Fig. 2.1
Transverse section of intestinal smooth muscles. Gap junctions (arrows) are points of low electrical resistance between cells. They allow depolarizing signals to be instantaneously propagated to a large number of cells (Photomicrograph kindly provided by Dr. Giorgio Gabella)
Most smooth muscles of the GI tract are organized into two layers that make up the muscular sheath of the intestinal wall. Cells of the inner circular muscle layer are circumferentially organized so that each level of the gut muscle fibers simultaneously contracts to produce an annular contraction. The circular muscle layer can be further subdivided into two lamellae, one thick, outer layer and another thin, inner layer. These layers differ electrophysiologically and in the neural innervations they receive: the inner lamella receives a greater proportion of fibers from the submucosal plexus , while the outer is predominantly innervated by the myenteric plexus (see below). Muscles of the outer lamella are also larger, less electron-dense, and have more gap junctions . Thus the two lamellae of the circular muscle layer are believed to have different functional responses; however, understanding of this area remains incomplete.
Longitudinal muscles of the gut are oriented such that their axis runs along the length of the bowel. With the exception of the colon where they are organized into separate thick cords (teniae coli ), the longitudinal muscles form a continuous outer muscle sheet encircling the bowel wall. Contraction and relaxation of the longitudinal muscle layer cause alterations in the length of the bowel, important for complex motor functions such as peristalsis (see Sect. “3.2” below).
1.2 Innervation of Gut Muscle
The gut receives extrinsic sympathetic and parasympathetic innervation, but it also has its own enteric nervous system capable of independent function in many instances. As shown in Fig. 2.2a, preganglionic parasympathetic motor neurons reach the gut either via the vagus nerve or the pelvic nerve, the latter supplying efferent fibers to the distal colon and rectum. Parasympathetic fibers mainly synapse with postganglionic parasympathetic or other enteric neurons located in the intestinal wall plexuses. In contrast, preganglionic efferent fibers emanate from the spinal cord and synapse at paravertebral ganglia, from which postganglionic fibers project to the intestine, following the celiac, superior mesenteric and interior mesenteric arteries in their respective distributions.
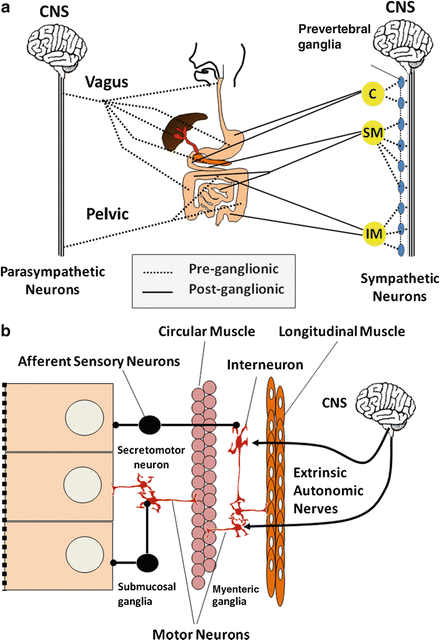
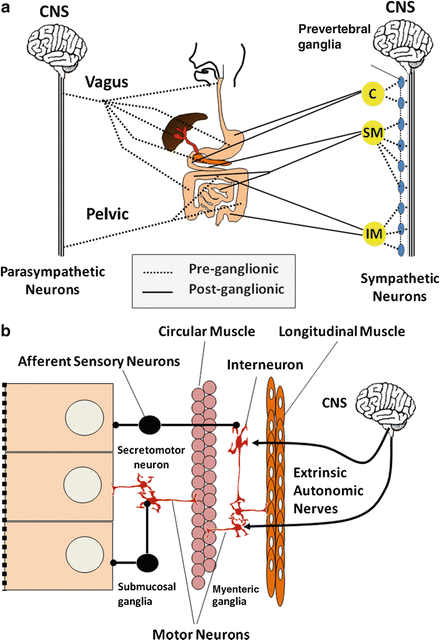
Fig. 2.2
Neural innervation of the autonomous (extrinsic) nervous system and enteric (intrinsic) nervous system of the gut. Gut functions are regulated by (a) autonomic nerves and (b) enteric neural network. C celiac, SM superior mesenteric and IM inferior mesenteric plexuses, CNS central nervous system
Intrinsic enteric neurons are predominantly found within two major gut plexuses (Fig. 2.2b). The myenteric or Auerbach’s plexus, located between the circular and longitudinal muscle layers, is a thin layer array of ganglia, ganglion cells, and inter-ganglionic nerve tracts that serve to interconnect the plexus. Enteric neurons of this plexus largely innervate longitudinal muscles and the outer lamella of the circular muscle layer. A large number of peptides and nonpeptide neurotransmitters are expressed by myenteric plexus neurons. These include vasoactive intestinal peptide (VIP), nitric oxide , peptide histidine isoleucine (PHI), substance P, and neurokinin A (NKA), to name a few. Many of these neurons have projections into adjacent muscle layers, where they are either excitatory or inhibitory, but some are interneurons involved in integrative functions. The submucosal or Meissner’s plexus is the other neuronal array found between the submucosal layers and circular muscle. This plexus has neurons that are functionally distinct from those of the myenteric plexus and, relative to intestinal motor function, appear to be projecting mainly to the inner lamella of the circular muscle layer.
Enteric neurons have been extensively studied and found to be extremely diverse and complex. At least three criteria have been used to classify enteric neurons, i.e. morphology (Dogiel type I, II and III), electrophysiological properties (S and AH neurons), and type of neurotransmitters they express. Because these classifications have been extensively discussed by numerous recent reviews, the following discussion will mostly focus on distinction of enteric neurons by the content of neurotransmitters. This provides some functional connotations meaningful for understanding their role in regulating gut motility. However, basic principles of smooth muscle electrophysiology should be reviewed first.
2 Smooth Muscle Electrophysiology
2.1 Myogenic Control System
Slow Waves and Spike Potentials
Two types of electrical activity are found in gut smooth muscle cells; they are slow waves which contribute to the basic electrical rhythm and spike potentials . Except for the esophagus and proximal stomach , most parts of the bowel have a spontaneous rhythmical fluctuation in resting membrane potential or slow waves, generally between −60 and −65 mV. This fluctuation represents an interval of cyclic depolarization followed by repolarization and is called the basic electrical rhythm (BER). The frequency of BER oscillations varies from one part of the bowel to another (Fig. 2.3), and depolarization is not necessarily accompanied by muscular contractions.
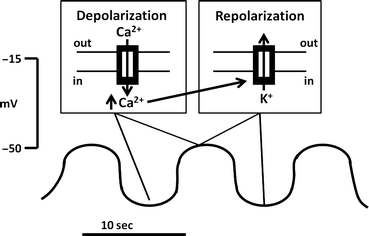
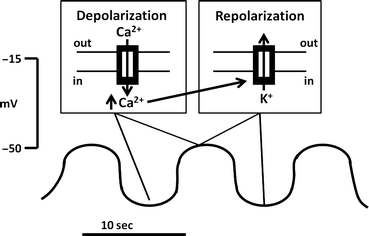
Fig. 2.3
Basic electrical rhythm or BER. The BER is a rhythmical fluctuation in resting membrane potential , characterized by intervals of cyclic depolarization followed by repolarization. BERs arise from specialized pacemaker cells. Depolarization phases are not always accompanied by muscular contraction. Inserts illustrate ion fluxes that are involved in depolarizing phases
The origin of the BER appears to be specialized “pacemaker” cells (interstitial cells of Cajal). These stellate, muscle-like cells interconnect, have projections to muscle cells, and receive neural fibers from nearby myenteric plexuses. In the stomach and small intestine , they are located at the inner circular muscle layer, near the myenteric plexus. In the colon, dominant pacemaker cells appear to be located in the submucosal border of the circular muscle layer. As in the conduction system of the heart, dominant pacemakers, i.e. ones having greater cyclic frequency, capture and entrain subsidiary pacemakers. Thus, in any region of the gut, the electrical characteristics of the dominant regional pacemaker will be not only rapidly propagated circumferentially but also transmitted orad and aborad. However, a descending gradient of pacemaker frequency is known to exist in most regions of the gut, with the possible exception of the colon. Thus, when propagation of a pacemaker BER along the long axis of the gut weakens, more distal subsidiary pacemakers will assume the pacemaker role. Studies have suggested that an aboral ascending gradient may be present in the colon. As will be discussed below, this hierarchical arrangement in BER frequency in various regions of the gut may have important functional significance.
Generation of Slow Waves
Because of the tight circumferential electrical coupling of smooth muscle cells, the BER propagation is extremely rapid and can also be measured in adjacent muscle cells in any given gut segment. The mechanism for generation of the BER is believed to be the consequence of a balance between the depolarizing inward flux of calcium through dihydropyridine-insensitive Ca2+ channels and the repolarizing efflux of K + from the cell through Ca2+-activated K+ channels (Fig. 2.3, insert). The cycle begins with the slow buildup of Ca2+ ions in the cell (a depolarizing process) that progressively inactivates further Ca2+ influx and simultaneously activates Ca2+-activated K+ channels, leading to K+ efflux (a repolarizing process).
Although depolarizations of the BER in stomach and colon can occasionally lead to small motor contractions, most contractions occur after rapid changes in membrane potential differences called spike potentials , which are superimposed on the depolarizing phase of the BER (Fig. 2.4). These “action potentials” are quite different from those seen in mammalian nerve or striated muscle. Their depolarizing phase is caused by the activation of Ca2+-dependent K+ channels or increased activity of nonselective cation or Cl− channels. This is often initiated by stimulation of cells with excitatory ligands. The resulting influx of K+ depolarizes the cells, causing an activation of voltage dependent Ca2+ channels. However, this is only one component contributing to the observed increases in cytosolic Ca2+ necessary for producing muscular contraction. In circular muscle cells, the other component involves inositol 1,4,5-triphosphate (IP 3 )-stimulated Ca 2+ release from intracellular stores, with IP3 generated from ligand-stimulated hydrolysis of membrane phosphatidylinositol . In longitudinal muscle cells, IP3 appears to have little role in activating increases in [Ca2+]i. Little IP3 is generated after ligand stimulation, and addition of IP3 to permeabilized cells has little effect in releasing Ca2+, consistent with the lack of IP3 receptors regulating internal calcium pools. In these cells, excitatory ligands appear to activate Cl− channels, depolarizing the cells and stimulating the opening of voltage-sensitive Ca2+ channels. However, the end result in both circular and longitudinal muscles may be the same. Initial increases in cytosolic calcium augment additional and more sustained increases through the activation of plasma membrane or sarcoplasmic Ca2+ channels.
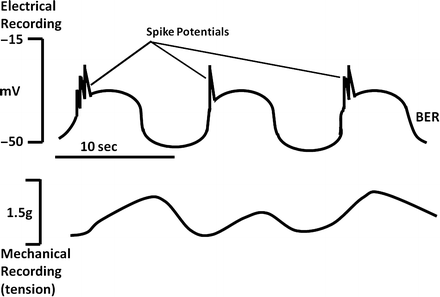
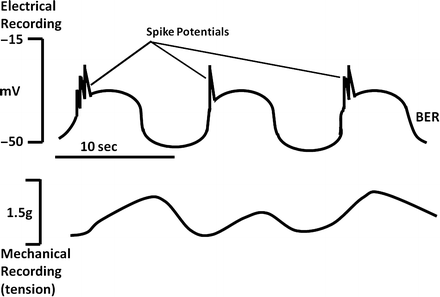
Fig. 2.4
Relationship between BER and smooth muscle contraction. The likelihood and magnitude of smooth muscle contractions depend on the number of rapid changes in membrane potential (spike potentials ) which are superimposed on the depolarizing phase of the BER
Stimulated increases in Ca2+ are required for electromechanical coupling. This explains why not all phases of smooth muscle BER are associated with contraction. Even at the peak of membrane depolarization, if the threshold potential for initiating these events is not achieved, contraction will not occur. Spike potentials superimposed on phasic BER depolarization increase the probability that the threshold potential will be reached (Fig. 2.5). The appearance and frequency of spike potentials are greatly affected by hormonal agents and neurotransmitters. The greater the number of spike potentials per BER cycle, the greater is the degree of muscular contraction. Epinephrine , for example, markedly decreases spike potentials and inhibits contraction, whereas acetylcholine stimulates them and promotes contraction. The major function of the BER, therefore, is to dictate when contractions can occur in a certain area in the bowel. Its basic rhythm does not appear to be affected by hormones and neurotransmitters. If there are no spike potentials, contractions will generally not occur. On the other hand, if an agent promotes numerous spike potentials, the frequency of muscular contractions cannot exceed that of the BER.
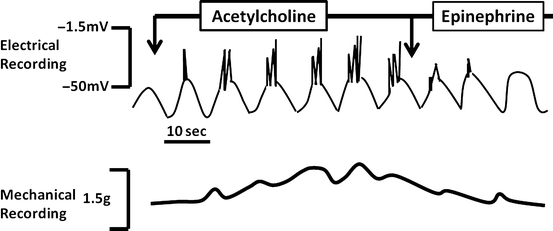
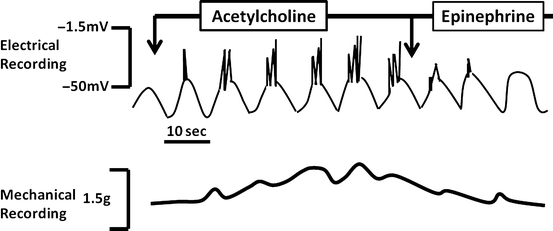
Fig. 2.5
Regulation of smooth muscle contraction. The appearance and frequency of spike potentials are greatly affected by hormonal agents and neurotransmitters. The greater the number of spike potentials there are per BER cycle, the greater is the degree of muscular contraction
2.2 Electromechanical Coupling
Contraction of Smooth Muscles
How do stimulated increases in cytosolic Ca2+ cause contraction of smooth muscles? Increased cytosolic Ca2+ binds to the calcium-regulatory protein calmodulin, forming a complex that activates myosin light chain kinase (MLC kinase) (Fig. 2.6). MLC kinase stimulates the phosphorylation of myosin, a critical step in initiating the actin-myosin interaction essential for muscular contraction. Protein kinase C, activated through the generation of diacylglycerol, also appears to have a role, especially in maintaining the duration of contraction.
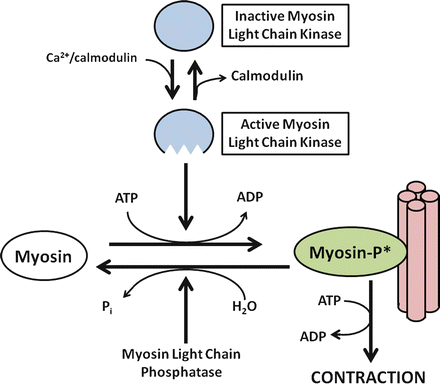
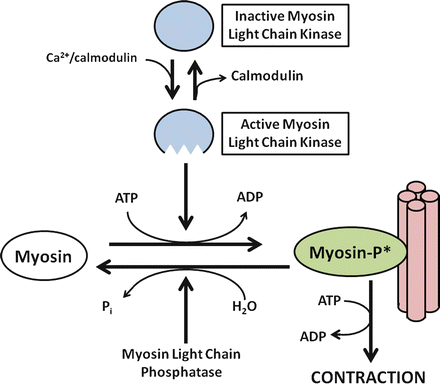
Fig. 2.6
Ca2+-dependent smooth muscle contraction. Increased cytosolic Ca2+ binds to calmodulin, a calcium-regulatory protein important for activating myosin light chain (MLC) kinase. Activating MLC kinase stimulates the phosphorylation of myosin, a critical step in initiating the actin-myosin interaction essential for muscular contraction
Relaxation of Smooth Muscles
Relaxation of smooth muscles involves restoration of basal Ca 2+ levels, decreased MLC kinase activity, and commensurate increase in MLC phosphatase action. Several mechanisms appear to be involved in decreasing cytosolic Ca2+, including the activation of Ca2+-ATPase to pump Ca2+ out of the cells or back into internal Ca2+ stores. In addition, repolarization of the plasma membrane inhibits further entry of Ca2+ via voltage-activated calcium channels. MLC phosphatase causes the dephosphorylatioin of myosin, with cessation of the actin-myosin interaction.
3 Neural and Hormonal Regulation of Gut Motility
3.1 Neurohumoral Regulators
Gut motility is regulated by the balance of effects received from excitatory and inhibitory regulatory agents. These agents can regulate motor functions in several different ways (Fig. 2.7). Some neurotransmitters and hormones have direct effects on smooth muscle, including agents such as acetylcholine , epinephrine , norepinephrine , tachykinins (such as substance P), and opioid peptides such as enkephalins and dynorphins.
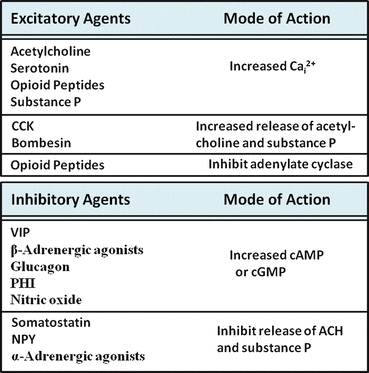
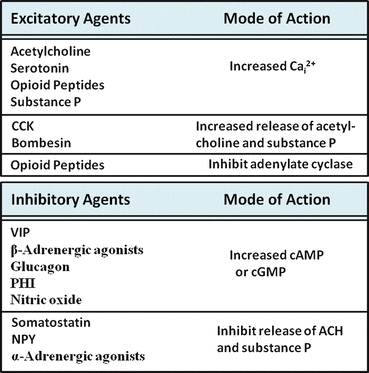
Fig. 2.7
Regulation of intestinal motor function. Agents involved in the regulation of intestinal motor function may be excitatory (upper panel) or inhibitory (lower panel). The mode of action of each representative agent is shown on the right side of each box
Inhibitory Agents
Inhibitory agents generally work by stimulating increases in cyclic adenosine monophosphate (cAMP) or cyclic guanosine monophosphate (cGMP), secondary messengers that appear to counteract the effects of increased cytolic Ca2+ in several ways. For example, the stimulation of cAMP- and cGMP-dependent protein kinases have been shown to inhibit IP3 formation and its effects on Ca2+ release from internal calcium stores. These kinases may also stimulate mechanisms involved in the re-uptake or lowering of cytosolic calcium, including stimulation of Ca2+-ATPase of plasma membrane or cellular organelles. As a third mechanism, increases in cyclic nucleotides may play a role in stimulatory mechanism, such as Ca2+-dependent K+ channels, which repolarize the cell and inhibit the actions of excitatory stimuli. Inhibitory agents that stimulate increases in smooth muscle cell cAMP include vasoactive intestinal peptide (VIP), glucagon , and peptide histidine isoleucine (PHI) or peptide leucine methionine (PHM), in humans. Nitric oxide is inhibitory because it stimulates soluble guanylate cyclase and increased cellular cGMP levels.
Excitatory Agents
Most excitatory agents of intestinal motility work by stimulating increases in cytosolic Ca 2+ and/or inhibiting the formation of cyclic nucleotides. For instance, acetylcholine ’s excitatory actions are mediated by muscarinic receptor-stimulated increases in cytosolic Ca2+. Other agents such as μ- and δ-opioid receptor agonists also inhibit adenylate cyclase activity, in addition to stimulating increases in cytosolic Ca2+. Serotonin (5-hydroxytryptamine, 5-HT), on the other hand, simultaneously stimulates increases in cytosolic Ca2+ through 5-HT2 receptors and increases in adenylate cyclase activity and cAMP via stimulation of 5-HT4 receptors. These seemingly counterproductive actions probably serve as a form of cellular feedback regulation.
Other Regulatory Agents
Other regulatory agents of intestinal motility act indirectly, either by stimulating or inhibiting the release of other neuropeptides or hormones. As an example of this modulatory role, cholecystokinin (CCK) and bombesin stimulate intestinal motility by enhancing the release of excitatory peptides such as acetylcholine and substance P. By contrast, neuropeptide Y and somatostatin inhibit the release of these agents. As another layer of complexity, agents such as somatostatin can further inhibit motility by inhibiting the release of opioid peptides that normally would inhibit VIP release of nitric oxide production. The net effect, therefore, is decreased inhibitory tone on release of VIP secretion, causing inhibition of motility.
3.2 Types of Gut Motility
Digestive and absorptive functions of the gut are very much dependent on certain characteristic patterns of motility which is present in various parts of the bowel. Each serves important functional roles, including mixing, propulsion, and separation of luminal contents. These actions are possible because of the coordinated interaction of excitatory and inhibitory neurons. As schematically shown in Fig. 2.8, stimulation of intramural baroreceptors by the presence luminal contents simultaneously activates proximal excitatory neurons that stimulate contraction and inhibitory neurons in adjacent segments that induce receptive relaxation. Thus, the regulation of each segment of bowel must be dynamic and integrated with other parts of the gut, or the consequences could be potentially disastrous.
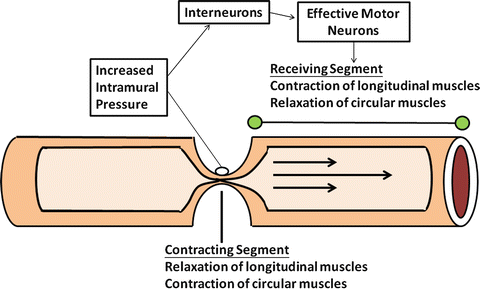
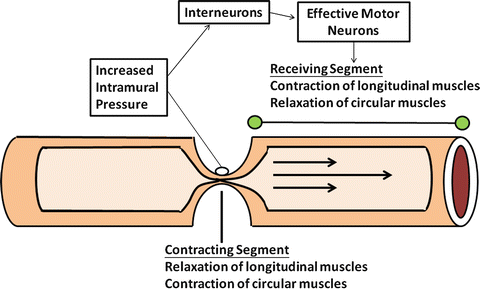
Fig. 2.8
The mechanism of peristaltic reflex. Contraction and relaxation of each adjacent segment of the gut involved are highly integrated
There are three major types of motility pattern in the gut (Fig. 2.9). (1) Segmentation is the non-propulsive annular contraction of the circular muscle layer that is predominantly found in the small and large intestines. Its major function is to mix intestinal chyme through its squeezing action; (2) Tonic contractions characterize certain regions of the gut that serve as sphincters for dividing the gut into functional segments. Important aspects of these regions are mechanisms that can inhibit tonic contraction to allow luminal contents to pass at appropriate times; (3) Peristalsis describes a highly integrated, complex motor pattern marked by sequential annular contractions of gut segments that produce a sweeping, propulsive wave forcefully moving luminal contents distally. Further discussion of these patterns in the context of specific bowel functions is provided below.
< div class='tao-gold-member'>
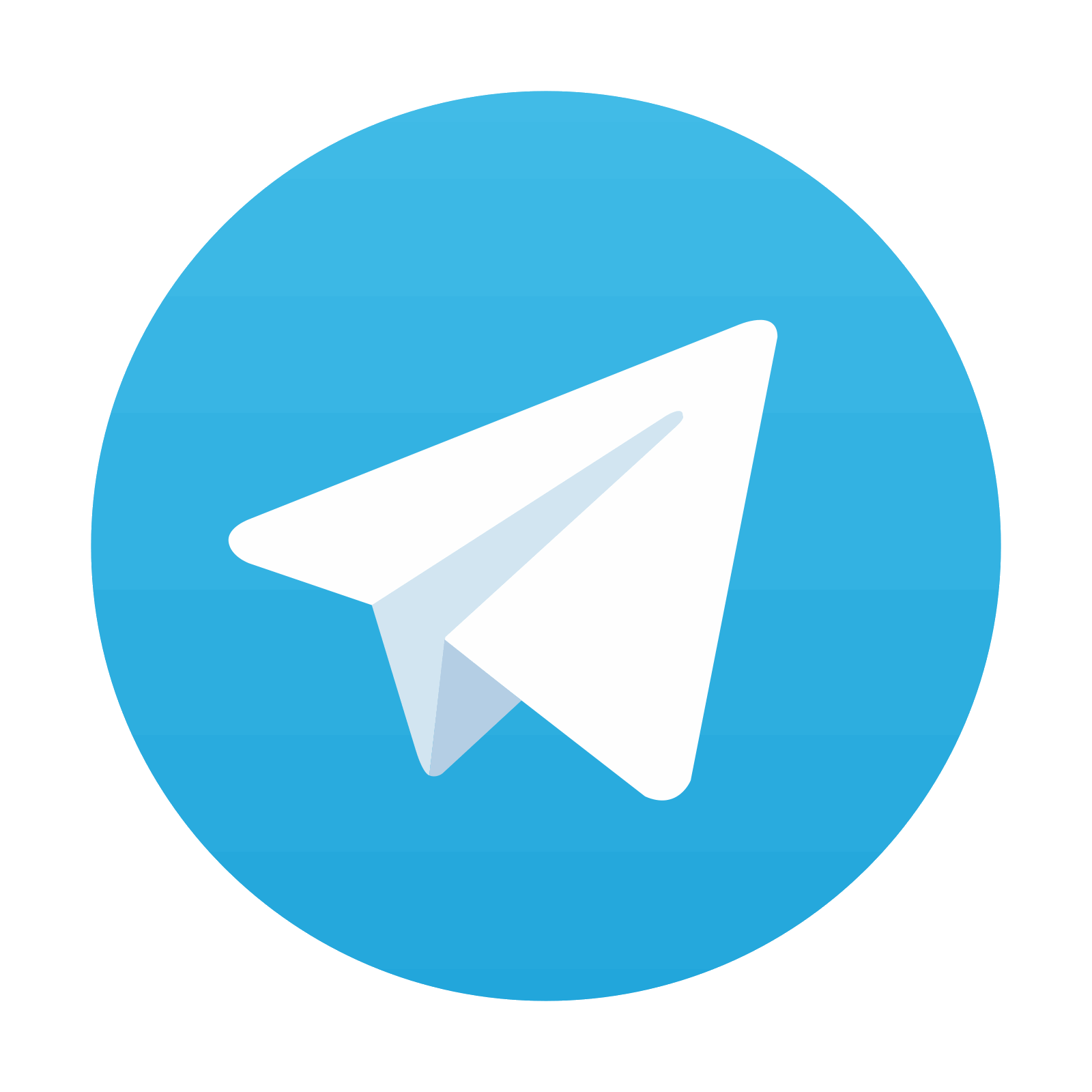
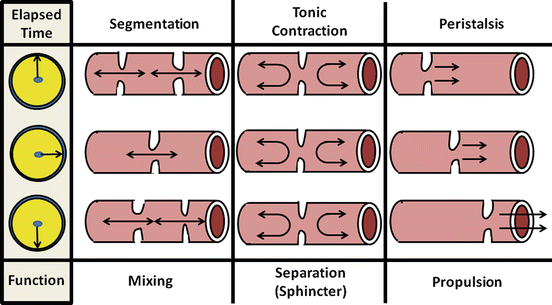
Only gold members can continue reading. Log In or Register a > to continue
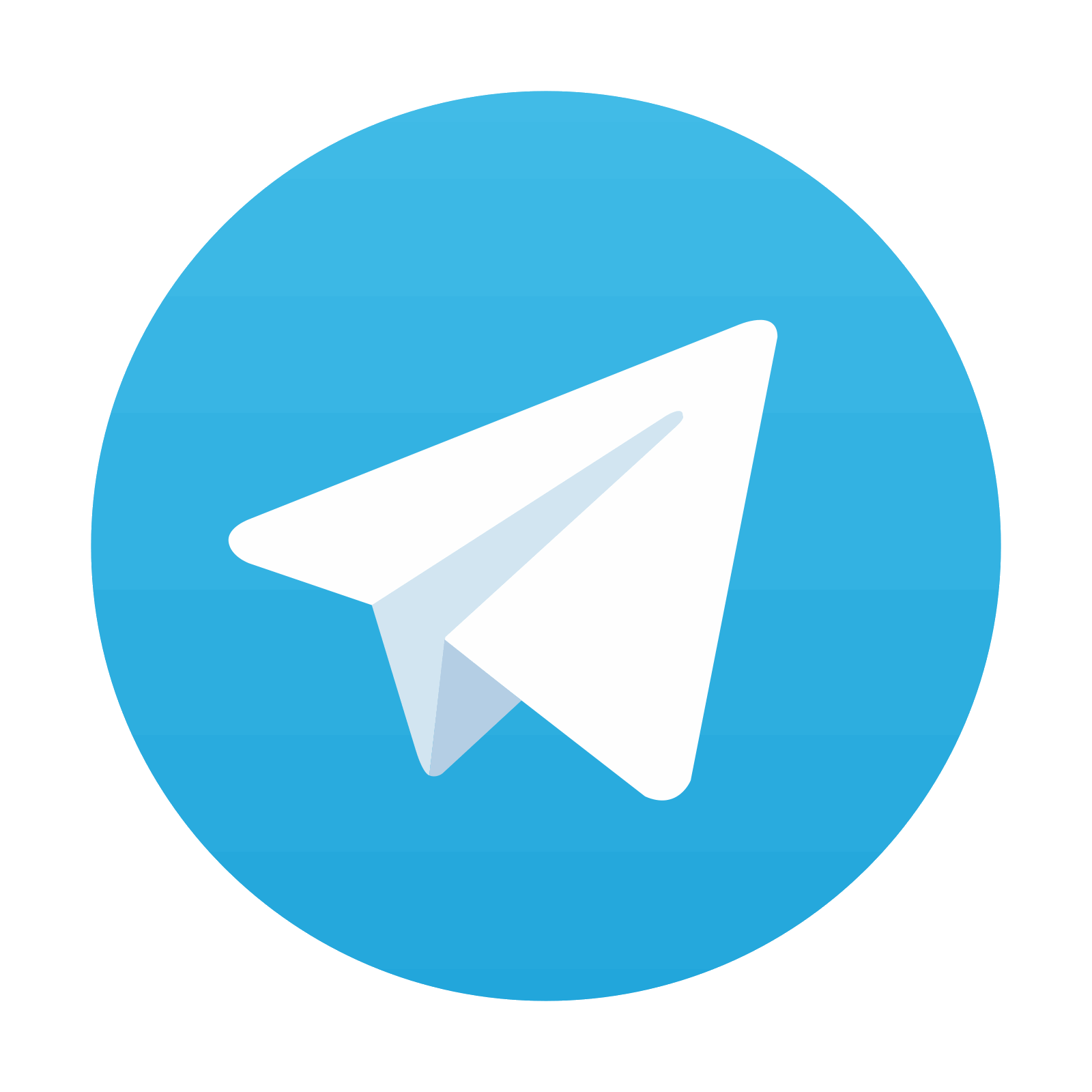
Stay updated, free articles. Join our Telegram channel
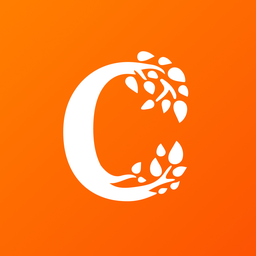
Full access? Get Clinical Tree
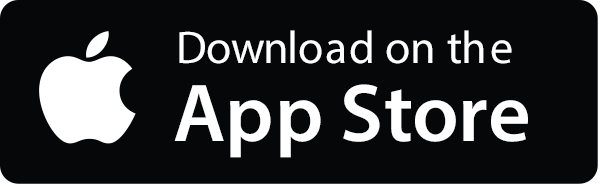
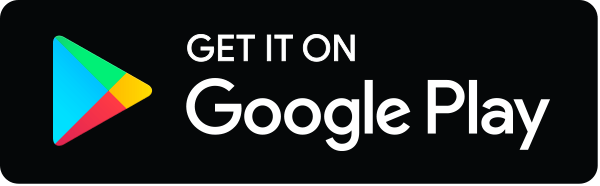