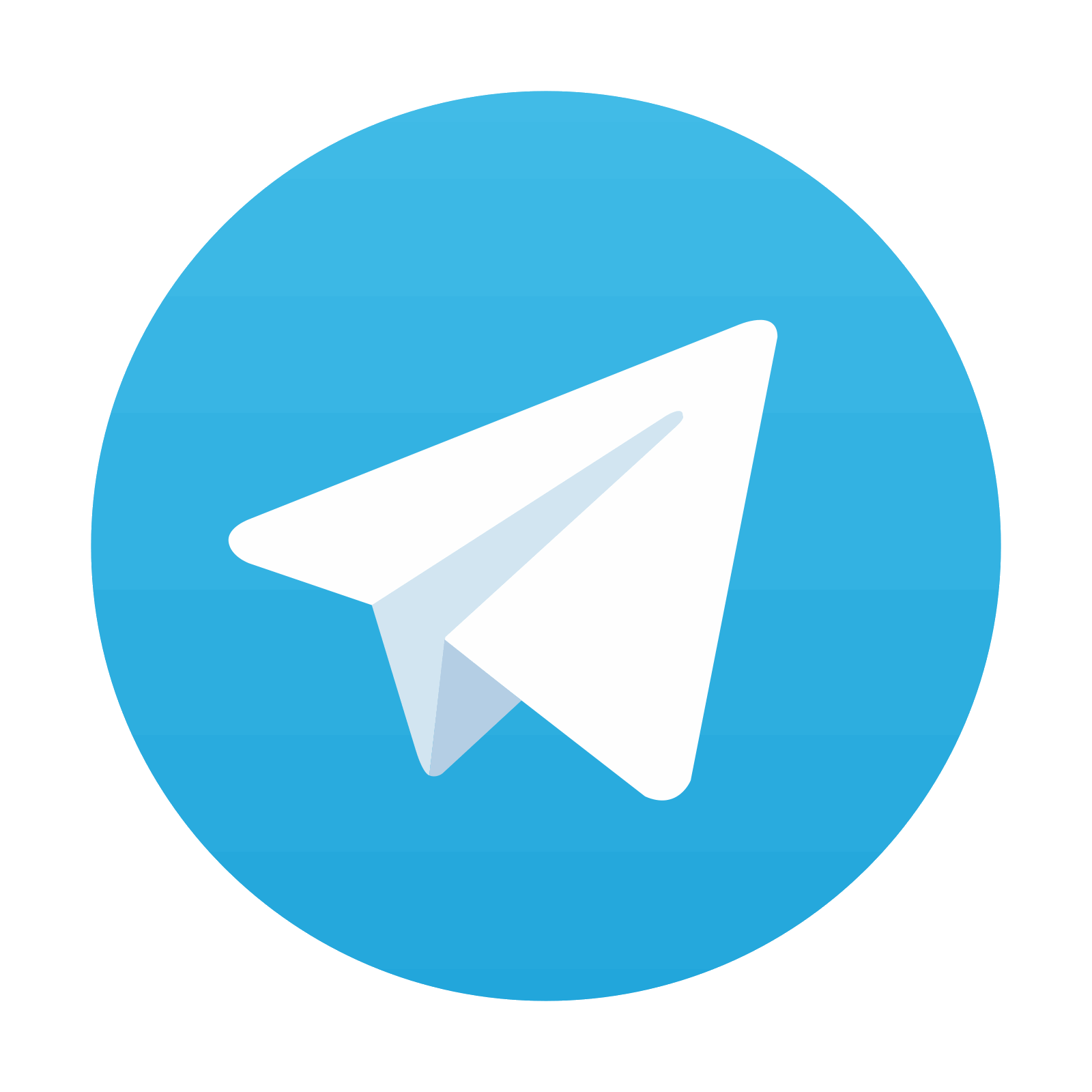
Stay updated, free articles. Join our Telegram channel
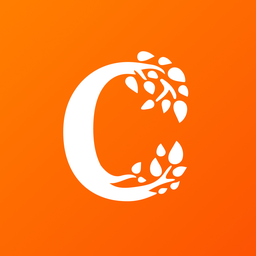
Full access? Get Clinical Tree
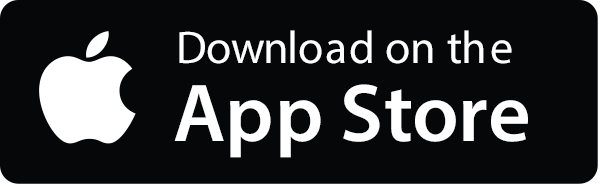
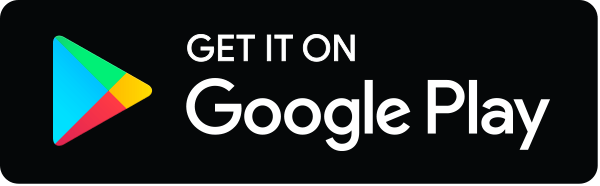