Fig. 5.1
Schematic illustration of an NIRF imaging system. Essential components of an NIRF imaging system include an NIR excitation light, collection optics, NIR filters, an NIRF video camera, a white-light illumination source, a CCD video camera, and a computer and imaging display system
During the surgery, an NIRF contrast is administered intravenously, topically, intraparenchymally, or intraluminally depending upon the application. There are several NIRF contrasts that have been used in experimental, preclinical, and clinical investigations, including methylene blue, 5-aminolevulinic acid (5-ALA), indocyanine green (ICG), and 800CW [3, 6]. For FIGS, NIRF contrasts with excitation >750 nm and red-shifted emission are the preferred fluorescent molecules due to the significant tissue penetration of excitation light and emission light as well as the lack of tissue autofluorescence at that wavelength [3, 4]. Methylene blue, while widely used as a blue dye for non-NIRF imaging purposes in medicine, can act as an NIRF imaging contrast at very low concentrations, emitting fluorescence at 700-nm wavelength [15]. It has also been used extensively as a blue dye for sentinel lymph node mapping without NIRF imaging [16]. 5-ALA is the major substrate for protoporphyrin synthesis [17]. After given to patients, it induces synthesis and accumulation of the fluorescent molecule protoporphyrin IX in epithelial and neoplastic tissues and generates fluorescence signals at 700 nm [17, 18]. 5-ALA is also known as a photodynamic therapy agent [17]. ICG, first approved by the FDA in 1958 as a contrast agent for retinal angiography [6], has since been widely used in preclinical and clinical investigations for NIRF imaging [3, 4]. ICG is an ideal NIRF imaging contrast for FIGS based on the abovementioned criteria [3, 4]. However, the major drawback of ICG is its lack of a reactive chemical group for labeling selected biomolecules [6]. ICG cannot be conjugated to targeting biomolecules, such as antibodies, with the purpose of creating cell-type-specific fluorescent contrasts [6]. Further information on ICG will be detailed in the following section of this chapter. Lastly, 800CW, which is 50 times brighter than ICG, is a newer NIRF agent currently in the preclinical development [6, 19, 20]. 800CW can be conjugated to a number of biomolecules including antibodies and peptide ligands for targeted fluorescence imaging [6, 19]. 800CW has a similar excitation and emission profile to ICG; thus, NIRF imaging systems used for ICG fluorophore can be used for 800CW [6].
Fluorescein, a non-NIRF imaging contrast with excitation and emission wavelengths of 494 and 518 nm, respectively, was approved by the FDA as a contrast agent for angiography and has been used extensively by ophthalmologists in the diagnosis of corneal abrasions, corneal ulcers, and retinal disease [3]. However, fluorescein is not ideal for FIGS secondary to its excitation and emission wavelengths within a spectrum with significant tissue autofluorescence, reducing its sensitivity [3]. Nonetheless, two landmark studies using antibodies labeled with fluorescein as intraoperative imaging contrasts have provided proof-of-principle demonstrations that FIGS has a potential to improve cancer surgery by “painting” cancer cells with a bright color [21, 22].
Indocyanine Green
Indocyanine green (ICG) is a water-soluble, tricarbocyanine dye with a molecular weight of 774.98 Da and peak spectral absorption at 800 nm. Autofluorescence of tissues and blood is very low in the 800-nm wavelength range, making the signal-to-noise ratio very high in the ICG-NIRF imaging. The chemical name for ICG is 1H-benz[e]indolium, 2-[7-[1,3-dihydro-1,1-dimethyl-3-(4-sulfobutyl)-2H-benz[e]indo-2-ylidene]-1,3,5-heptatrienyl]-1,1-dimethyl-3-(4-sulfobutyl)-, hydroxide, inner salt, sodium (Fig. 5.2). It was originally developed in the Kodak Research Laboratories in the early 1950s and was used as a cyan-forming layer of Technicolor film [4]. ICG was first used medically for cardiac output evaluation in 1956, and it later received FDA approval for clinical use [4, 23]. Soon thereafter, it was found that ICG was excreted exclusively by the liver into the bile, leading to its application for measuring hepatic function and blood flow [23]. In 1969, Kogure et al. demonstrated ICG infrared absorption angiography of the canine brain vasculature [24]. In the 1970s, Flower et al. studied retinal and choroidal blood flow using ICG angiography with a modified fundus camera and Kodak high-speed infrared black and white film [25]. ICG angiography eventually became widely accepted as a diagnostic modality for various eye diseases in ophthalmology.
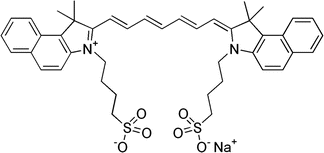
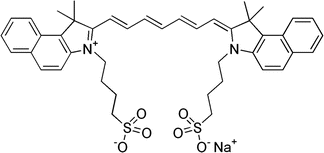
Fig. 5.2
Chemical structure of indocyanine green (ICG)
ICG is an FDA-approved NIRF dye for clinical use for cardiovascular function testing, hepatic clearance, and retinal angiography [4]. ICG’s safety profile has allowed its clinical applications and research to be expanded to other fields. Off-label uses of ICG for clinical studies are very common in many medical disciplines [3, 4]. ICG for injection is a sterile, lyophilized dark green powder containing 25 mg of ICG with no more than 5 % sodium iodide. ICG dye for clinical use can be obtained from Akorn, Inc. (Lake Forest, IL, USA) and Novadaq Technologies Inc. (Ontario, Canada). Since ICG contains sodium iodide, it should be used with caution in patients who have a history of an allergy to iodides. Anaphylactic or urticarial reactions have been reported in patients with and without history of allergy to iodides. Anaphylactic deaths have also been reported following ICG administration during cardiac catheterization [4]. Other more common side effects of ICG administration include nausea, headache, discoloration of feces, and diaphoresis [4]. No research reports have been published regarding ICG’s carcinogenicity, mutagenicity, and impairment of fertility. It is also unknown whether ICG can cause fetal harm when administered to a pregnant woman. ICG should be given to a pregnant woman only if clearly indicated and under caution.
The ICG solution must be prepared fresh. Freshly prepared ICG solutions are considered to be extremely safe; however, ICG becomes unstable once in solution and must be used within 6 h. Aged ICG solution should never be used. The concern is the potential link between aged ICG preparation and cardiac arrhythmia [26]. There is no clear explanation for this phenomenon. It has been known that based on high-performance liquid chromatography analyses, freshly prepared ICG contains two fractions, the genuine ICG (95–99 %) and a degradation product of ICG. The degradation product of ICG is likely dimerized ICGs based on its molecular weight [27]. Ott et al. reported that ICG solutions aged after 7 and 30 days of daylight exposure containing only the degraded ICG could trigger severe cardiac arrhythmias within 10 s after being injected in pigs and could result in death [26]. For this reason, one should always prepare the ICG solution right before injection. If additional injections are needed 6 h after the first injection, a new vial of ICG should be used.
After intravenous injection, ICG binds to plasma proteins, mainly to albumin and lipoproteins [4]. Its half-life in the bloodstream is only 2½–3 min. ICG is rapidly taken up by hepatocytes and is excreted via the hepatobiliary system, resulting in intense fluorescence in the liver and the bile duct. ICG is not metabolized in the liver and does not undergo enterohepatic recirculation [3, 4]. After intravenous injection, short-term tissue retention of ICG is only observed in the liver and kidneys, presumably because one of the possible ICG membrane carrier molecules, bilitranslocase, is only expressed in these two organs [4, 7]. ICG is known to be retained in liver cancer cells longer than normal hepatocytes after intravenous injection, making it ideal for intraoperative liver cancer detection [28, 29]. ICG is generally not excreted through the nephron, and therefore, there are no fluorescence signals in the urinary collecting system after intravenous injection of ICG [20]. Since ICG is not retained in tissues besides the liver after intravenous injection, it is ideal for angiography of small vessels [4]. While ICG binds to plasma proteins nonspecifically, ICG conjugated to nanoparticles could potentially be retained in specific tissue types or cancer cells [6].
Near-Infrared Fluorescence Imaging in Robotic Surgery
Many seminal preclinical and clinical research works leading to the current clinical applications of ICG-NIRF imaging in robotic urologic surgery were carried out in the Department of Urology at the University of Rochester Medical Center [7, 9]. Those research activities were supported by the Department of Urology and Novadaq Technologies Inc. Early research efforts explored ICG-NIRF imaging in intraoperative angiography, tissue perfusion, lymphatic drainage, cholangiography, and nerve labeling with animal models. Retention of ICG in the renal parenchyma was noticed, albeit in a shorter period than ICG retention in the liver. Since it was known that hepatocyte carcinoma cells retain ICG longer than normal hepatocytes, we hypothesized that it was possible that kidney cancer cells might exhibit similar ICG retention as the liver cancer does, allowing us to “paint” renal cancer in bright color with the ICG-NIRF imaging. Initial studies, led by Dr. Dragan Golijanin, were launched to investigate clinical applications of intraoperative ICG-NIRF imaging in renal cancer surgery, including open, laparoscopic, and robotic partial and radical nephrectomies. To our surprise, initial findings showed that malignant renal tumors were hypofluorescent or afluorescent in contrast to bright normal parenchyma. This led us to focus on “reverse painting” renal tumors for partial nephrectomy, i.e., painting the normal parenchyma with bright color, thus leaving the renal tumor as black under the ICG-NIRF imaging. These pilot studies with open, laparoscopic, and robotic partial nephrectomies using the Novadaq NIRF laparoscope (SPY imaging system) were later published [7, 9]. In January 2009, Novadaq announced an alliance with Intuitive Surgical Inc. to develop a SPY imaging system to be integrated with the da Vinci Surgical Robotic System. Novadaq provided specific hardware components for Intuitive Surgical Inc. to develop the current NIRF imaging platform (named Firefly system) integrated into the da Vinci Si model. A clinical trial with robotic partial nephrectomy with the Firefly system was done at the University of Rochester Medical Center in 2010 [10], which led to FDA approval of the system. Intuitive Surgical Inc. initiated commercialization of the Firefly system in February 2011 and full launch of the system began in July 2011.
The Firefly system is well integrated into the da Vinci Surgical Robot and user friendly, without impeding the surgeon’s workflow. The system has a 1080i 3D high-definition stereoscopic fluorescence-capable camera and a laser generator emitting near-infrared wavelength light at 806 nm. The normal white light view and NIRF view can be quickly toggled back and forth using a foot paddle and hand controllers. After switching to the NIRF mode, the surgical field is illuminated in shades of black and gray, and ICG fluorescence signals are displayed in a bright green color with the 3D view unaffected. The video can be viewed in real time on all video monitors connected to the system in the operating room. The white-light system has been reengineered with a LED illuminator, providing even better imaging quality than a non-Firefly system. For unclear reasons, unlike the Novadaq NIRF laparoscope, Intuitive Surgical Inc. did not incorporate the capability of combining fluorescence images with white-light views in the current system; therefore, surgeons can only view the fluorescence images with black and white backgrounds. The current Intuitive Surgical da Vinci Surgical Platform with NIRF imaging capability is depicted in Fig. 5.3.
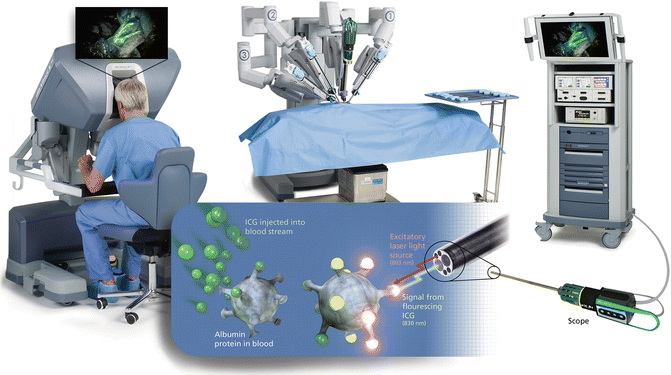
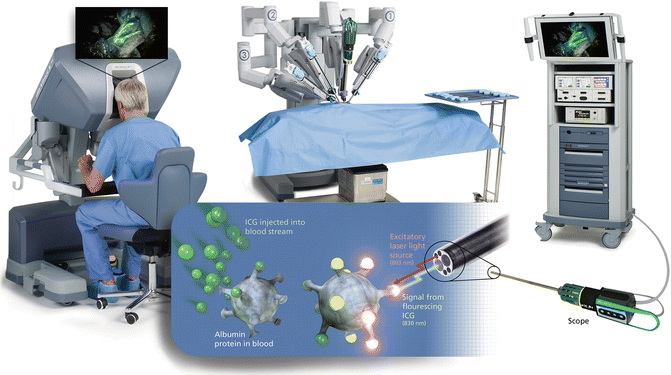
Fig. 5.3
The Intuitive Surgical da Vinci Surgical Platform with NIRF imaging capability (© 2014 Intuitive Surgical, Inc.)
After robotic surgery platforms with NIRF imaging capability became available, robotic surgeons in many centers quickly embraced this technology. Feasibility and pilot studies from different surgical specialties continue to emerge. A recent animal study in cardiac surgery has shown that the use of the ICG-NIRF imaging is feasible and beneficial in off-pump robot-assisted coronary artery bypass procedure [30]. The system easily facilitated the identification of the internal mammary artery, delineation of the coronary anatomy, and assessment of anastomotic patency [30]. For robotic single-site cholecystectomy, intraoperative ICG-NIRF cholangiography appeared to be effective to prevent common bile duct injury. This new technology was shown to be superior to the conventional intraoperative cholangiography [31, 32]. In another recent study evaluating the influence of the ICG-NIRF imaging on the location of bowel transection during robotic left-sided colorectal surgery, Hellan et al. reported that the ICG-NIRF imaging provided important additional information and resulted in a change of the proximal transection location in 40 % (16/40) of patients [33]. The ICG-NIRF imaging has also been explored by gynecologists for SLN mapping during uterine and cervical cancer surgery [16]. In urology, the ICG-NIRF imaging has been used in many different robotic procedures, including partial nephrectomy, radical prostatectomy, and upper urinary tract reconstructive procedures [10, 34–37]. These clinical reports, though considered preliminary pilot studies, have spurred significant interests in FIGS and paved the way for future advancements in this field.
Applications of Near-Infrared Fluorescence Imaging in Robotic Urologic Surgery
Partial Nephrectomy
Nephron-sparing surgery is currently a gold standard for surgical management of small renal masses and should be performed whenever technically feasible [38–40]. Even for renal tumors equal to or larger than 7 cm, partial nephrectomy may provide similar oncologic outcomes compared with radical nephrectomy in select patients [41]. Several retrospective studies have demonstrated the benefits of partial nephrectomy with improved long-term survival [42–45]. The robot-assisted laparoscopic partial nephrectomy approach has facilitated the increasing use of minimally invasive nephron-sparing surgery. When the NIRF imaging system became integrated into the da Vinci Si Surgical System, Tobis et al. carried out a pilot study on the first 11 patients who underwent a robotic partial nephrectomy with this system at the University of Rochester Medical Center in 2010 [10]. After renal hilar dissection was completed and the renal tumor was exposed, a bolus dose of ICG was given intravenously by the anesthesiologist. Different dosages were tested ranging from 0.75 to 7.5 mg. Since the half-life of ICG is only 2–5 min, repeated injections with different dosages were performed to achieve the optimal level of fluorescence. During the study, renal vascular structures could be clearly visualized [10]. Most RCCs appeared to be nonfluorescent or hypofluorescent, similar to the findings observed during open and laparoscopic surgery [7, 9, 10]. Clear macroscopic tumor margins were displayed with strong fluorescence signals [10]. This pilot study demonstrated that the ICG-NIRF imaging incorporated into the da Vinci System was a safe and effective addition to the robotic surgery platform for a robotic partial nephrectomy. This technology may provide additional advantages for intraoperative studies on the renal vasculature, renal perfusion assessments, and tumor margin identification, which may further improve the robotic partial nephrectomy [10]. Figure 5.4 demonstrates ICG-NIRF images of renal vasculature, and Fig. 5.5 shows a typical afluorescent renal tumor.
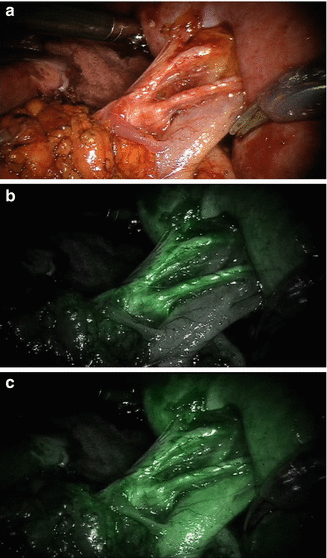
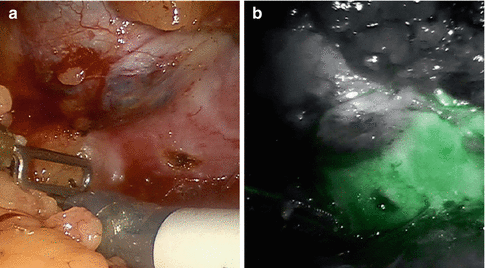
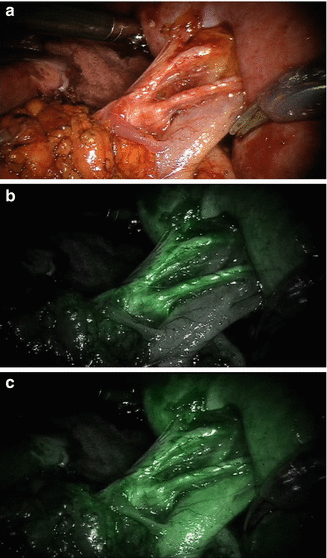
Fig. 5.4
ICG-NIRF imaging of renal vasculature at the renal hilum. (a) A white-light image of a renal hilum with the main renal artery, secondary and tertiary branches, and the renal vein exposed. (b) The arterial phase of ICG-NIRF imaging after ICG was given intravenously. The renal arterial structure turned fluorescent. (c) Renal vein turned fluorescent a few seconds later after renal artery became fluorescent
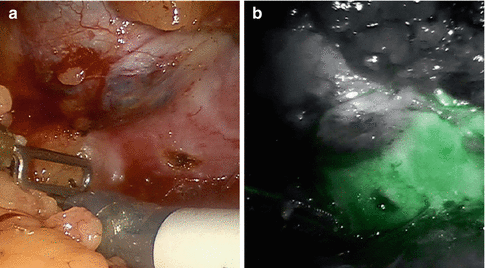
Fig. 5.5
A fluorescent renal tumor under ICG-NIRF imaging. (a) A white-light image of a renal tumor. (b) Corresponding NIRF image of the same renal tumor. Note that the normal renal parenchyma exhibited fluorescent green color while the renal tumor (renal cell carcinoma) showed no fluorescent signals
Krane et al. reported a comparative study of 94 patients who underwent a robotic partial nephrectomy with or without the ICG-NIRF imaging and found that ICG-NIRF imaging was associated with decreased warm ischemia time and subjectively enhanced visualization of renal vasculature and the mass-parenchymal interface [34]. A subsequent analysis by the same group, to evaluate the relationship of fluorescence patterns and pathologic diagnosis, showed that ICG-NIRF imaging could not differentiate renal malignancies from benign lesions [46]. All 23 benign lesions were either hypofluorescent or afluorescent, while 74 out of 77 (96 %) malignant renal tumors were also either hypofluorescent or afluorescent [46]. However, this study did highlight the usefulness of ICG-NIRF imaging in verifying macroscopic negative margins during a robotic partial nephrectomy. Since this technology is still in its infancy, there is currently a lack of standardization for the use of ICG-NIRF imaging. While the optimal dose of ICG injection during a robotic partial nephrectomy has not been established, it is clear that underdosing of ICG prevents adequate fluorescence of the peritumor renal parenchyma, while overdosing results in tumor fluorescent and loses the purpose of fluorescence differentiation between the normal renal parenchyma and the tumor tissues. Angell et al. described a dosing strategy to optimize ICG dosing for NIRF imaging during robotic partial nephrectomy [37]. In their study, a minimum of two doses of ICG were given intravenously, with an initial testing dose followed by a calibrated second dose before the renal artery was clamped for tumor resection. The test dose was given as soon as the tumor was identified. If the test dose was too high, causing renal parenchyma and tumor fluorescence, then the surgeon could wait until fluorescent signals were washed out from the kidney and the tumor in order to give another lower dose of ICG while at the same time continuing with additional dissection and preparation for the tumor resection [37]. During the 18-month study period, a total of 79 cases underwent a robotic partial nephrectomy using the NIRF imaging. The initial ICG testing doses ranged from 0.625 to 2.5 mg, and the calibrated second doses ranged from 0.625 to 5 mg. Overall, 65 of 76 tumors exhibited fluorescent patterns consistent with expectations based on pathologic findings. Of the 60 RCC patients, 55 (92 %) showed no fluorescence corresponding to the original impressions reported by Tobis et al. [10, 37]. Angell et al. recommended a starting dose of 1.25 mg based on their experience [37]. In our current practice, we start initial dosing at 2.5 mg of ICG. After the normal kidney parenchyma turns a green fluorescent color and the fluorescent intensity is optimal, the renal artery is clamped with laparoscopic bulldogs. If the intensity is not optimal, additional time is allowed for the ICG signals to be washed out and the dose is readjusted. While the arterial blood flow stopped, ICG is retained in the kidney and will last until the renal artery is unclamped. After renorrhaphy is completed, another dose of ICG may be given to reevaluate kidney perfusion as needed. The clinical value of this immediate post-partial nephrectomy perfusion assessment is unknown.
Patel et al. described their experience of a robotic partial nephrectomy for a rare renal epithelioid angiomyolipoma using NIRF imaging with ICG [47]. The tumor, a 6.5-cm right lower pole renal mass, appeared to be nonfluorescent under NIRF imaging using an intravenous injection of 5 mg of ICG. Thus, intraoperative NIRF imaging provided a real-time visual differentiation between the mass and the normal renal parenchyma. Additional experiences using the ICG-NIRF imaging system in managing uncommon types of renal tumors are needed.
While select small exophytic renal tumors could be excised without using renal hilar vessel control, the majority of endophytic and relatively large tumors require vascular control. An early unclamping technique has helped decrease the warm ischemic time during laparoscopic partial nephrectomy [48]. Gill et al. reported a strategy to further minimize the ischemic injury in robotic and laparoscopic partial nephrectomies by fine dissection of the renal vessels into the renal hilum and parenchyma to control the immediate tumor-feeding vessels and avoid the global ischemia [49]. NIRF with ICG has further facilitated the development of selective clamping and super-selective clamping in the robot-assisted laparoscopic partial nephrectomy [36, 50, 51]. NIRF with ICG renal angiography and perfusion assessment is easy to perform and allows surgeons to see the demarcation of the affected area clearly and with greater confidence.
Preoperative CT angiogram with 3D reconstruction based on 0.5–1 mm cuts is helpful for planning of super-selective clamping. Intraoperatively, the entire renal hilum is exposed in case the global arterial and/or venous control becomes necessary during the procedure. The renal vessels are mobilized to the tertiary and quaternary branches as necessary. A dose of 5 mg of ICG given intravenously is sufficient for optimal renal angiography and perfusion assessment. When the administration of ICG begins, vascular bulldogs are placed on selected arteries and the light is switched to NIRF. In complex cases, intraoperative ultrasound may be used for additional confirmation of tumor location and tumor blood flow, as tumors may be completely nonfluorescent and additional large vessels may be feeding the area. These tumor-feeding vessels may be ligated with Hem-o-lok clips (Teleflex Medical, Research Triangle Park, NC, USA). If satisfactory arterial control is achieved, the tumor can be excised under normal white-light view. The normal and NIRF view can be quickly toggled back and forth to check tumor margins. Once the tumor is excised, the tumor bed is sutured to control small bleeding vessels and repair the renal collecting system if warranted. The vascular bulldogs are removed and additional bleeding points are controlled with precise suturing. Renorrhaphy with the sliding clip technique is then employed to close the renal defect. Figure 5.6 demonstrates ICG-NIRF imaging to facilitate super-selective clamping of quaternary renal arterial branch during a robotic partial nephrectomy. Three reports have been published recently describing the experience with ICG-NIRF imaging for super-selective arterial clamping during robotic partial nephrectomy [36, 50, 51]. These authors concluded that NIRF with ICG renal angiography simplified the robotic partial nephrectomy with selective renal arterial clamping and will have an impact on functional outcome in terms of preservation of renal function by decreasing warm ischemia time.
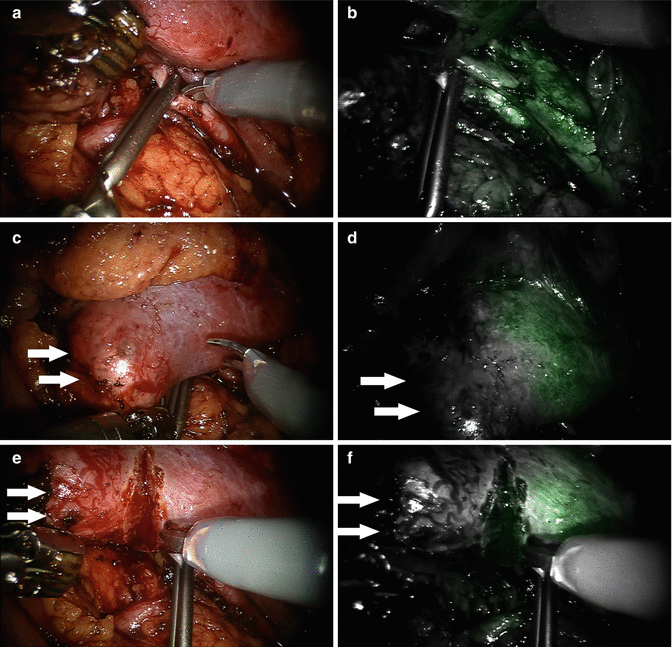
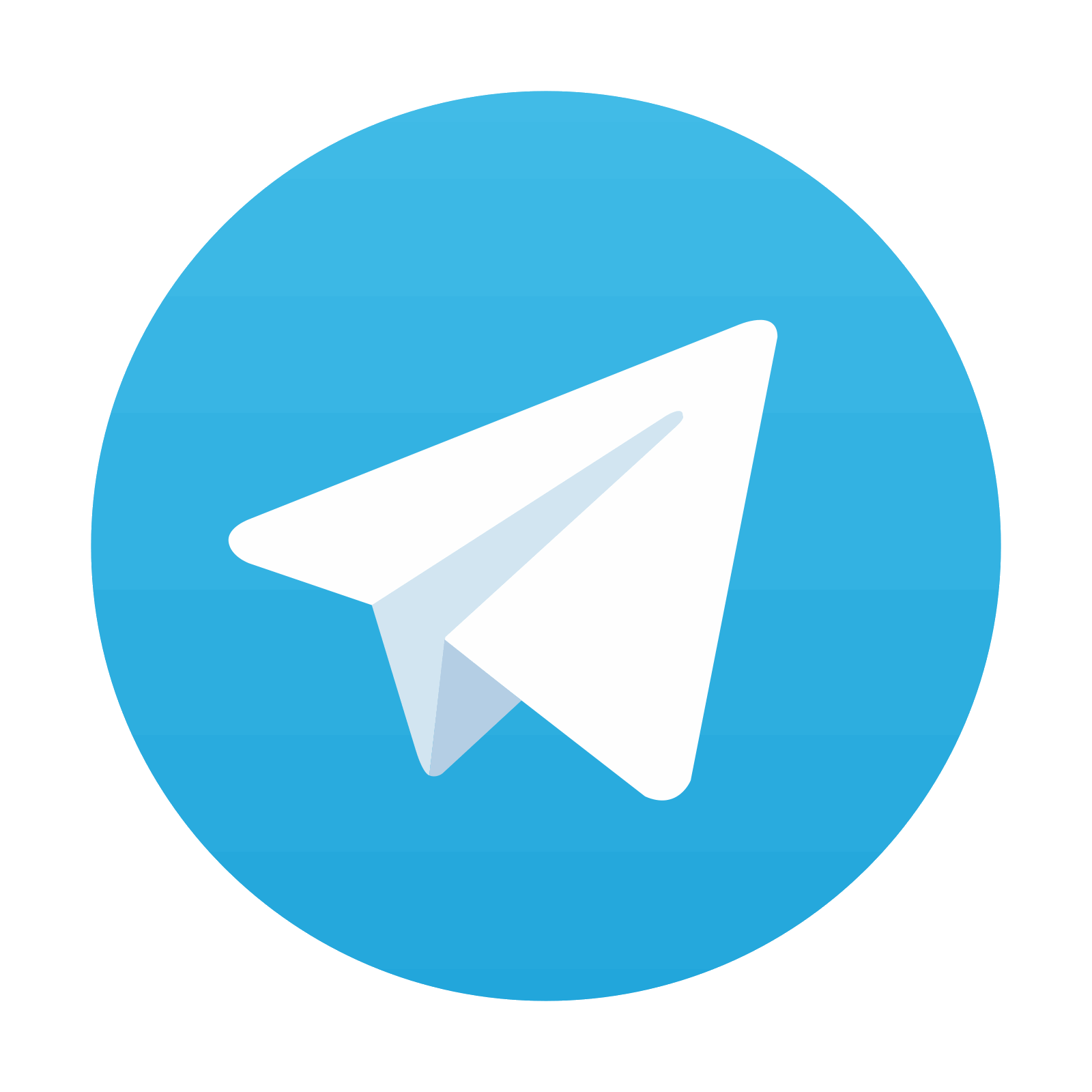
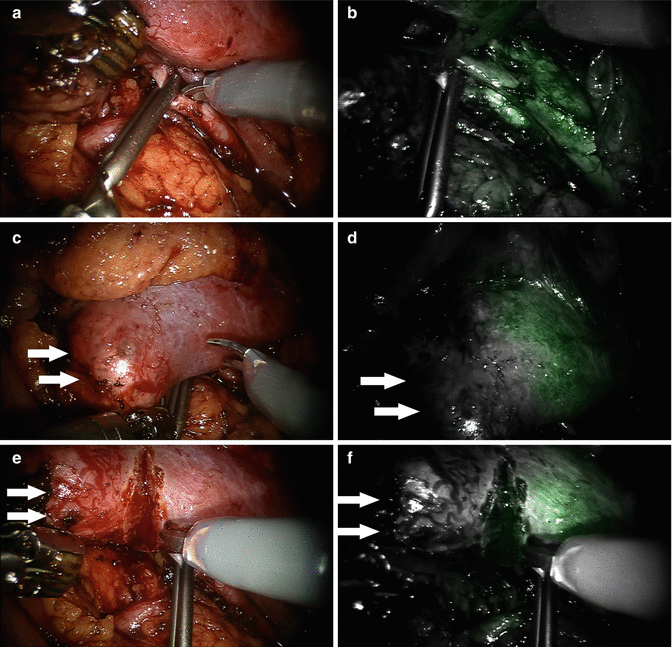
Fig. 5.6
ICG-NIRF imaging during a robotic partial nephrectomy with super-selective renal arterial clamping. (a and b) White-light and NIRF views of a renal arterial quaternary branch feeding to the lower pole of the kidney clamped with a small laparoscopic bulldog. Note that under the NIRF view (b) the distal portion of the renal arterial segment was not fluorescent, indicating no blood flow through the arterial brunch. (c, d) White-light and NIRF views of the demarcation of the renal parenchyma after the renal arterial quaternary branch was clamped. Note that the NIRF image (d) shows a clear demarcation line. (e, f) White-light and NIRF views of excision of the renal tumor after super-selective clamping. No significant bleeding was encountered. Arrows in the figure indicate the renal tumor
< div class='tao-gold-member'>
Only gold members can continue reading. Log In or Register a > to continue
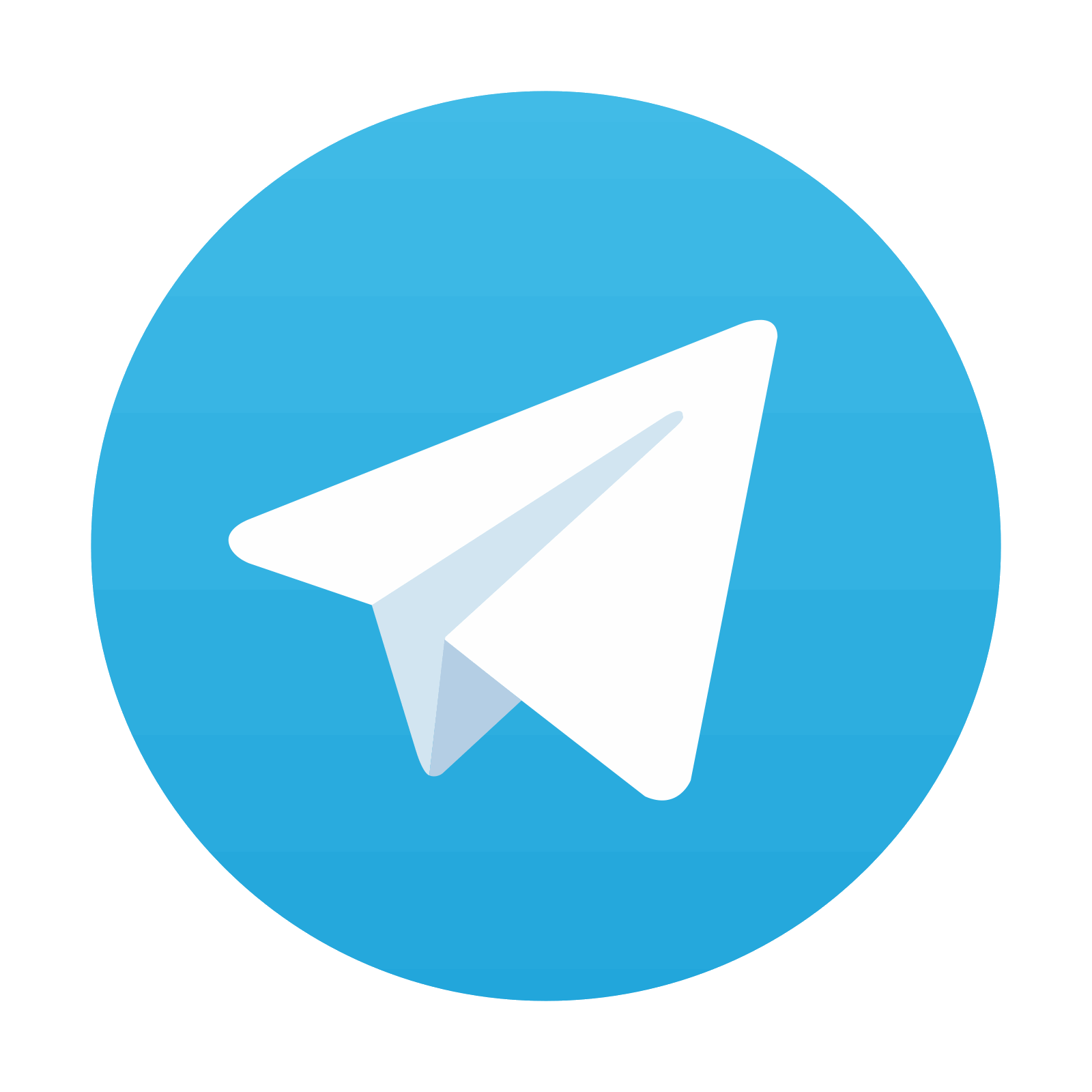
Stay updated, free articles. Join our Telegram channel
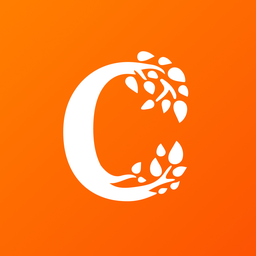
Full access? Get Clinical Tree
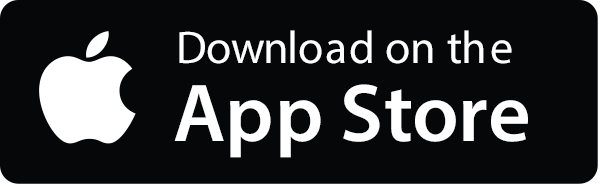
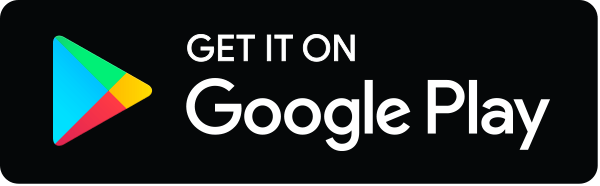
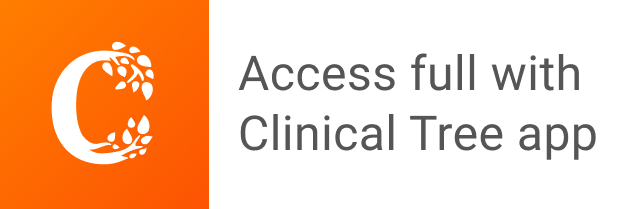