Few cell types more elegantly embody the dictum that “form follows function” than do those of polarized epithelia. It is the unique architecture of renal epithelial cells that permits them to mediate vectorial transport. This transport, in turn, essentially determines the body’s fluid and electrolyte composition. This chapter reviews the structures of renal epithelial cells and explores the mechanisms through which these structures are generated and maintained.
Keywords
epithelial; polarity; membrane; domain; matrix; cell adhesion; junctions
Introduction
Many of the chapters in this volume are devoted to the mechanisms through which the nephron is able to convert the glomerular filtrate into concentrated urine that is responsive to the metabolic status of the organism as a whole. The multifactorial nature of this problem means it needs to be treated at several levels of resolution. A meaningful description of renal tubular functions requires an understanding of the nephron’s properties as an integrated tissue, as well as those of its constituent parts, including the cells and molecules that contribute to its transport functions.
As detailed elsewhere in this volume, the nephron is a remarkably heterogeneous structure. Throughout its length, the renal tubule is notable for the marked variations in the morphologic and physiologic properties of its epithelial cells, reflecting the numerous and diverse responsibilities that neighboring segments are called on to fulfill. At the tissue level, the function of the kidney is critically dependent on the geometry and topography of the nephron. The precise juxtaposition of various epithelial cell types, which manifest distinct fluid and electrolyte transport capabilities, in large measure specifies the course of modifications to which the glomerular filtrate is exposed. This dependence on geometry also extends to renal function at the cellular level.
The Nature and Physiologic Implications of Epithelial Polarity
Despite their variations in form and function, all of the epithelial cells that line the nephron share at least one fundamental characteristic. Like their relatives in other tissues, all epithelial cells are polarized. The plasma membranes of polarized epithelial cells are divided into two morphologically and biochemically distinct domains. In the case of the nephron, the apical surfaces of the epithelial cells face the tubular lumen. The basolateral surface rests on the epithelial basement membrane, and is in contact with the interstitial fluid compartment. The lipid and protein components of these two contiguous plasmalemmal domains are almost entirely dissimilar. It is precisely these differences that account for the epithelial cell’s capacity to mediate the vectorial transport of solutes and fluid against steep concentration gradients. Thus, the subcellular geometry of renal epithelial cells is critical to renal function.
The principal cell of the collecting tubule provides a useful illustration of the importance of biochemical polarity for renal function. As described in other contributions to this volume, the principal cell is required to resorb sodium against a very steep concentration gradient. It accomplishes this task through the carefully controlled placement of ion pumps and channels. The basolateral plasma membrane of the principal cell, like that of most polarized epithelial cells, possesses a large complement of Na + /K + -ATPase. This basolateral sodium pump catalyzes the energetically unfavorable transport of three sodium ions out of the cell in exchange for two potassium ions, through the consumption of the energy embodied in one molecule of ATP. The apical surface of the principal cell lacks sodium pump, but is equipped with a sodium channel, which allows sodium ions to move passively down their concentration gradient. Through the action of the sodium pump the intracellular sodium concentration is kept low and the driving forces across the apical membrane favor the influx of sodium from the tubular fluid through the apical sodium channels. Thus, the combination of a basolateral Na + /K + -ATPase and an apical sodium channel lead to the vectorial movement of sodium from the tubule lumen to the interstitial space against its electrochemical gradient. This elegant mechanism is critically dependent upon the principal cell’s biochemical polarity. If the sodium pump and the sodium channel occupied the same plasmalemmal domain, then the gradients generated by the former could not be profitably exploited by the latter. Thus, the vectorial resorption or secretion of solutes or fluid is predicated upon the asymmetric distribution of transport proteins in polarized epithelial cells.
The fact that epithelial cells manifest biochemical polarity implies that they are endowed with the capacity to generate and maintain differentiated subdomains of their cell surface membranes. Newly synthesized membrane proteins must be targeted to the appropriate cell surface domain, and retained there following their delivery. During tissue development, cell division, and wound healing, plasmalemmal domains must be delimited and their biochemical character established. Clearly, specialized machinery and pathways must exist through which this energetically unfavorable compositional asymmetry can be supported. The nature of these specializations has been the subject of intense study for decades. While firm answers are not yet available, a number of fascinating model systems have been developed, and valuable insights have emerged. This chapter will focus on what is known of the processes through which tubular epithelial cells create their polarized geometry.
Epithelial Cell Structure: Morphology and Physiology
The renal tubular epithelium is composed of a remarkably varied collection of cell types, ranging from the highly specialized glomerular epithelial cells with foot processes that faciliate filtration of the blood through the basement membrane, to the simple squamous epithelium of the loop of Henle. A detailed delineation of its morphologic diversity is beyond the scope of this chapter. However, certain essential features are shared among all cell types in the tubular epithelium and, indeed, most other epithelial cell types found in the body. Among these are a differentiated, microvillar apical surface facing the tubular lumen, a lateral surface specialized for cell–cell interactions and regulation of transepithelial permeability, and a basal surface that adheres to the basement membrane. Furthermore, as described previously, the basolateral plasma membrane is particularly important in ion transport, because it is the location of the Na + /K + -ATPase and the cell is able to modulate its surface area in response to the transport activity of individual cell types. The cell–cell adhesive relationships are responsible for the integrity of the epithelium, and also dictate the permeability of the epithelium to small molecules that, in part, give each segment of the epithelium its physiological identity. Furthermore, adhesion of epithelial cells to each other and to the basement membrane sends spatial signals to the cells essential for the establishment and maintenance of epithelial cell polarity. In the following sections the morphology and functional composition of the apical and basolateral domains of the plasma membrane will be described, after detailing the nature of the junctional complex that mediates cell–cell adhesion.
The Junctional Complex
All epithelial cells, including those of the kidney tubule, are joined together along the lateral surfaces by a series of intercellular junctions first noted by their characteristic ultrastructural appearance and relative locations on the lateral plasma membrane. These include the tight junction or zonula occludens , the adherens junction ( zonula adherens or intermediate junction), desmosomes, and gap junctions. In most mammalian epithelia the tight junction is located at the apical-most edge of the lateral membrane closely followed by the adherens junction. Desmosomes and gap junctions have less specific locations on the lateral membrane. Desomosomes and gap junctions will be described briefly, followed by a more comprehensive description of tight junctions and adherens junctions, because of their essential functions in the organization, physiology, and morphogenesis of epithelia.
Desmosomes are large, multiprotein complexes primarily responsible for the mechanical strength of cell–cell interactions. They are formed after the assembly of adherens and tight junctions. By transmission electron microscopy they appear as discrete, focal concentrations of dense material in the cytoplasm of adjacent cells, as well as in the intercellular space. In contrast to adherens and tight junctions, desmosomes do not form an adhesive belt around the entire epithelial cell, but are a kind of “spot weld” at various points on adjacent lateral membranes. They are composed of both integral membrane proteins of the cadherin family called desmogleins and desmocollins, and peripheral membrane proteins known as desmoplakins, as well as a variety of other protein constituents. Adjacent cells adhere to each other through cadherin-mediated interactions. The peripheral components then provide mechanical stability to this interaction, via keratin intermediate filaments in the cytoplasm of each cell. Ultrastructurally, these appear as a mass of hair-like protrusions interacting in parallel with each plaque and then splaying out into the cytoplasm. In this manner, desmosomes link all cells in the epithelium. While there is evidence that desmosomal components may play an active role in regulating some aspects of cell–cell adhesion and even gene expression, in general their function is considered to be relatively passive.
Gap junctions are so named because of the characteristic 3 nm gap between adjacent cells that is evident using transmission electron microscopy. Examination of freeze-fracture specimens, which permits visualization of the internal topography of membranes, reveals the gap junction as a discrete array of intramembranous particles or connexons. Each connexon is composed of five identical connexins, a family of transmembrane proteins. Connexons on adjacent cells interact through their extracytoplasmic domains to form a series of low-resistance channels. These permit the passage of small molecules of less than 1 kDa, linking neighboring cells in the epithelium both electrically and metabolically. In the kidney, it is likely that gap junctions play important roles during morphogenesis and repair, although their precise functions have not been investigated in detail.
Among the numerous functions subserved by epithelia, perhaps the most important is that of a barrier between the intra- and extracorporeal spaces. In the case of the kidney, the extracorporeal space is defined by the lumen of the renal tubule. That the chemical composition of urine differs substantially from that of the interstitial extracellular fluid bathing the epithelial basement membranes is evidence that the barrier provided by the tubular epithelium is tight to both small and large molecules. There are two components to this barrier, arranged in parallel. The first is comprised of the apical and basolateral membranes of the epithelial cells, which together serve as a pair of series resistances to the flow of solutes across the epithelia. The second barrier is provided by the tight junction or zonula occludens that controls movement of molecules between the cells along the so-called paracellular pathway.
The tight junction defines a border between the apical and basolateral plasma membranes. In columnar and cuboidal cells of the renal epithelium, it is found at the apical extremity of the lateral membrane and in the plane of the apical surface. Analysis by transmission electron microscopy originally suggested that the tight junction is a zone of partial fusion between the plasma membranes of adjacent cells. Although this is no longer believed to be the case (see below), the ultrastructure of the junctions is consistent with this interpretation. When cells that have been treated with osmium are examined at high magnification, their membranes are distinguished by a characteristic pattern. The two leaflets of the lipid bilayer appear as a “unit membrane,” defined by a pair of darkly stained parallel lines separated from one another by 5–10 nm. In areas corresponding to the tight junction, the four parallel lines representing the two unit membrane of adjacent cells are replaced by three lines, which led to the suggestion that the two outer leaflets contributed by the neighboring cells have in some way merged to form a new trilaminar membrane structure.
The putative outer leaflet fusion suggested by morphologic studies received some support from examination of lipid mobility in polarized epithelial cells. The mobility of outer leaflet lipids is restricted by the tight junction. Labeled lipid probes inserted into the outer leaflets of epithelial apical or basolateral plasma membranes have unimpeded mobility within their respective domains, but cannot cross the tight junction. Furthermore, outer leaflet lipids are unable to diffuse between neighboring epithelial cells through the tight junction. In contrast, inner leaflet lipids can apparently move freely between the two plasma membrane domains, suggesting that the tight junction presents no barrier to their diffusion. These observations are consistent with a model of the tight junction, in which the outer leaflets of the lipid bilayer participate in the formation of some junctional structure, while the inner leaflet remains unperturbed. These results also suggest that the lipid composition of the apical inner leaflet is necessarily identical to that of the basolateral one, because any differences would quickly be randomized by diffusion. Thus, the differences in lipid compositions of the apical and basolateral surfaces alluded to in the introduction to this chapter must be entirely contributed by the constituents of the outer leaflet.
Electron microscopy has provided further insights into the structure of the tight junction. Examination of freeze-fracture replicas of epithelial cells reveals the tight junction to be composed of continuous branching and interwoven strands that surround the entire perimeter of the cell. These strands appear as elevations in the P or cytoplasmic fracture face, and are matched by grooves in the E or external face. In some cell types the strands have a fibrillar appearance, and no discrete subunit structure can be resolved. In other cell types, and in samples not fixed with glutaraldehyde, the strands can appear more as a series of particles. Although some early models postulated that the strands were composed of unusually structured lipids, it is now certain that they are primarily composed of integral membrane proteins (see below). Observations of a number of cell types with different amounts of transepithelial electrical resistance revealed a rough correlation between the number and complexity of the anastomosing strands and the degree of transepithelial electrical resistance. While this correlation certainly exists in at least some epithelia, the amount of resistance is now known to be a function of the specific complement of proteins making up tight junctions in different cells.
The first tight junction protein identified was, appropriately, ZO-1 ( zonula occludens -1). ZO-1, however, turned out to be a cytoplasmic peripheral membrane protein, suggesting that other, integral transmembrane proteins capable of mediating cell–cell contact and the intermembrane permeability barrier must exist. Shortly thereafter, occludin, a multispanning membrane protein, was identified, followed by many other proteins. It is now clear that the tight junction is an extremely complex structure composed of at least three different families of transmembrane proteins including: multiple claudins; occludin and other members of the MARVEL family; and the junctional adhesion molecules or JAMS. Additional peripheral membrane proteins are also part of the tight junction, including ZO-1, -2, and -3, and cingulins. It is also evident that the functions of these protein complexes extend beyond regulating solute permeability to participation in epithelial cell polarization.
Claudins are the most important tight junctional proteins controlling paracellular permeability of small molecules. They are the major protein constituent of the tight junctional strands seen in freeze-fracture; expression of claudins in fibroblasts produces characteristic strands and promotes cell–cell adhesion. The claudin family consists of at least 24 members in mammals. All are tetraspanning transmembrane proteins of 20–27 kD, with two extracellular loops. With one exception, the cytoplasmic C-terminal sequence of claudins interacts with ZO-1, -2, and -3. Interacting claudins on neighboring epithelial cells create charge selective channels, with the overall permeability of the tight junction to ions dependent on the particular mix of claudins expressed in the cell. This was illustrated dramatically in the renal epithelial cell line MDCK (Madin–Darby canine kidney) when expression of claudin 8, in addition to other endogenous claudins, reduced the paracellular movement of mono- and divalent cations without affecting the permeability of anions or uncharged solute molecules. In the kidney tubular epithelium, cells of the proximal tubule, which has a transepithelial electrical resistance of 6–10 Ωcm 2 , express claudins 2, 6, 9, 10, and 11, while cells of the collecting duct, with a much higher resistance of 1000 Ωcm 2 , express claudins 3, 4, 6, 7, 8, 10, and 14 ( Figure 1.1 ). Other cell types along the nephron express other combinations, yielding a range of increasing resistances in the proximal-to-distal direction ( Figure 1.1 ).

The selective barrier created primarily by claudins is sometimes referred to as the “pore pathway,” because it permits movement only of small ions and other uncharged small solute molecules. However, in at least some epithelia, there is also a kind of “leak pathway” that allows passage of larger molecules, including macromolecules. The nature of the leak pathway and its regulation is poorly understood. Occludin, which is also a tetraspanning membrane protein unrelated to claudins, may play a role in the leak pathway, together with ZO-1 and the actin cytoskeleton. Even though it is counterintuitive, an electrically tight pore pathway can co-exist with an active leak pathway, although the molecular and structural basis of this has not been fully clarified.
The tight junction is a structure whose function is highly dependent on interactions between integral and peripheral components and the actin cytoskeleton. ZO-1 and its family members are perhaps the most important class of proteins linking the various tight junctional proteins together. ZO-1 contains multiple PDZ (PSD95-Dlg-ZO-1) protein interaction domains. These bind to both claudins and JAMs, while other regions of the molecule bind to occludin and actin. ZO-1 is also capable of binding to components usually identified with adherens junctions, and to a wide variety of signaling molecules. While it is still valid to view the regulation of paracellular permeability as the primary function of the tight junction, it is more appropriate to think of the overall structure as a component of a larger apical junctional complex responsible for a multiplicity of adhesive, signaling, and membrane trafficking functions.
Originally, the tight junction was looked at as a stable, static structure in intact epithelia. Recent results using, among other approaches, expression of fluorescent tight junction proteins in cultured and intact epithelia, indicate that, in fact, the tight junction is highly dynamic. In the intestine, the epithelial leak pathway will open to permit uptake of glucose beyond the capacity of the Na + -glucose transporter in the apical membrane. This process is controlled by the actomyosin cytoskeleton, since drug-induced actin depolymerization, as well as activation of myosin light chain kinase (MLCK), compromises the epithelial barrier. Breakdown in the barrier is accompanied by simultaneous endocytosis of occludin, both implicating occludin in the regulation of the leak pathway and further demonstrating the cell’s capacity to reshape the junction. Tumor necrosis factor (TNF), which is involved in the pathogenesis of Crohn’s disease, will cause barrier breakdown through a mechanism also dependent on MLCK. Although these studies of tight junction plasticity have concentrated on the intestine, it would be surprising if similar mechanisms were not operable in the renal tubular epithelium, especially in the proximal tubule which morphologically resembles intestinal absorptive cells, and where uptake of a variety of filtered materials occurs.
The adherens junction, or zonula adherens , forms a belt just below the tight junction in most epithelial cells, connecting them via extracellular interactions and cytoplasmic linkages to the actin cytoskeleton ( Figure 1.2 ). In the electron microscope, adherens junctions appear as a dense, somewhat amorphous concentration of submembranous staining, with a mass of impinging actin filaments. The major adhesive component of the adherens junction is E-cadherin. E-cadherin is a single-pass transmembrane protein that consists of a series of calcium-binding extracellular or EC repeat domains, and a cytoplasmic tail that interacts with members of the catenin family. In adherent cells E-cadherin is concentrated in the adherens junction, but can also be more diffusely distributed over the lateral plasma membrane. Adhesion between cells occurs through trans interactions between the EC1 domains contributed by different cells in the presence of calcium, which maintains the proper conformation of the extracellular part of E-cadherin. Interactions occur initially through individual molecules, but are then consolidated and strengthened through lateral interactions of individual units.

The stability of E-cadherin-mediated adhesion is dependent on the binding of catenins to the cytoplasmic tail of E-cadherin. P120-catenin binds to a specific octapeptide located in the cytoplasmic juxtamembranous part of the cytoplasmic tail, and appears to be responsible for maintaining the stability of E-cadherin in the membrane, preventing its endocytosis and degradation. It is also involved in signaling related to cell motility, and is a substrate for the Src-receptor tyrosine kinase. The second catenin that associates with E-cadherin is β-catenin, which binds to the carboxy terminus of the cytoplasmic tail in a phosphorylation-dependent manner. Certain serine phosphorylations of E-cadherin increase the affinity of the β-catenin–E-cadherin interaction, while phosphorylation of serines on β-catenin disrupt the interaction with E-cadherin, and with α-catenin. In addition to its role in cell–cell adhesion, β-catenin is itself an important signaling molecule that is capable of entering the nucleus and regulating transcription of genes related to cell proliferation and differentiation. Its function in transcription is carefully regulated by the Wnt signaling pathway by keeping the cytoplasmic concentration of β-catenin low, either through its interaction with E-cadherin or through its degradation by a mechanism dependent on a cytoplasmic “destruction complex” and ubiquitination.
In the adherens junction, β-catenin serves as a bridge between E-cadherin and α-catenin that, in turn, interacts with the actin cytoskeleton. In this manner, cell–cell adhesion through the adherens junction is given both a degree of mechanical stability and mobility through actomyosin contraction. Originally the β-catenin–α-catenin–actin interaction was believed to be somewhat static, but recent evidence indicates that it is very dynamic. Alpha-catenin can exist as either a monomer or dimer, with the monomer able to bind β-catenin, but not actin, and the dimer able to bind actin, but not β-catenin. Three pools then exist in the cell: a monomer pool bound to β-catenin; a free cytoplasmic monomer pool; and a dimer pool bound to actin. As the adherens junction forms and consolidates, a high concentration of monomers is transported to a localized site on the membrane through β-catenin interactions. This then drives dimer formation and a dynamic linkage to the actin cytoskeleton. Localized concentration of α-catenin dimers can also inhibit Arp2/3, a mediator of actin branching essential for cell migration, and thus facilitate the transformation from a migrating cell to an adherent polarized cell during processes such as injury repair. The recognition that the adherens junction is dynamic and plays a role in cell motility has helped to transform our view of epithelia from that of a static sheet of cells to one of interlocking cells capable of constant motion and remodeling, all the while maintaining a precise permeability barrier between the inside and outside compartments of the body.
The Apical Microvillar Surface
The apical brush border membrane is perhaps best epitomized by the one that graces the epithelial cells of the proximal tubule. Named for its appearance, the proximal tubular brush border is comprised of densely packed parallel microvilli which rise like the bristles of a brush from the level of the tight junctions to a height of 1 to 1.3 μm. The proximal tubular brush border is by far the most luxuriant to be found in the nephron; although the apical membranes of other renal epithelial cell types are endowed with small collections of microvillus-like structures, much less is known about the structural specializations characteristic of the apical membranes of more distal renal epithelial cells.
The functions subserved by apical microvilli are not entirely clear. Certainly their most dramatic and obvious effect upon the properties of the apical membrane is manifest as a tremendous amplification of the apical membrane surface area. For the proximal tubule this amplification is in the order of 20-fold. As is the case for the epithelia of the small intestine, it is through this redundancy that the proximal tubular epithelial cells markedly increase the efficiency of both their absorptive and degradative functions.
Physiologically, the proximal tubule is responsible for the resorption of ~60% of the filtered load of fluid and solutes. Furthermore, it mediates the digestion of essentially all of the polysaccharides and peptides present in the glomerular filtrate, and transports the resultant sugars and amino acids from the lumen to the interstitial fluid space. It is apparent, therefore, that the epithelial cells of the proximal tubule must be specially equipped, in order to cope efficiently with the comparatively enormous quantities of fluid and substrates that rapidly transit this nephron segment. The presence of an extravagant brush border greatly increases the fraction of the tubular fluid that comes into close contact with the enzymatic and transport systems arrayed on the microvillar surfaces prior to its passage from this tubule segment into the descending loop of Henle. Concomitantly, it proportionally multiplies the number of enzymatic and transport systems available to modify the substrates dissolved in the tubular fluid. Thus, the brush border membrane provides the scaffolding for the relatively massive arsenal of enzymatic and transport machinery required to accomplish the proximal tubule’s function as a high-capacity and high-throughput absorptive system.
Ultrastructurally, a microvillus is composed of a bundle of ~19 parallel thin filaments that are linked to one another and to the overlying surface membrane by protein cross bridges. The thin filaments extend well beyond the base of the microvillus, and are anchored in a dense matrix of fibers oriented parallel to the plane of the membrane. This meshwork, referred to as the terminal web, underlies the entire apical surface and anastomses with the filaments that radiate from the lateral desmosomes and zonulae adherens ( Figure 1.2 ). The functional implications of these structural arrangements have become clearer as their components have been biochemically identified.
The thin filaments that form the microvillar core are composed of actin ( Figure 1.2 ). Ultrastructural studies employing heavy meromyosin reveal that all of the filaments in the bundle share a single polarity, and are oriented with their nucleating end towards the microvillar tip. At their termination in the microvillar tip the filaments are received by an electron-dense cap whose molecular identity has yet to be established. As they emerge from the base of the microvillus, the actin filaments are caught up in the fibers of the terminal web ( Figure 1.2 ). Fodrin, or non-erythroid spectrin, comprises one of the major components of this network. It appears to function beneath the brush border as an actin fiber cross-linker. Another of the chief constituents of this fibrillar matrix is a non-muscle form of myosin II that belongs to the same myosin subfamily as its skeletal muscle counterpart. Bipolar myosin thick filaments appear to interact with the actin filaments as they sweep out of the microvillar sheath to join the terminal web. Paired anti-parallel myosin filaments cross-link the actin filaments of neighboring microvilli to one another, forming a connection which bears close comparison to the actin–myosin arrangement characteristic of the striated muscle sarcomere. The analogy is strengthened by the presence in the microvillar rootlet of tropomyosin, a protein that functions in skeletal muscle to regulate the interaction between actin and myosin.
This marked molecular similarity between the terminal web and the skeletal muscle contractile unit prompted speculation that this arrangement might also be functionally homologous. A number of investigators have postulated that activation of myosin-based contraction at the microvillar base might lead to microvillar shortening. Repetitive activation of such a mechanism would lead to a piston-like extension and retraction of these membranous processes, which in turn might stir the surrounding tubular fluid. Such a mixing motion is certainly teleologically appealing, in that it would help to ensure that the tubular fluid is uniformly exposed to the enzymatic and transport systems of the proximal tubular apical membrane surface. No evidence for any such concerted and dynamic properties of microvilli has yet been gathered.
Biochemical studies have shed light on the identities and functional properties of some of the proteins which contribute to the interfibrillar cross bridges observed in transmission electron micrographic profiles of microvilli. Howe and Mooseker identified a protein of molecular weight 110 kDa that participates in cross-linking the filaments of intestinal microvilli to the plasma membrane. This protein exhibits a high affinity for the calcium-binding protein calmodulin, which participates in the transduction of a number of calcium-regulated phenomena. Of further interest was the fact that the 110 kDa protein manifests a myosin-like Mg-ATPase activity. Addition of ATP to intact microvilli results in solubilization of the 110 kDa protein, and disruption of the cross-links between the actin filaments and the microvillar membrane. Thus, attachment of the plasma membrane to the thin filaments may be regulated by ATP and calcium. The degree to which this putative capacity for structural modulation plays a role in microvillar function has yet to be clarified. Subsequent molecular analysis revealed that the brush border 110 kDa protein belongs to the myosin I family of unconventional myosin molecules. Unlike skeletal muscle myosin (which is assigned to the myosin II classification), brush border myosin I molecules possess a single globular head group, and do not form bipolar filaments. Members of the myosin I family, including brush border myosin (myosin Ia), have been found to associate with the membranes of intracellular vesicles, prompting the hypothesis that these motor proteins serve to propel vesicles through the cytoplasm along actin filament tracks. Co-localization studies have demonstrated that brush border myosin I and the microtubule-dependent motor protein dynein can be found together on the membranes of post-Golgi vesicles. This observation has inspired the hypothesis that apically-directed vesicles depart the Golgi along microtubule tracks powered by the action of dynein. Upon their arrival at the actin-rich terminal web, they switch engines and are carried the rest of the way to the brush border by myosin I. While brush border myosin I is abundantly expressed in intestinal epithelial cells, it may be present at lower levels in the renal proximal tubule. Since the myosin I family is large and diverse, however, it is extremely likely that an as-yet-unidentified member of this class subserves similar structural and mechanical functions in the epithelial cells of the kidney.
Another protein that apparently participates in the organization of the microvillus has a molecular weight of 95 kDa, and has been dubbed villin. Villin belongs to a large family of actin-binding proteins. Prominent in its structure is a pair of sequence domains that appear to be involved in associations with f-actin. The presence of this tandem repeat justifies the contention that villin mediates the bundling of actin fibers. It is interesting to note that villin is a calcium-binding protein, and that interaction with calcium alters its behavior in the presence of actin filaments. In experiments carried out with purified villin in solution, it has been found that this protein bundles actin filaments when the free calcium concentration is less than 1 μM. When the calcium concentration rises to 10 μM, villin severs actin filaments into short protofilaments. At intermediate calcium concentrations, villin binds to actin filaments at their growing ends, forming a cap that prevents further elongation. Due to the dynamic nature of the microfilament polymer, this capping results in the formation of shortened filaments. It is not known whether these calcium-dependent activities of villin are manifest in vivo. If villin does indeed sever or shorten actin filaments within the living cell, it would seem likely that perturbations which produce elevations of intracellular calcium concentrations may lead to structurally significant alterations in the organization of the microvillar scaffolding. During embryonic development, villin is expressed throughout the cytoplasm of epithelial cells prior to the elevation of a brush border. At later stages, villin becomes localized to the cytosolic surface of the apical membrane, and is subsequently incorporated into forming microvilli. This behavior has led to the suggestion that the localization of villin to the apical surface is a watershed event in the biogenesis of microvilli. Thus, the formation of inter-filamentous bridges, presumably mediated by villin, may be a critical first step in the organization of the microvillar infrastructure. Supporting this model are the results of experiments in which Caco-2 intestinal epithelial cells were stably transfected with a vector encoding antisense villin mRNA. The consequent reduction in villin expression resulted in a loss of the brush border and mis-sorting of a subset of apical microvillar proteins. It must be noted, however, that results from gene knockout experiments argue against an obligate role for villin in microvillus formation. Mice whose villin genes have been disrupted, and which produce no villin protein, are able nonetheless to generate morphologically and apparently physiologically normal brush borders. Presumably, other components of the microvillar infrastructure can shoulder the cross-linking and organizational duties normally performed by villin. Such functional redundancy is typical of biological systems endowed with architecture as esthetically elegant and complex as that which graces the microvillus.
While villin is limited in its distribution to those cell types endowed with brush borders, another actin-bundling component of the microvillus is present in numerous structures. Plastin-1, which is also known as fimbrin, is a 68 kDa polypeptide associated with the interfilamentous cross bridges that can also be detected in hair cell stereocilia and in ruffled borders. Plastin-1 is clearly a multivalent actin-binding protein, and participates in the cross-linking of the microvillar actin filament array. Structural studies suggest that the cross-linking activity of plastin-1 constitutes the principal means through which the parallel actin filaments are interconnected in microvilli, and the length and organization of brush borders are abnormal in plastin-1 knockout mice. A third bundling protein, known as espin, also participates in the organization of the microvillar actin filaments. While microvilli appear to form normally in the absence of espin, espin overexpression leads to microvillar lengthening by exerting subtle effects on the relative rates of actin filament polymerization and depolymerization. Simultaneous knockout of plastin-1, villin, and espin produces animals whose brush borders are short, and characterized by reduced numbers of disorganized actin filaments and mislocalized myosin. Interestingly, localization of enzymes that are normally concentrated in microvilli is markedly compromised in epithelial cells from these animals, suggesting that the organization of the overlying plasma membrane is dependent upon the structural integrity of the microvillar actin bundle.
Several other polypeptides, associated with the microvillus core and the terminal web, have also been identified. Among the most interesting and important of these is ezrin, a member of the ezrin–radixin–moesin family of proteins. The C-terminal tails of these polypeptides bind to actin filaments, while their N-termini interact with proteins in the membrane. A number of proteins involved in the generation or regulation of intracellular second messengers associate in macromolecular complexes with ezrin–radixin–moesin family members, suggesting that in addition to functioning as linkers these proteins may also act as scaffolding for the assembly of components involved in signal transduction. Knockout of ezrin expression results in shortened and poorly formed brush border microvilli, and perturbations in the organization of the terminal web. In addition, ezrin participates in forming complex molecular scaffolds that regulate and stabilize the expression of solute transport proteins in the apical membranes of renal epithelial cells.
The terminal web mentioned above consists of three morphologically distinguishable domains. In addition to the cytoskeletal fibers that receive the rootlets of the microvilli, fibers that arise from desmosomes and the zonula adherens contribute to this meshwork. The desmosomal fibers consist primarily of 10 nm intermediate filaments composed of keratins. At the level of the zonula adherens , the cell is ringed by a complex of randomly polarized actin filaments which also contains myosin and tropomyosin ( Figure 1.2 ). In vitro experiments have demonstrated that this ring has the capacity to contract circumferentially. This capacity has led to the idea that contraction of the zonula adherens ring might contribute to the alterations in tight junctional permeability which have been observed in several epithelial systems in response to certain second messengers and osmotic stress, as described earlier. Thus, activation of sodium-coupled glucose uptake in cultured intestinal epithelial cells has been shown to induce a decrease in transepithelial resistance. This effect is dependent upon the activity of myosin light chain kinase. It is thought that by shortening in a “purse-string” fashion, these filaments might actually draw neighboring cells away from one another, and thus modify the structure and permeability of the occluding junctions. The relevance of this model to the functioning of renal epithelia has yet to be established.
The anisotropy and structural complexity that characterize the filamentous core of the microvillus apparently also extend to its overlying plasma membrane. The proteins embedded in, and associated with, the plasmalemma of the proximal tubule brush border are not uniformly distributed over its surface, but rather are restricted to specific subdomains. This lateral segregation is epitomized by the behavior of two transmembrane polypeptides, maltase and gp330. The 300 kDa enzyme maltase is distributed over the entire surface of the microvilli themselves, but is absent from the intermicrovillar membrane regions. In contrast, the heavily glycosylated gp330 (also known as megalin) is restricted in its distribution to these intermicrovillar regions. The restriction of megalin to the intermicrovillar regions appears to be mediated by its interactions with protein components of the endocytic machinery. Ultrastructural examination of the intermicrovillar regions reveals the presence of coated pits. The cytosolic surface of the plasma membrane in these domains is coated with an electron dense material that biochemical and immunoelectron microscopic studies have demonstrated to be clathrin. The presence in these intermicrovillar pits of morphologic and compositional features associated with the process of endocytosis has led investigators to believe that this domain mediates the retrieval of large peptides and proteins from the proximal tubular fluid. The proximal tubular epithelial cells are responsible for capturing and degrading any proteins that pass through the glomerular filtration barrier. This function is apparently served by the profusion of coated pits and vesicles that decorate the surfaces of membranes at the microvillar base. Megalin is a member of the LDL receptor family and, together with cubulin, serves as a receptor that binds to and mediates the uptake of filtered proteins and peptides. Megalin knockout mice exhibit low molecular weight proteinuria, establishing the critical role for megalin as the proximal tubule’s pre-eminent scavenger.
Finally, it is worth noting that most or all of the epithelial cells of the nephron are endowed with a single primary cilium ( Figure 1.2 ). This non-motile cilium possesses a ring of nine microtubules, but lacks the central pair of microtubules found in motile cilia. This primary cilium appears to serve sensory functions. Bending the primary cilium, in response to flow or mechanical stimuli, induces calcium signaling in renal epithelial cells. Furthermore, the functional integrity of the primary cilium appears to be a prerequisite for the maintenance of normal renal tubular architecture. A number of cystic diseases of the kidney are attributable to mutations in genes encoding proteins found in cilia. Similarly, mice in which expression of ciliary proteins has been disrupted develop cysts. The mechanisms through which loss of the cilium’s mechanosensory functions leads to cystic transformation remain to be established.
The Basolateral Plasma Membrane
The rigid subservience of structure to function so elegantly exemplified by the apical microvillar membrane also extends to the basolateral surface of the epithelial plasma membrane. As was mentioned above, the basolateral membrane possesses the ion pumps that power the transepithelial resorption of solutes and water. The resorptive capacity of a given cell type is thus largely dependent on the quantity of ion pumps embedded within its basolateral membrane. This parameter appears, in turn, to be roughly proportional to the surface area encompassed by this membrane domain. Consequently, renal epithelial cells that participate in resorption of large quantities of ions and fluid (such as those of the proximal tubule), as well as cells that carry out resorption of ions against steep concentration gradients (such as those of the thick ascending limb of the loop of Henle), are endowed with basolateral plasma membranes whose surface areas are amplified through massively redundant infoldings.
As was detailed in the discussion of the apical membrane, the lateral distribution of proteins within the plane of the basolateral membrane is not uniform. This fact is most dramatically illustrated by epithelial cell types that lack the deeply invaginated basolateral infoldings discussed above. Studies have demonstrated that the Na + /K + -ATPase is concentrated in subdomains of the basolateral membranes of small intestinal epithelial cells. The sodium pump is essentially restricted to the lateral membranes of these cells, and is absent from the basal surfaces that rest on the basement membrane. Dislodging these cells from the underlying basement membrane produces a redistribution of the sodium pump throughout the entire basolateral surface. These results suggest that the sodium pump is either actively or passively prevented from entering the basal domain of the plasma membrane, in some manner that is dependent on an intact interaction with the basement membrane. The meshwork of cytoskeletal elements associated with those sites at which the epithelial cell is anchored to the basement membrane may be too dense to allow membrane proteins such as the sodium pump to penetrate. Conversely, cytoskeletal restraints whose integrity requires cell attachment to the basement membrane might retain the sodium pump within the lateral subdomains. In each of these scenarios, the cytoskeleton plays an important role in determining the subcellular distribution of a transmembrane protein. Research over the years has made it quite clear that the cytoskeleton plays a critical role in defining polarized domains, and in determining aspects of their protein compositions.
The Basement Membrane
The basement membrane, while not strictly part of the epithelium, is such an essential contributor to epithelial function that it cannot be excluded from any comprehensive description of the renal epithelium. The basement membrane is a thin layer of secreted and assembled extracellular matrix that underlies all epithelia and endothelia in the body, and also surrounds skeletal muscle fibers and peripheral nerves. In the past, the terms basement membrane and basal lamina were used inconsistently to describe morphological features of this layer, but there is no longer sufficient reason to distinguish these terms from each other, and they may be used interchangeably. In the kidney, the tubular basement membrane is comparable to that found under other epithelia in the body, while that of the glomerulus is more complex and unusual. In the glomerulus, the basement membrane is synthesized from proteins secreted by both podocytes and the closely apposed endothelium, resulting in a double-thick layer of matrix proteins that is an essential part of the glomerular blood filter. Diseases affecting the glomerular basement membrane often lead to compromise of the filter and proteinuria. Detailed discussion of this barrier and its specialized and distinctive composition is beyond the scope of our overall discussion of the biology of the renal epithelium, and will not be pursued in this chapter.
Basement membranes are visible by transmission electron microscopy of glutaraldehyde-fixed and heavy metal stained thin sections of epithelia, and classically appear as an electron dense layer ( lamina densa ) separated from an electron lucid ( lamina lucida ) layer adjacent to the basal epithelial surface. While these morphological features were originally believed to have a structural basis, there is now evidence that they may be fixation artifacts. All basement membranes are composed of a common set of protein components which include laminins, type IV collagen, heparan sulfate proteoglycans, and nidogen, although the specific types of these can vary depending on both developmental stage and tissue, as well as accompanying pathology. The most important component is probably laminin, because of its role in both assembly of the basement membrane and signaling. Laminins consist of large (~400–800 kDa) heterotrimeric secreted glycoproteins. In mammals, five α-, three β-, and three γ-subunits have been identified in at least 15 different heterotrimeric complexes. Prototypical laminins are cross-shaped molecules in which the short arms of the cross are contributed by the amino-termini of each subunit, and the stem by a coiled-coil made up of the carboxy-terminal halves of each subunit. Typically, the amino-termini of each subunit consist of a globular LN or polymer domain that is involved in basement membrane assembly. The carboxy-terminus of the α-subunit is folded into a series of five globular domains (G1–5) that are essential for binding to the cell surface. Laminins are named according to their subunit composition, such that LM-511, the most common laminin in the kidney, is composed of the α5-, β1-, and γ1-subunits. Like all collagens, collagen IV is a trimeric molecule composed of combinations of various type IV α-subunits that fold into an elongated triple helix. In contrast with fibrillar collagens such as collagen I, type IV collagen does not form bundles, because of the persistence of carboxy-terminal noncollagenous domains (NC1) and interruptions in the collagen repeats within the triple-helix forming regions that render the molecule more flexible. The most common types of proteoglycans found in the basement membrane are perlecan and agrin. Each is a complex molecule that contains a variety of structural motifs resembling those found in laminins, in addition to substantial negatively-charged heparan sulfate polysaccharides. Nidogen (also called entactin) is a relatively small basement membrane protein that acts primarily to link laminin and collagen IV in the assembled structure. In addition to the core components of laminin, collagen IV, and proteoglycans, a variety of other minor components may also be present under particular, poorly-defined conditions, including extracellular matrix proteins normally considered to be primarily components of the interstitial matrix, such as fibronectin.
Basement membranes initially form during embryogenesis, and are then remodeled during development. In addition, de novo basement membrane assembly may occur in adults following injuries that interrupt basement membrane continuity. Assembly is believed to occur through a mass-action process driven primarily by laminin polymerization. Laminin molecules secreted by epithelial cells bind to receptors on the basal cell surface until the density of bound molecules permits formation of heterotrimeric complexes of α, β, and γ amino-terminal LN domains contributed by three different laminin molecules. The resulting structure is a polymerized network of molecules closely associated with the basal surface. Subsequently, collagen IV intercalates into this primary network to form a secondary network created by head-to-tail interactions between collagen IV molecules. The two networks are then linked through nidogen interactions between laminin and collagen, and other molecules, including notably proteoglycans, fill the spaces within the interlocked laminin and collagen networks.
During pathological processes such as renal cyst formation and recovery from ischemic injury to the tubular epithelium, there is evidence that the atypical laminin isoform LM-332 is expressed. This laminin consists of the α3-, β3-, and γ2-subunits, with both the α3- and γ2-subunits lacking amino-terminal LN domains, precluding the molecule from participating in typical network formation. The specific function of LM-332 in these pathological situations is unknown, but one hypothesis is that it interacts with prototypical laminins, such as LM-511, to terminate or even disrupt normal basement membrane assembly, facilitating remodeling of the basement membrane, and possibly signaling the epithelium to differentiate into a more plastic state suitable for injury repair.
The basement membrane interacts with epithelial cells primarily by binding to the integrin family of extracellular matrix receptors. Integrins are a superfamily of cell adhesion receptors found in nearly all cells. Each integrin consists of a heterodimer of α- and β-subunits, both of which are transmembrane glycoproteins. A total of 18 α- and 8 β-subunits are known in mammals, resulting in at least 24 heterodimers. Although integrins are known primarily as receptors for extracellular matrix proteins, they may also participate in cell–cell adhesion. Epithelial cells of the kidney and other organs typically express an array of integrins, including multiple forms with the β1-subunit, such as α2β1 and α3β1, as well as integrins with the β3-, β5-, and β6-subunits in combination with αV (A. Manninen, personal communication). Many, if not all, epithelial cells also express integrin α6β4. The β4-subunit is uniquely found in epithelial cells and, unlike most other epithelial integrins, interacts on the cytoplasmic side with cytokeratins, rather than the actin cytoskeleton. Integrin α2β1 is a collagen receptor, while α3β1 and α6β4 are receptors for multiple isoforms of laminin. The various αV-containing integrins are receptors for ligands containing the binding sequence arginine–glycine–aspartate (RGD), such as fibronectin and vitronectin. They may also play a role in activation of transforming growth factor β (TGFβ), which is important in epithelial repair and other processes. The MDCK cell line, for example, expresses α2β1, α3β1, α6β4, and several αV-containing integrins, with α3β1 and α6β4 mediating adhesion to different laminins, and with αVβ3 (and possibly other αV integrins) activating TGFβ to turn on specific laminin expression during wound-healing. In the kidney tubule, the complement of integrins expressed varies along the nephron, as does the expression of their extracellular matrix ligands, underlining their involvement not only in cell adhesion, but also in differentiation.
In adherent cells, most integrins facilitate adhesion through dynamic interactions with the actin cytoskeleton. Linkage to actin is mediated by adapter protein complexes that bind to integrin cytoplasmic tails and then to actin. Proteins found in these complexes include talin, which binds directly to integrins and activates their adhesive properties, paxillin, α-actinin, and vinculin. Studies of migrating cells suggest that initial adhesive interactions occur through small “focal complexes” that form on leading lamellipodia and are linked to branched actin through the action of the Rac1, a small GTPase of the rho family. As the cell moves over these contacts, they mature into larger “focal adhesions” that associate with robust actin stress fibers (at least in culture) controlled by another GTPase RhoA and its effectors. While the general elements of this model have been somewhat validated in epithelial cells during wound healing, the status of focal complexes and focal adhesions in mature polarized epithelia of the kidney and elsewhere remain, for the most part, unexplored. As mentioned previously, α6β4 is a novel integrin, in that it is epithelial-specific and is capable of assembling adhesion complexes that interact with the cytokeratin cytoskeleton. In normal skin, α6β4 is an essential part of hemidesmosomes. These are large protein complexes containing a second transmembrane protein BP180 in addition to α6β4, as well as the cytoplasmic proteins BP230 and plectin that interact with cytokeratin filaments. The type of hemidesmosome found in the skin (type I) is visible as a dense plaque on the cytoplasmic surface of the basal plasma membrane under the electron microscope. In the kidney such structures have not been reported. However, it is possible that a less developed type of hemidesmosome that is not apparent ultrastructurally (type II) is present in the kidney, although this has not been examined.
In addition to their role in mechanical adhesion, focal complexes and focal adhesions are also platforms for signaling. A variety of kinases including, notably, focal adhesion kinase (FAK) and members of the src family of tyrosine kinases, associate with integrin adhesion complexes and are activated by binding to the extracellular matrix. Subsequent signals then activate downstream serine/threonine kinases, such as integrin-linked kinase (ILK), and MAP kinases, such as ERK, to regulate a diverse range of processes including proliferation, migration, and apoptosis. Indeed, at least 180 different cytoskeletal, adapter, and signaling proteins are known to be associated with integrin adhesion complexes, depending on the cell type and circumstances.
In addition to integrins, other membrane proteins are involved in epithelial cell adhesion to the extracellular matrix, including dystroglycan, a laminin receptor, and possibly a membrane-bound form of the Lutheran antigen. There is evidence that glycolipids may also serve as transient laminin receptors. While it is not proven that any of these receptors play a direct role in epithelial polarization, they may act indirectly by affecting assembly of the basal lamina.
Biogenesis of Epithelial Polarity
In the kidney, polarization of epithelial cells occur under two different circumstances: early development of the tubular epithelium; and repair of an existing epithelium following injury. In mammals, formation of the renal tubular epithelium is initiated by induction of the metanephric mesenchyme by the invading ureteric bud. Following induction, cells of the mesenchyme form aggregates known as condensates, and these subsequently differentiate into polarized epithelial cells facing a central lumen. Extension of this lumen and further, more specialized, differentiation of epithelial cells eventually forms the nephron. In the case of injury by, for example, nephrotoxic substances or ischemia, the existing polarized tubular epithelium is disrupted in spots. Cells at the periphery of these damaged areas then convert from relatively sessile cells polarized along an apical-to-basal axis to flatter, more migratory cells that now have front–rear rather than apical–basal polarity and the capacity to proliferate, enabling them to fill gaps in the epithelium. Once continuity has been achieved, apical–basal polarity is restored. While front–rear and apical–basal polarization would seem to be quite distinctive, there is evidence that many of the important molecules and signals are shared. Indeed, as we are now beginning to understand, even front–rear polarization during injury repair is a close mechanistic cousin of apical–basal polarization.
In the following sections, our current understanding of the mechanism of epithelial polarization will be described, after a brief introduction to the predominant experimental system used to study these processes.
In Vitro Systems
The kidney’s complicated architecture and cellular heterogeneity renders it a poor substrate for studies designed to examine dynamic cell biological processes. Over the past four decades, the vast majority of research into the mechanisms through which epithelia generate and maintain their polarized phenotype has made use of several continuous lines of cultured epithelial cells. These cell lines retain many of the differentiated properties of their respective parent tissues in vitro . Thus, LLC-PK1 cells resemble the proximal tubule (although their precise origin is uncertain). Similarly, Caco-2, HT-29, and T84 cells behave like their progenitors, the colonocytes of the large intestine. Most importantly for this discussion, they manifest in culture the biochemical and morphologic features of the polarized state. Perhaps the best characterized and most heavily used of these culture models is the Madin–Darby canine kidney (MDCK) cell line ( Figure 1.3 ). MDCK cells were originally derived from normal dog kidney in 1959, and grown in culture as a partially transformed line; that is, MDCK cells grow immortally as a monolayer and will not form tumors in nude mice. Although their precise point of origin along the nephron is not entirely clear, their physiological and morphological properties suggest that they derive from cells of the thick ascending limb, distal tubule or collecting tubule.

The first clues to the polarized nature of the MDCK cell line came from the direct observation of these cells’ capacity for vectorial transport. When grown on impermeable substrata, MDCK cells form domes (also called blisters or hemicysts). Physiological studies have demonstrated that domes develop as a result of the transepithelial transport of solutes from the apical media to the basolateral surface. Water that passively follows these solutes results in the generation of the fluid-filled blisters. It is fair to say that domes arise in regions where the cells have literally pumped themselves up off the dish. In keeping with this dramatic propensity for unidirectional solute movement, each MDCK cell manifests a polarized distribution of ion transport proteins, including several routes for sodium entry into its apical membrane, and approximately one million molecules of the Na + /K + -ATPase in its basolateral plasmalemma. The popularity of MDCK cells for polarity research developed out of the seminal observations of Rodriguez-Boulan and Sabatini in 1978. In studies of enveloped virus budding from infected MDCK cells, these investigators found that influenza virus assembles at, and buds from, the apical cell surface ( Figure 1.3 ). Of even greater significance was the demonstration that the spike glycoproteins which populate the membranes of these viruses accumulate preferentially at the cell surface from which budding is to occur. Thus, the influenza hemagglutinin (HA) protein is predominantly on the apical surface early in infection. Similarly, the G protein of vesicular stomatitis virus (VSV) is almost exclusively basolateral in infected cells. The viral proteins provided investigators with the first experimentally manipulatable system for the study of membrane protein sorting. A large number of studies have subsequently elucidated the sorting of many endogenous MDCK cell proteins, as well as exogenous proteins expressed from vectors. This system remains the most thoroughly investigated paradigm and, as will be detailed below, has yielded important insights into the nature of pathways and signals that participate in membrane protein targeting and the overall biogenesis of epithelial polarity.
More recently, investigators have endeavored to develop new cell lines to study particular aspects of renal cell biology. For example, immortalization genes from human papillomavirus or a hybrid between adenovirus and SV40 have been used to create permanent cell lines from human proximal tubule cells. These lines are of particular interest because of the proclivity of the proximal tubule to suffer injury following ischemic insult. The cell lines retain some differentiated characteristics of the proximal tubule, including expression of brush border markers and sodium dependent/phlorizin-sensitive sugar transport. Cultures of cell lines derived in this fashion are not, however, always able to stably maintain the uniform morphology of a simple epithelium, limiting their usefulness for studies of epithelial polarity. More promising results have been obtained using cell lines derived from mice constitutively expressing immortalization factors as transgenes. Among these is the BUMPT-306 line derived from the mouse proximal tubule. While certainly not perfect, this line does grow as a simple epithelium, and has the added advantage of providing an in vitro correlate to in vivo mouse experiments.
The study of epithelial polarization using cell lines has been facilitated by culturing cells in configurations that more closely resemble in vivo conditions ( Figure 1.4 and 1.5 ). For example, many varieties of epithelial cells can be grown on permeable filter supports. Originally, these were designed to mimic the Ussing chamber used for physiological studies, but later turned out to also be very useful for biochemical and morphological experiments. In their most common commercially-available configuration, these supports are composed of polycarbonate filters with pore sizes typically in the range of 0.4 μm that form the bottom cup. The cup is then suspended in a plastic well containing culture medium, and medium is also added to the inner compartment of the cup ( Figure 1.4 ). Cells are plated on the upper surface of the filter. When a confluent monolayer is formed, it effectively creates a barrier between media compartments. The medium in the interior of the cup bathes the epithelial apical surface, whereas the basolateral surface communicates with the exterior media compartment through the pores of the filter ( Figure 1.4 ). As epithelial cells in the kidney and other organs would normally receive most of their nutrition from the basolateral (serosal) surface, permeable supports are, in a sense, a more natural growth environment than impermeable tissue culture plastic or glass. Indeed, there is some evidence that epithelial cells are more polarized in filter cultures than on solid substrata. Furthermore, the use of filters for the culture of epithelial cells permits investigators simultaneous and independent access to the apical and basolateral plasmalemma surfaces. This useful capacity has been extensively exploited in the experiments described in the protein sorting section of this chapter.


In addition to permeable supports, a number of investigators also culture renal and other epithelial cell lines embedded in a gel of collagen type I or other extracellular matrix molecules ( Figure 1.5 ). These are called three-dimensional (3D) cultures, to distinguish them from more common two-dimensional (2D) cultures on either solid or permeable surfaces. As with permeable supports, the idea behind 3D cultures is that placing the epithelial cell in an environment in which it is surrounded by extracellular matrix similar to that of the interstitium more closely resembles the in vivo environment. While that conclusion is subject to debate, there is no doubt that certain epithelial phenotypes are more readily expressed in 3D than in 2D cultures. Nevertheless, these phenotypes are often slow to develop, frequently taking 7–10 days, and may occur asynchronously; this, and the inaccessibility of the cultures, somewhat limits their usefulness for biochemical studies. With the advent of high resolution confocal fluorescence microscopy and the wide array of fluorescent proteins and probes, the impact of this limitation is lessened. In the case of MDCK cells, individual suspended cells develop into polarized cysts or, when stimulated with certain growth factors, tubules. As will be described below, use of 3D cultures has led to important fundamental observations about epithelial cell polarization. As a final note on the experimental use of 2D and 3D culture modalities, it is important to point out that formation of polarized cysts in 3D may be most closely analogous to the formation of the primordial kidney epithelium from condensed metanephric mesenchyme during development. In contrast, in vitro polarization of kidney epithelial cells in 2D cultures is more akin to the repair of existing kidney tubular epithelia following injury, a scenario that requires the spreading, migration, and proliferation of cells on a pre-existing surface to re-establish a contiguous epithelium.
Polarization Mechanisms: Spatial Cues from Cell Adhesion
It is now understood that not only epithelial cells but, indeed, all cell types are capable of polarizing in response to signals from the environment. Migrating cells of all types, for example, are polarized in the direction of migration in response to chemotactic or haptotactic gradients and even dividing cells can polarize through unequal division of their cytoplasm upon cytokinesis. Epithelial cells are unique, in that their polar organization is stable over time and the apical–basal axis of polarity exhibited by each cell in the epithelium is parallel to the axes of its neighbors. These features permit epithelial cells to form an asymmetrically organized, semipermeable surface that defines the borders of tissues in complex organisms, and separates the inside of the body from the outside environment, while helping to create and maintain distinctly different milieus on each side. The mechanism of epithelial cell polarization depends on specific spatial cues that cause the assembly and asymmetric reorganization of the intrinsic polarization machinery. The spatial cues are adhesive interactions between neighboring cells and the extracellular matrix, while the intrinsic machinery consists of primarily three polarity complexes called Par, Scribble, and Crumbs. If the pattern of cell adhesion is asymmetric, then the response of the cell is asymmetric, and a polar phenotype results ( Figure 1.6 ). This section will describe the specific nature of the cell–cell and cell–extracellular matrix adhesive interactions. The mechanisms by which adhesion is translated by the intrinsic polarization machinery into a polarized epithelial cell with distinct apical and basolateral plasma membrane domains will be presented in subsequent sections.

As mentioned previously, polarization of kidney epithelial cells occurs during initial differentiation of the primordial tubular epithelium from condensed mesenchyme, and after disruption of an existing mature epithelium following injury. These two situations are somewhat mimicked in vitro by, respectively, 3D culture and 2D culture of renal epithelial cell lines. It is important to recognize that the pattern of spatial cues provided by adhesive interactions is different in these two situations. In the developing kidney epithelium, cell–cell interactions in the condensed mesenchyme are believed to precede meaningful cell matrix interactions ( Figure 1.7 ). These condensates are spatially differentiated: cells at the peripheries of the condensates have both a “free” plasma membrane domain facing the outside of the condensates and the undifferentiated mesenchyme, and an “attached” plasma membrane domain in contact with other cells of the condensate. Following this rudimentary polarization, the adherent mesenchymal cells in the condensate become more polarized, eventually forming a lumen and reorganizing into a simple epithelium attached to a basement membrane. Similarly, in 3D collagen gel cultures of MDCK cells, individual cells are initially suspended in the gel. Spatial cues from collagen adhesion at this point are isotrophic rather than asymmetric, in that any signals impinging on the cell are unbiased with regard to direction ( Figure 1.6 ). As soon as that cell divides, an asymmetric spatial cue is elicited from the resulting cell–cell adhesion, because the cells remain attached. This situation persists through subsequent divisions until at some point a basement membrane is formed, and the individual cells create a lumen. In contrast, in a wounded epithelium and in 2D culture of epithelial cell lines, the persistent spatial cue from the extracellular matrix substratum is asymmetric, and then an additional although qualitatively different asymmetric spatial cue develops as cell–cell adhesion is restored ( Figure 1.6 ). The take-home message from this discussion is that epithelial cells utilize both cell–cell and cell–extracellular matrix interactions as asymmetric spatial cues to polarize. The order in which these cues are provided to the cell may yield qualitatively different results but, in the end, both are required for full apical–basal polarization.

The hierachical relationship between cell–cell and cell–substratum interactions in epithelial cell polarization is illustrated best by a description of early experiments utilizing the MDCK cell line. MDCK cells cultured in suspension as individual cells lack polarized plasma membrane domains. Upon attachment to a substratum in 2D culture, apical proteins are restricted to the free or apical surface, while basolateral proteins are distributed over the entire plasma membrane. This situation persists as the cell density increases and initial cell–cell contacts form until, as the cells reach confluency to form a true epithelium, basolateral proteins also become completely polarized.
The relative roles of cell–cell and cell–substratum interactions can also be dissected by culturing MDCK cells in medium containing reduced amounts of calcium. If cells are cultured on collagen-coated surfaces in medium with less than 5 μM calcium, they attach to the substratum but formation of cell–cell contacts mediated by the calcium-dependent adhesion protein E-cadherin is inhibited. Cells assume a rounded morphology with no appreciable lateral membrane. In this situation, an immature apical surface forms. Microvilli are decreased in number, and expression of apical proteins on the cell surface, although reduced in quantity, remains polarized to the free surface. Basolateral proteins, in contrast, are not polarized in cells cultured in medium containing low calcium concentrations. However, when the calcium concentration is raised to normal values (1.8 mM), then cell–cell contacts rapidly form and basolateral proteins polarize.
The culturing of MDCK cells as multicellular aggregates in suspension also permits the relative effects of cell–cell and cell–substratum interactions to be independently evaluated. Under these conditions, which are different from the 3D culture described previously because the cells are surrounded only by culture medium, aggregated cells gradually form cysts with small central lumina. In the absence of recognizable cell–substratum contact, both apical and basolateral polarization occurs, with the apical surface facing the outside of the cell aggregate. At this time, the tight junctional protein ZO-1 is found distributed over the entire lateral membrane, where cell–cell contacts occur. As the lumen forms, the cells secrete and deposit type IV collagen and laminin into the lumen. Interaction with this interior extracellular matrix then triggers redistribution of ZO-1 to the point of intersection between the apical and lateral membrane. Thus, as in the reduced calcium experiments, cell–cell and cell–substratum interactions have somewhat independent but complementary effects on cell polarization.
The adherens junction provides the spatial cue from cell–cell interactions leading to polarization of epithelial cells. One of the most important components of the adherens junction is E-cadherin, the calcium-dependent homophilic cell adhesion molecule discussed previously. Suppression of E-cadherin expression by small-interfering RNAs prevents polarization, clearly implicating it in the polarization process. However, other cell–cell adhesion proteins such as nectins and JAMs may be important as well. Nectins are transmembrane adhesion molecules containing immunoglobulin-like extracellular domains. When epithelial cells transition from a migratory phenotype, as they exhibit during wound healing, nectins may be one of the earliest molecules in nascent cell–cell adhesions. Homophylic interaction of nectins between neighboring cells activates a variety of signaling pathways, among which are those involving the small GTPases Cdc42 and Rac1. This, in turn, leads to reorganization of the cortical actin cytoskeleton and, consequently, facilitates the formation and stabilization of E-cadherin-mediated contacts, creating the mature adherens junction. During this process, both nectins and JAMs may initiate the asymmetric activation of the intrinsic polarization machinery. Nectins and JAMs bind Par3, an element of the Par polarization complex (see below). As the adherens junction matures, other polarization complexes bind and specification of the apical and basolateral plasma membrane domains occurs, with the dividing line located just at the adherens and tight junctions, collectively referred to as the apical junctional complex. At the same time, a complex of proteins known as the exocyst is also assembled at this location. The exocyst is a kind of tethering complex designed to capture transport vesicles carrying newly synthesized membrane proteins. Delivery of these proteins to this region of the plasma membrane then facilitates the development of the apical and basolateral surfaces.
It is likely that the spatial cue emanating from cell–substratum adhesive interactions originates from integrin-mediated cell interactions with the basement membrane protein laminin. In the developing kidney, conversion of condensed mesenchyme into a polarized epithelium is dependent on laminin. The laminin α1-subunit and integrin α6β1, a laminin receptor, are first detected in the induced mesenchyme following condensation. Laminin localizes to the periphery of the condensate, suggesting that the crude polarization caused by condensation leads to polarized laminin secretion to the periphery. In organ culture antibodies against laminin α1 or the integrin α6-subunit block formation of a polarized epithelium from condensed mesenchyme, suggesting that their involvement in epithelial differentiation is critical.
Results from a variety of other experimental systems generally support the conclusion that laminins and their integrin receptors play a role in epithelial polarization. Mutations in either integrin or laminin subunits lead to disruption of epithelial differentiation and polarization in the nematode Caenorhabditis elegans , and the fruit fly Drosophila melanogaster . Expression of laminin in the early mouse embyro generally coincides with development of epithelial tissues. In embryoid bodies derived from cultures of aggregated embryonic stem (ES) cells, an LM-111 containing basement membrane forms between the endoderm and the polarizing inner cell mass cells. When ES cells deleted of both laminin γ1 alleles are aggregated into embryoid bodies, the inner cell mass forms, but does not polarize. Laminin has also been implicated in the polarization of MDCK cells. When MDCK cells are cultured in 3D collagen gels for 7–10 days, they form polarized cysts with the apical surface facing the lumen, and the basal surface facing the extracellular matrix. Under these conditions, the cells secrete LM-511 and form a discrete layer closely associated with the basal surface, resembling an assembled basement membrane. When MDCK cells are treated either with a function-blocking anti-β1 integrin antibody or express dominant-negative Rac1 during cyst formation, the laminin layer does not form properly, although laminin is secreted, and the cells display an inverted and somewhat disorganized polarity with apical antigens expressed on surfaces facing the extracellular matrix. Addition of excess exogenous LM-111 to the collagen gel partially rescues both basement membrane assembly and correct polarization in cells expressing dominant-negative Rac1, possibly by driving laminin assembly adjacent to the basal plasma membrane. Inverted polarization of the cyst caused by anti-β1 integrin can also be rescued by expression of constitutively-active Rac1. These experiments suggest that a “serpentine” signaling pathway exists, leading from ligation of a β1 integrin outside the cell, to Rac1 activation inside the cell, to laminin assembly outside the cell, and finally to polarization. It is important to emphasize that the perturbations that lead to inverted polarity do not seem to affect the segregation of apical and basolateral proteins in individual cells, but instead affect the orientation of apical–basal axes in the cyst. It is unclear how intracellular signaling events can cause laminin assembly. Recent findings have implicated the polarization signaling molecule Par1b and dystroglycan, a non-integrin laminin receptor, in effecting laminin assembly, but the mechanisms are not apparent.
Polarization Mechanisms: The Intrinsic Polarization Machinery
In epithelial cells, the intrinsic polarization machinery has traditionally been separated into three so-called polarity protein complexes that function collectively to control the formation of apical and basolateral domains of the plasma membrane. Many of the components of the polarity complexes were initially identified in mutant screens in Caenorhabditis elegans and Drosophila melanogaster , but homologs that function in an essentially identical manner have also been identified in mammals. The first, and perhaps most important, polarity complex generally associated with apical surface determination is the Par complex composed of Par3, Par6, atypical protein kinase C (aPKC), and the small-GTPase Cdc42 ( Figure 1.8 ). A second apical complex is the Crumbs complex, containing in mammals the transmembrane protein Crumbs (Crb3 in the kidney) associated with Lin-7 (PALS1), and PALS1-associated tight junction protein (PATJ). The final complex, which is located on the lateral surface in polarized epithelia, is the Scribble complex composed of Scribble (SCRIB), discs large (Dlg), and lethal giant larvae (Lgl). In the case of the Par and Crumbs complex, physical interactions between the components have been demonstrated, while interactions of Scribble complex components have been implied by genetic experiments. In addition to these polarity complexes, other proteins are involved in regulating apical–basal polarization in mammals or lower organisms including, in particular, Par1b and LKB1 (Par4). In the core polarity protein complexes, only aPKC and Cdc42 have enzymatic activities: the former a serine/threonine kinase; and the latter a GTPase-mediated molecular switch, while the others are largely scaffolds with a myriad of binding domains, including on many PDZ domains, that facilitate association with the apical junctional complex of the polarized cell.

The mechanisms by which these complexes function to help establish and maintain apical–basal polarity is often described as a process of mutual antagonism or inhibition that creates and maintains a particular balance between the amounts of distinct apical and basolateral plasma membranes, with the “front line” of this battle being the apical junctional complex ( Figure 1.8 ). While the concept of mutual antagonism is not inaccurate, how this translates into intuitively comprehensible mechanisms has, until recently, not been obvious. Studies in Drosophila have highlighted the critical role of Par3 (Bazooka or Baz in Drosophila ) and have, to some extent, called into question the idea of distinct, somewhat independently functioning polarization protein complexes. A compelling model proposes that initiation of polarization commences when Par3 (Baz) associates with the region of cell–cell adhesion that will become the adherens junction, prior to the concentration of DE-cadherin (the Drosophila version of E-cadherin) in that location. Association is facilitated by phosphoinositides. Once the adherens junction forms, then Par3 binds Par6 and aPKC, and Cdc42 is activated. Activation of aPKC then leads to phosphorylation of Par3, as well as Crb and Lgl, following the delivery of Crb to the plasma membrane through vesicles. The phosphorylation of Par3 on serine 980 by aPKC weakens the interaction between Par3 and aPKC, as well as the interaction between Par3 and the Crb complex protein PALS1 (Lin-7, Stardust or Sdt in Drosophila ). These changes permit formation of the apical complex Crb–PALS1–PATJ, while interactions between Crb and Par6 prevent association of Par3 with the forming apical surface. At the same time, Par1-mediated phosphorylation of Par3 creates binding sites for the scaffold 14-3-3, and the subsequent Par3-14-3-3 interaction further inhibits the association of Par3 with Par6/aPKC. Since Par1 is localized to the lateral surface, this prevents Par3 from assembling on the lateral surface. Lgl is also phosphorylated by aPKC, which prevents its apical localization. With Dlg and Scrib, it then contributes to maintaining the identity of the lateral membrane in a manner that is poorly understood.
In all likelihood this scenario, derived primarily from experiments on Drosophila , is also valid in renal epithelial cells. Studies in MDCK cells, in particular, have illuminated a number of steps and, in some instances, provided additional critical details. As mentioned previously, nascent cell–cell contacts may be initiated by nectins and JAMs prior to the coalescence, stabilization, and assembly of the adherens junction nucleated by E-cadherin and its associated proteins. Nectins and JAM, which bind Par3, may serve to localize Par3 to the forming junction. In addition to its roles in facilitating the assembly of the other polarization complexes as described earlier, Par3 binds phosphoinositides in the membrane, as well as the phosphoinositide phosphatase PTEN (phosphatase and tensin homolog). This phosphatase converts phosphatidylinositol (3,4,5)P 3 (PIP3) on the inner leaflet of the membrane to phosphatidylinositol (4,5)P 2 (PIP2). The latter lipid then facilitates the development of the apical surface by binding annexin2, which then recruits Cdc42, leading to the cascade of events involving aPKC described earlier. The importance of the conversion of PIP3 to PIP2 in the formation of the apical surface was highlighted dramatically when exogenous PIP2 was added to the basolateral pole of mature MDCK cell cysts in 3D culture. Incorporation of PIP2 into the basolateral plasma membrane led to gradual extension of the apical surface to the basal pole with collapse of the lumen.
The description of the polarization process so far has focused only on events initiated by cell–cell adhesion. However, as was described in the previous section, adhesion of epithelial cells to the basement membrane is also a key part of polarization, functioning particularly to orient the apical–basal axis in a coordinated fashion in neighboring cells. How basement membrane adhesion and, particularly, adhesion to laminin does this is not clear, but it likely involves the microtubule cytoskeleton ( Figure 1.2 ). In individual MDCK cells adherent to a substratum in 2D culture, the organization of microtubules resembles that in other nonepithelial adherent cells, in that microtubule growth is initiated from a juxtanuclear centrosome or microtubular organizing center, and extends radially towards the cell surface. Once cell–cell adhesion is initiated, the microtubule organizing activity begins to disperse to the cell periphery and, as the cell polarizes, it relocalizes diffusely to the apical surface. In response to this, microtubules reassemble along the apical–basal axis with the plus (growing) ends pointing basally. Networks of microtubules also form roughly parallel to the basal and apical surfaces ( Figure 1.2 ). Reorganization of the microtubules depends on a family of GTPases known as septins, which provide directionality to the process, while stabilization and anchoring depend on the plekstrin homology-like domain family A protein LL5, microtubule plus-end tracking proteins, and the APC (adenomatous polyposis coli) tumor suppressor. LL5 anchors microtubule plus ends to the basal plasma membrane by associating with integrin-mediated cell adhesions to laminin. Furthermore, the polarity protein Par1b, which has been implicated in assembly of the laminin network in the basement membrane, is also important in the formation of the apical surface in 3D cysts, reportedly through a mechanism that depends on signaling from the extracellular matrix. Thus, there appears to be a signaling network linking laminin assembly and microtubule reorganization to formation of the apical surface in the correct location. How these microtubules mechanistically contribute to polarization is not clear, but it is known that they provide tracks for vesicle transport that are necessary for formation of the apical lumen through its population with specific proteins, a process facilitated by septins.
Ultimately, cooperation of extrinsic spatial cues and the intrinsic polarization machinery creates a polarized cellular infrastructure composed of adhesive interactions, a spatially-differentiated cytoskeleton, and asymmetrically disposed signaling complexes. Completion of polarization, and its maintenance during cell and tissue function, then depends on the targeting of specific membrane proteins to the apical and basolateral plasma membrane domains. How this is accomplished is the subject of the following section.
Sorting Pathways
One of the first, and perhaps most easily addressed, questions presented by the phenomenon of epithelial polarity relates to where, within the cell, sorting occurs. The membrane proteins that populate the apical and basolateral plasmalemmal domains are all synthesized in association with the membranous elements of the rough endoplasmic reticulum (RER). It has further been shown that after their co-translational insertion into the membranes of the RER, apically- and basolaterally-directed proteins share the same cisternae of the Golgi complex as they transit the secretory pathway en route to their respective sites of ultimate functional residence. Immunoelectron microscopic studies performed on MDCK cells doubly infected with the VSV and influenza viruses revealed, through double labeling, that the influenza HA protein and the VSV G protein could be co-localized throughout the cisternae of the Golgi complex.
This observation was confirmed and extended through a series of elegant biochemical studies. It had previously been shown that when cells are incubated at 20°C newly synthesized membrane proteins accumulate in the trans-most cisterna of the Golgi complex. Elevating the temperature to 37°C relieves this block, and allows the proteins to proceed to the cell surface. By examining the nature of the complex N-linked glycosylation associated with the VSV G protein, it was demonstrated that sialic acid residues are added in the 20°C compartment. These investigators took advantage of the fact that in addition to the HA protein the membrane of the influenza virus contains a neuraminidase in their efforts to determine whether segregation of the apically directed influenza proteins from the basolaterally targeted VSV G protein occurs before or after the 20°C block compartment. They found that in singly infected cells incubated at 20°C, the VSV G protein became heavily sialylated. In contrast to this, when cells which had been doubly infected with both the VSV and influenza viruses were incubated at 20°C, little if any sialic acid could be detected on the newly synthesized VSV G protein. These results demonstrate that as late as the 20°C block compartment, which corresponds to the trans-most cisterna of the Golgi complex, the newly synthesized apical neuraminidase and basolateral VSV G protein are still intermingled and capable of physical interaction. The segregation of these two classes of proteins from one another must, therefore, occur at or after this subcellular locus. It is interesting to note that immunoelectron microscopic studies of endocrine cells reveal that proteins destined for packaging in secretory granules are separated from those bound for constitutive delivery to the cell surface in the trans-most cisterna of the Golgi complex. Observations such as these have prompted investigators to speculate that this compartment, which is referred to as the Trans Golgi Network (TGN), might be the site of several intracellular sorting events. More recently, it has been shown that sorting may occur as well at the level of the recycling endosome. Loading endosomes with HRP-conjugated transferrin, and subsequently disrupting endosome function through the deposition of peroxidase reaction product, prevents the surface delivery of a subset of newly synthesized basolateral membrane proteins. While the delivery of the VSV G protein to basolateral plasma membrane is prevented by this ablation of the recycling endosome, the delivery of the newly synthesized Na + /K + -ATPase to the basolateral surface is unaffected by this maneuver. Thus, it would appear that proteins can pursue more than one route from the TGN to the basolateral membrane domain.
Three epithelial sorting pathways can be imagined ( Figure 1.9 ). In the direct model, sorting would take place prior to cell surface delivery. Segregation of basolateral from apical proteins would be completed intracellularly, and proteins would never appear, even transiently, in the inappropriate membrane domain. The random sorting scheme dictates that no separation of apical from basolateral proteins occurs prior to arrival at the cell surface. Following their insertion into the plasmalemma, proteins that find themselves in the wrong surface domain would be removed by endocytosis, and either transcytosed to the proper surface or degraded. Finally, the indirect paradigm predicts that all newly synthesized plasmalemmal proteins initially appear together, either at the apical or basolateral membrane. The proteins for which this delivery is correct would be retained in that membrane domain, while those which had been mis-delivered would be internalized and transcytosed to their sites of ultimate functional residence.

These three models, although perhaps somewhat simplistic, are valuable for the relative ease with which they can be experimentally distinguished. Over the past two decades a great deal of effort has been invested in identifying which of these routes is, in fact, operational. The rather surprising answer appears to indicate that the sorting pathway pursued varies among different cell types, and even among different proteins within the same cell type.
Technical Approaches
Much of the early research into the nature of epithelial sorting pathways was carried out on MDCK cells that had been infected with the VSV or influenza viruses. The infected cells produced massive quantities of viral proteins, and retained their polarized distribution throughout at least the initial stages of the infection. These properties greatly facilitated the detection of cohorts of newly synthesized membrane proteins in the pulse chase protocols generally employed to monitor the polarity of cell surface delivery. Pulse labeling experiments demonstrated that the VSV G protein was not accessible to apically added antibodies at any point during its post-synthetic processing. In the case of the influenza HA protein, the converse was true. Proteases or antibody probes added to the media compartment bathing the basolateral surfaces of MDCK cells grown on filters could not cleave or interact with this polypeptide during its journey to the apical cell surface. From these results, it was concluded that the direct model of sorting applied for at least these two proteins in MDCK cells.
Other labeling tools have also been brought to bear on the study of sorting pathways. The N-hydroxysuccinimidyl (NHS) derivative of biotin is a membrane impermeant molecule that will covalently combine with the ε-amino groups of exposed lysine residues. Proteins thus modified are substrates for precipitation or detection with avidin-conjugated secondary reagents. These tools can be used to follow the fate of large numbers of membrane proteins that have been exposed at one or other cell surface to the NHS biotin compound. Using such a protocol, it has been demonstrated that several MDCK cell apical and basolateral membrane proteins are directly targeted to their appropriate membrane domains.
Advances in the development of genetically encodable probes have also permitted sorting pathways to be visualized directly. By creating chimeras that incorporate photoactivatable versions of the green fluorescent protein (GFP), the trafficking of newly synthesized proteins of interest can be observed in intact cells in real-time. Alternatively, the SNAP tag similarly permits newly synthesized cohorts of proteins to be observed in pulse chase protocols. The 20 kDa SNAP-Tag is a modified version of the DNA repair protein 0 6 -alkylguanine-DNA alkyltransferase, which cleaves para-substituted benzyl guanines (BGs) by covalently transferring the substituted benzyl group to its active thiol. The resulting thioether bond irreversibly prevents the reacted SNAP-Tag from participating in any further labeling reactions. Fluorescent BG derivatives allow for the labeling and detection of SNAP-Tagged fusion proteins in either live or fixed cells. Through the combination of a “pre-pulse” blocking step with non-fluorescent BG, followed by selective labeling of newly synthesized protein with fluorescent BG, it is possible to follow a cohort of protein as it is synthesized and trafficked.
Sodium Pump Targeting
Further support for the direct pathway paradigm in MDCK cells came from studies on the sorting of the endogenous Na + /K + -ATPase. Filter grown MDCK cells which had been pulse-labeled with [35S]-methionine were exposed to the N-azidobenzoyl (NAB) derivative of ouabain at either their apical or basolateral surfaces during the course of a 90 minute chase. NAB-ouabain will bind to catalytically active sodium pumps with high affinity and, following UV photolysis, will become covalently incorporated into the protein backbone of the Na + /K + -ATPase α-subunit. By analyzing immunoprecipitates prepared from these cells using an anti-ouabain antibody, it was possible to demonstrate that no sodium pump in a state competent to bind ouabain ever appears at the apical surface. Subsequent studies using a SNAP-tagged version of the Na + /K + -ATPase expressed in the same MDCK cell line recapitulated the finding that the newly synthesized pump trafficks directly to the basolateral surface in these cells.
Another investigation of sodium pump sorting in a different clonal line of MDCK cells made use of the NHS biotin surface-labeling technique, and arrived at a conclusion diametrically opposed to the one described above. The results of this study indicated that the Na + /K + -ATPase is randomly delivered to the apical and basolateral plasmalemmal surfaces. The authors further suggested that stabilizing interactions with cytoskeletal elements which underly the basolateral, but not the apical, cell surface result in a much longer residence time for pump inserted into the basolateral domain. These studies are thus consistent with a model in which sodium pump is not sorted intracellularly, but instead achieves its basolateral distribution through a mechanism based on random delivery followed by differential stabilization.
The apparent discrepancy among these studies appears to be attributable to differences in the pathways and processes through which these closely related cell lines achieve the polarized distribution of the Na + /K + -ATPase. While one line targets the pump directly to its basolateral destination, the other delivers it randomly and depends upon cytoskeletal interactions to stabilize only the basolateral pool. Clearly, therefore, while cytoskeletal interactions may be sufficient to localize the Na + /K + -ATPase to the basolateral surface, they are not the sole mechanism involved in producing the sodium pump’s anisotropic distribution. Instead, they may act as a failsafe mechanism to back up and reinforce the initial biosynthetic sorting of the Na + /K + -ATPase, to ensure that its polarized distribution is attained and maintained.
The preceding discussion suggests that the direct scheme cannot be applied to all epithelia or even to all MDCK cell clones. An alternative system has been shown to apply to the liver, for example. Cell fractionation studies performed on liver by Bartles et al. reveal that several apical membrane proteins appear in the fraction corresponding to the hepatocyte basolateral plasma membrane prior to being delivered to the apical surface. This route has been especially well-documented for the polymeric immunoglobulin receptor (pIgR) expressed by hepatocytes. This 120 kDa polypeptide serves to carry dimeric IgA from the blood to the lumena of the bile cannaliculi. During its biosynthesis, the pIgR is transported directly from the TGN to the basolateral cell surface where it is available to bind dimeric IgA. Independent of any interaction with IgA the receptor becomes phosphorylated in the basolateral plasmalemma, and the phosphorylated form is internalized and carried by a transcytotic vesicle to the apical, or canalicular, surface. Following its insertion into the apical plasma membrane the ectodomain of the pIgR is cleaved and released into the bile as an 80 kDa protein referred to as secretory component. Association with secretory component helps to protect the bound IgA from intestinal proteases. Coupled with other results, the behavior of pIgR in hepatocytes supports the contention that apical membrane proteins arrive at their site of ultimate functional residence via obligate mis-delivery to the basolateral domain. This paradigm may not apply to all apical proteins in hepatocytes. Studies of the trafficking of apical members of the multidrug resistance (MDR) family of transport proteins indicate that these polytopic membrane proteins do not make an appearance at the basolateral surface en route to the apical membrane. Thus, within a single polarized cell type multiple trafficking routes can be employed to target different proteins to the same place.
A combination of the direct and indirect paradigms seems to be involved in membrane protein delivery in cultured intestinal cells. The Caco-2 line of human colon carcinoma cells can be grown on filters and subjected to the NHS-biotin labeling protocol described above. Such experiments reveal that the basolateral protein followed is vectorially targeted. Analysis of the apical polypeptides produced a somewhat more complicated picture. A fraction of these proteins appeared to transit through the basolateral plasmalemma prior to their apical delivery. The remainder of the apical proteins studied in this sampling were sorted intracellularly and inserted directly at the apical domain. Related and somewhat more complicated results have been gathered from studies on the biogenesis of brush border hydrolases by colonocytes in situ .
To complete this already confusing picture it is necessary to return to a discussion of targeting studies in MDCK cells. A cDNA encoding the pIgR has been expressed by transfection in this cell line. Remarkably, the sorting pathway pursued by this protein in the cultured renal epithelium is apparently identical to the rather baroque scheme that characterizes its route in hepatocytes. From the TGN, the pIgR travels to the basolateral surface from which it is internalized and subsequently transcytosed to the apical pole or recycled to the basolateral side. These observations demonstrate that an obligate mis-delivery pathway is either created or simply revealed in MDCK cells expressing the pIgR. Results from studies employing real-time imaging to follow the surface delivery of a class of newly synthesized apical proteins support the possibility that this indirect pathway does, in fact, exist constitutively in MDCK cells. As discussed in greater detail in the section on sorting signals, proteins whose attachments to the membrane are mediated by covalent linkage to glycosylphosphotidyl inositol (GPI) lipids tend to accumulate in the apical domains of most varieties of polarized epithelial cells. A study that employed live cell imaging to track the sorting of GPI-linked proteins found that these proteins were delivered to the basolateral surface, after which they were endocytosed and carried to the apical plasmalemma. It is worth noting, however, that subsequent studies found that several GPI-linked proteins were delivered directly to the apical membrane in MDCK cells, without making any detectable transient appearance at the basolateral surface.
This apparent diversity of sorting pathways is perhaps not as surprising as it first appears. The relative flow of membranous vesicles from the Golgi complex to the two plasmalemmal surfaces in different epithelial cell types is likely to reflect a cell’s biologic mission, as well as the nature of the environment in which it functions. It appears, for example, that although hepatocytes produce copious quantities of secretory proteins, none are released directly into the bile. It has been proposed that newly synthesized membrane proteins depart the Golgi in the same transport vesicles that carry proteins destined for constitutive secretion. Were this the case, then cells which do not produce a secretory content targeted for one or another membrane domain may also lack a direct traffic of membrane vesicles directed from the Golgi to that domain. The full complement of plasmalemmal proteins might thus be forced by default to share the same carrier out of the Golgi, and to be sorted by transcytosis subsequent to cell surface delivery. Some hepatic apical membrane proteins may transit through the basolateral surface, because there is very little non-stop cargo traveling from the TGN to the apical domain in this particular cell type. The apparent multiplicity of sorting pathways available to different proteins within the same cell type may reflect specializations relevant to these proteins’ functions. Diversity may also arise from the nature of the signals and mechanisms that mediate these proteins’ polarized distribution. The potential contribution of this latter influence will be referred to again in sections to follow. The lack of a single answer or unifying solution to the problem of sorting pathways is a theme that carries through the entire study of epithelial polarity. A number of equally effective mechanisms appear to have evolved for segregating membrane proteins into distinct domains. It remains to be determined how these differing approaches benefit their respective tissues and contribute to the maintenance of their unique functions.
Regulation of Renal Transport Protein Function by Endocytosis and Recycling
The delivery of a protein to its site of functional residence in a domain of the plasma membrane does not end that protein’s involvement with the cellular sorting machinery. Cell surface proteins are subject to endocytic internalization, after which their fate is determined through processes that are mechanistically closely related to those that mediate biosynthetic sorting. Following their delivery to endosomes, endocytically internalized proteins can be recycled, meaning that they are returned to the same domain of the plasma membrane. Alternatively, they can be carried by transcytosis to the opposite plasma membrane domain. Finally, they can be targeted for degradation in the lysosome. The cellular machinery and molecular signals that govern these post-endocytic sorting processes appear to closely resemble those that mediate biosynthetic sorting.
From the perspective of the renal tubular epithelial cell, perhaps the most important implication of post-endocytic sorting relates to its role in the regulation of ion transport protein activity. The activities of a number of critically important transport proteins are controlled by regulated membrane insertion and endocytotic events. Included on this list are the aquaporin 2 water channel (AQP2), the ROMK potassium channel, and the ENaC sodium channel of collecting duct principal cells ; the V-type proton ATPase of collecting duct intercalated cells ; the Na,Cl co-transporter (NCC) of the distal convoluted tubule ; the Na phosphate co-transporter (NaPi), the Na proton exchanger (NHE3), and the Na + /K + -ATPase of the proximal convoluted tubule. These regulated trafficking events are precipitated by a variety of hormonal and physical stimuli, and involve the participation of a diverse collection of signaling cascades and trafficking proteins. While their details will be explored in far greater individual detail in the chapters that relate to each of the specific tubule segments and relevant transport systems, it is worth noting that these regulated trafficking processes exploit many of the biosynthetic sorting signals and components of the cellular trafficking machinery that are discussed in the following sections.
Sorting Signals
Rodriguez-Boulan and Sabatini’s 1978 observation that viral spike glycoproteins are targeted to opposite domains of polarized epithelial cells gave rise to the hypothesis that sorting signals – that is, the information required to direct a protein or proteins to a given subcellular location – might be wholly contained within the structure of the sorted proteins themselves. Evidence in favor of this contention has come from studies examining the distribution of viral membrane proteins expressed by transfection (rather than infection) in polarized cultured cells. A number of investigators have shown that the influenza HA protein, the VSV G protein, and related viral spike glycoproteins are sorted correctly in the absence of any other proteins encoded by viral genomes. It is apparent, therefore, that all of the addressing information necessary to produce the polarized distributions of these polypeptides must be embodied within the proteins themselves. It has further been shown that this information is almost certainly associated with the protein backbone, rather than with any post-translational modification. Cells whose capacity to add asparagine-linked sugar residues has been impaired, either through mutation or via treatment with tunicamycin, are nonetheless able to correctly target the viral spike proteins. Observations such as these have sparked an intensive search for the actual molecular information that specifies localization, and for the machinery that acts upon this information. It must be stated at the outset, however, that despite the rather confident and declarative tone of this section’s heading the identification and characterization of epithelial sorting signals and mechanisms is far from complete.
Several distinct classes of signals have been found to specify basolateral sorting. Perhaps the best characterized of these are short motifs that contain tyrosine residues and resemble or overlap with sequences involved in endocytosis. Work from a number of groups has suggested that sequences in the cytosolic tail of membrane proteins determine the rates at which these proteins are internalized. The presence of a tyrosine residue appears to be a critical determinant of the efficacy of an endocytosis signal. The rapid endocytosis of both the LDL receptor and the transferrin receptor, for example, is dependent upon the presence of short, tyrosine-containing sequences in these proteins’ cytoplasmic tails. Mutation of this tyrosine residue to any other amino acid vastly reduces the rates at which both of these proteins are internalized. The apically sorted influenza HA protein is normally endocytosed extremely slowly. Addition of a tyrosine residue to the cytosolic tail of the influenza HA protein causes it to behave like the LDL receptor or transferin receptor with respect to endocytosis – that is, it is rapidly internalized and recycled. When this altered form of the HA protein is expressed in MDCK cells it is detected predominantly at the basolateral plasma membrane. It would appear, therefore, that a signal that is permissive for endocytosis is also competent to mediate basolateral accumulation.
Studies of the VSV G protein reveal that its basolateral sorting is also driven by a tyrosine-containing motif. Uptake measurements suggest, however, that the VSV G protein is internalized relatively slowly, suggesting that its tyrosine-based motif confers basolateral targeting, but not rapid endocytosis. Mutagenesis studies of the tyrosine-modified influenza HA protein, as well as several other basolateral membrane proteins, indicate that while internalization signals and basolateral sorting signals can share the same critical tyrosine residues, they are not identical. Altering residues near the tyrosine can produce apically sorted influenza HA protein that is rapidly endocytosed, and basolateral HA protein that is internalized only slowly. Thus, basolateral and endocytosis signals can overlap, sharing one or more residues, but are clearly distinguishable from one another. Presumably, therefore, they must be interpreted by non-identical cellular machinery.
Data pointing to a similar conclusion have been gathered for Fc receptors. One of the Fc receptor isoforms includes a di-leucine sequence in its cytoplasmic tail. This sequence has been shown to function as an endocytosis signal, and it also appears to confer basolateral targeting when the protein is expressed in polarized cells. Once again, alteration of residues flanking the di-leucine motif demonstrates that the sequence requirements for basolateral sorting are distinct from those that specify internalization.
Tyrosine-containing basolateral sorting signals that are entirely distinct from recognizable endocytosis motifs have also been detected. The LDL receptor depends upon a basolateral sorting signal that bears no sequence resemblance to any known internalization motif. Although this motif includes a tyrosine residue, mutation of that tyrosine to phenylalanine still permits basolateral localization. A distinct tyrosine-containing motif appears to mediate the internalization of the LDL receptor. In the absence of the primary basolateral signal, this endocytosis motif can mediate a basolateral sorting function. Once again, however, with the exception of the tyrosine residue, the amino acids that contribute to the basolateral and endocytic aspects of this signal are distinct from one another.
Several basolateral sorting signals unrelated to tyrosine residues have also been reported. The well-characterized tyrosine-based endocytosis motif of the transferrin receptor is completely distinct from this protein’s basolateral targeting signal, which resides in a different portion of the cytoplasmic tail. The peptide processing enzyme furin cycles between the trans-Golgi network and the basolateral plasmalemma. Its trafficking to the basolateral surface appears to be driven by residues that are associated with a casein kinase II phosphorylation site. The invariant chain of the major histocompatibility class II complex is sorted to the basolateral membrane by virtue of the dihydrophobic sequence Met–Leu. Once again, endocytic internalization of this molecule is conferred by a similar dihydrophobic sequence, Leu–Ile, which is present at another position on the cytoplasmic tail. All of the basolateral sorting motifs discussed thus far function in the context of membrane proteins that span the bilayer once. As will be discussed below, a distinct cadre of molecular sequences appears to mediate the targeting of many ion transporters and other multispanning membrane proteins.
The list of identified basolateral sorting signals is considerably more extensive than the inventory of characterized apical membrane protein sorting signals. Perhaps the best studied member of this latter roster is not, in fact, a protein-based signal at all, but is instead constituted entirely of phospholipid. Glycophospholipid (or GPI)-linked proteins are synthesized as transmembrane polypeptides that are co-translationally inserted into the membrane of the RER. While still associated with the ER, the GPI-linked protein’s ectodomain is proteolytically removed and transferred to a preassembled structure composed of a complex glycan tethered to the membrane through its attachment to a molecule of phospholipid (frequently phosphotidylinositol). Previous work has shown that in polarized epithelial cells, most of the GPI-linked proteins reside in the apical plasmalemma. Interestingly, the apical surface also plays host to most of the cell’s complement of glycolipid. Investigators prepared a construct in which the VSV G ectodomain was wedded to the transmembrane tail of Thy-1, which carries a signal for glycophospholipidation. The resultant GPI-linked G protein is sorted to the apical membrane. The results of these and related experiments have generally been interpreted to indicate that a strong apical sorting signal is embodied in some component of the GPI linkage itself. The transmembrane domains of several single-spanning apical membrane proteins appear to carry information important for apical targeting. The transmembrane domains of the influenza virus neuraminidase and HA proteins, for example, are sufficient to mediate sorting to the apical surface when they are included in constructs expressed by transfection in MDCK cells. As will be discussed below, the same mechanisms that are thought to be involved in recognizing the GPI tail as an apical sorting motif may also interpret signals embedded in transmembrane domains. Furthermore, transmembrane domain sorting signals may be important not only in the localization of single spanning membrane proteins, but may also determine the distributions of polytopic ion pumps such as the Na + /K + – and H + /K + -ATPases (see below). It should also be noted that the extracytoplasmic or ecto domains of several apical proteins appear to incorporate directional signals. Roth et al. have shown that the ectodomain of the influenza HA protein is sufficient to specify apical targeting. When a cDNA construct encoding an anchor-minus form of the HA protein, which lacks both the cytosolic and transmembrane segments, is expressed in polarized cells it is secreted exclusively into the apical medium compartment. This is also true well for the polymeric immunoglobulin receptor. These results suggest that a signal involved in apical sorting resides in the lumenal portion of the HA molecule, and that this signal remains interpretable when it is presented as a soluble protein or in association with portions of a basolateral membrane polypeptide. Finally, N-linked sugar groups, which are also present on the extracytoplasmic domains of membrane proteins, can in some circumstances contribute apical sorting information. It is logical to conclude from this discussion that machinery necessary to read and interpret this putative ectodomain apical sorting information must be exposed at the lumenal surface of the organellar compartments involved in the segregation and targeting of newly synthesized membrane proteins.
As discussed above in the section on sorting pathways, not all of the plasma membrane proteins expressed by polarized epithelial cells pursue a direct course to their sites of ultimate functional residence. The polymeric immunoglobulin receptor (pIgR) for example, when examined in its native liver or in transfected MDCK cells, travels first to the basolateral surface and subsequently to the apical pole. A number of studies have examined the contributions that various portions of the pIgR molecule may make to this complicated sorting behavior. Anchor-minus ectodomain constructs of the pIgR are secreted apically from transfected MDCK cells. Furthermore, deletion of the pIgR cytosolic tail results in a membrane protein that travels directly to the apical surface without ever appearing at the basolateral side. These observations have led to the suggestion that the ectodomain of the pIgR receptor contains an apical sorting signal, and that this protein’s cytosolic tail embodies information that is required for its initial appearance at the basolateral plasma membrane. Extensive mutational analysis reveals that a trio of amino acids in the sequence His, Arg, Val is primarily responsible for the vectorial targeting of the newly synthesized pIgR protein to the basolateral plasmalemma. This motif constitutes yet another addition to the growing collection of distinct amino acid sequences that can encode basolateral sorting.
During its tenure at the basolateral membrane, the pIgR’s cytosolic tail becomes phosphorylated on a serine residue. The phosphorylation event occurs both in liver and in transfected MDCK cells. Intriguing experiments demonstrated that the addition of this phosphate group acts as a switch that allows the apical sorting signal to predominate, and results in the protein’s transcytosis to the apical side. Site directed mutagenesis has been performed on the cDNA encoding the pIgR in order to convert the serine of interest into either an alanine or an aspartate residue. When expressed in MDCK cells, the wild-type, as well as the two mutant forms, are all initially targeted to the basolateral surface, and all three undergo endocytosis and recycling at similar rates. Interestingly, however, while the wild-type receptor undergoes fairly rapid transcytosis, the alanine form remains largely associated with the basolateral plasma membrane. In contrast, the aspartate form is transcytosed at a rate that exceeds that characteristic of the non-mutant form. These observations suggest that the negative charge associated with the phosphate and aspartate residues permits or activates the incorporation of the pIgR into transcytotic vesicles, and thus initiates the protein’s delivery to the apical surface. The mechanism through which this signal is detected and interpreted remains unclear.
The recognition and segregation of pIgR destined for transcytosis probably occurs in an endosome following internalization from the basolateral surface. The second sorting event involved in the targeting of the pIgR is thus almost certainly completed at a subcellular location distinct from the TGN. This behavior suggests that, once again, the sorting of apical from basolateral proteins need not occur exclusively on the exocytic pathway. The endosome or an endosome-related compartment appears competent to sense and act upon the sorting signals that are necessary for the pIgR’s apical localization. It remains to be determined whether signals detected in the endosome correspond to the same ectodomain-associated information that mediates the apical secretion of an anchor-minus form of the pIgR. The segregation of this secretory form to the apical pathway almost certainly occurs during its passage through the Golgi, and is not likely to involve elements of the endocytic apparatus.
Most ion transport proteins and receptors span the membrane several times, and many are composed of multiple subunits. Their intricate structures complicate the search for sorting signals and increase the likelihood that multiple independent or hierarchical signals might be present. This is clearly the case for the gastric H,K-ATPase. Acid secretion in the stomach is mediated by the gastric H + /K + -ATPase. This dimeric ion pump is stored within an intracellular population of membranous vesicles, known as tubulovesicular elements (TVEs), in gastric parietal cells. Stimulation of acid secretion by secretagogues induces the TVEs to fuse with the parietal cell apical plasma membrane, resulting in the formation of deeply invaginated secretory canaliculi rich in H + /K + -ATPase. The cessation of acid secretion involves the retrieval of the H + /K + -ATPase from the cell surface, and the regeneration of the TVE storage compartment. Both the α- and β-subunits of the H + /K + -ATPase belong to the large P-type ATPase gene family. Their closest cousins in this collection are the corresponding α- and β-subunits of the Na + /K + -ATPase. Interestingly, while the H + /K + -ATPase functions at the apical surface of gastric parietal epithelial cells, the Na + /K + -ATPase is restricted in its distribution to the basolateral plasmalemma, in this and most other epithelial cell types. The homology relating these ATPases has permitted the creation of chimeric ion pumps, whose subunits are composed of complementary portions of the H + /K + – and Na + /K + -ATPase α- and β-polypeptides. By expressing these constructs in cultured polarized epithelial cells it has been possible to determine the molecular domains of the ion pump subunit proteins that are responsible for their sorting. Through this analysis it has become clear that both the α- and β-subunit polypeptides of the H + /K + -ATPase contain molecular signals which can contribute to the targeting of the holo-enzyme. Expression of a large number of progressively more refined α-subunit chimeras reveals that an eight amino acid sequence within the α-subunit of the H + /K + -ATPase is sufficient to specify apical sorting. This domain is predicted to reside within a transmembrane helix, thus suggesting that protein–lipid or protein–protein interactions within the plane of the membrane are responsible for pump sorting.
The β-subunit of the H + /K + -ATPase contains a tyrosine-based sorting signal that functions to internalize the pump complex from the surface of the gastric parietal cell, and return it to an intracellular regulated storage compartment. This internalization is responsible for the cessation of gastric acid secretion following the removal of secretagogue stimulation. This was demonstrated by generating a transgenic mouse which expresses an H + /K + -ATPase β-subunit lacking this endocytosis signal. These animals are unable to re-internalize H + /K + -ATPase from the apical surfaces of their gastric parietal cells. Consequently, they produce elevated gastric acid secretion during the interdigestive period. Mice carrying the mutant β-subunit develop gastritis and gastric ulcerations, with histologic features that are essentially identical to those found in human disease. Examination of renal potassium clearance in these animals reveals that the same β-subunit sorting signal regulates active potassium resorption in the collecting tubule.
Several studies have begun to define other signals employed in the polarized sorting of polytopic membrane proteins. Recently, for example, a novel motif has been identified in the cytoplasmic tail of the seven-membrane-span receptor rhodopsin that mediates this protein’s apical sorting when it is expressed in MDCK cells. Another member of the seven transmembrane G protein-coupled receptor family, the P2Y2 receptor, manifests an apical sorting signal in one of its extracellular loops. Furthermore, studies of neurotransmitter re-uptake systems have demonstrated that the four members of the highly homologous GABA transporter gene family are differentially sorted in epithelial cells and in neurons. The GAT-1 and GAT-3 isoforms, which are restricted to axons when expressed endogenously or by transfection in neurons, are sorted to the apical membranes of epithelial cells. The GAT-2 and betaine transporters, which are 50–67% identical to GAT-1 and GAT-3, behave as basolateral proteins in epithelia, and are restricted to dendrites when expressed in neurons. Production of chimeric and deletion constructs have permitted the identification of very short amino acid sequences at the extreme C-terminal tails of these transporters which manifest targeting information. The nature of these sequences suggests that they may interact with polypeptides containing PDZ-type protein–protein interaction domains, raising the possibility that this association may play a direct role in the sorting of ion transport proteins. A similar PDZ-dependent mechanism also appears to mediate the apical trafficking of CFTR. The basolateral isoform of the Na,K,2Cl co-transporter, NKCC1, has recently been shown to be targeted to the basolateral surface by virtue of a di-leucine motif in its cytoplasmic C-terminal tail. The renal isoform of this protein, NKCC2, depends for its apical localization on a two non-contiguous stretch of amino acids that appear to collaborate to form a conformation-dependent signal in the context of the fully folded transporter’s C-terminal domain. The mechanism through which this non-contiguous conformational determinant is recognized by the cellular sorting machinery has yet to be determined.
Cell Type-Specific Sorting: Patterns
The message encoded within a membrane protein’s sorting signal is dependent not only upon its own specific biochemical composition, but also upon the cellular context in which it is expressed. Several examples of membrane proteins that are differentially targeted in distinct epithelial cell types have been documented. The vacuolar H + -ATPase, for example, accumulates at the apical surfaces of α-type intercalated cells, but at the basolateral plasmalemmas of β-type intercalated cells in the renal collecting duct. Similarly, the Na + /K + -ATPase is basolateral in most epithelia, but behaves as an apical protein in cells derived from the neural crest, such as choroid plexus and retinal pigment epithelium. Targeting of particular proteins or classes of proteins can also vary as a function of the differentiation states of epithelial cells. For example, the sorting of well-characterized polarity markers expressed in Drosophila via germ line transformation was followed in the developing Drosophila embryo. Human placental alkaline phosphatase (PLAP) is a glycosylphosphatidyl inositol (GPI)-linked protein that accumulates at the apical membranes of mammalian epithelial cells. A chimeric construct composed of the transmembrane and cytosolic portions of the vesicular stomatitis virus (VSV) G protein coupled to the ectodomain of PLAP has been found to behave as a basolateral protein when expressed in the MDCK cell system. The subcellular distributions of these proteins were examined in the epithelial tissues of transgenic Drosophila embryos which expressed these proteins under the control of a heat shock promoter. In the surface ectoderm both PLAP and PLAPG were restricted to the basolateral membranes throughout development. Internal epithelia derived from the surface ectoderm accumulated PLAP at their apical surfaces, while PLAPG retained its basolateral distribution. The redistribution of PLAP from the basolateral to the apical plasma membrane was found to be coincident with the invagination of the surface epithelium to form internal structures, suggesting that the sorting pathways which function in the epithelium of the Drosophila embryo are developmentally regulated.
In light of both the multiplicity of sorting signals presented in the preceding section, and the apparent potential for heterogeneity in their interpretation discussed above, it is natural to wonder whether any logic or consistency governs nature’s solution to the deceptively simple problem of apportioning proteins among two separate membrane domains. Upon further reflection, however, the complexity and degeneracy of the “sorting code” can be seen as a tremendous virtue. Two different epithelial cell types may need to target a given membrane protein to opposite surfaces of their respective plasma membranes in order to fulfill their unique physiologic functions. These same functions may also require, however, that other membrane proteins occupy the same surface distributions in both cellular contexts. Thus, while the sodium pump occupies the apical membranes of the cells of the choroid plexus and the basolateral membranes of renal epithelial cells, receptors for basement membrane components are present at the basolateral surfaces of both cell types. If only a single class of basolateral sorting signal and a single class of apical sorting signal existed, then it would not be possible for a cell to selectively alter the distribution of one set of plasmalemmal proteins, without simultaneously altering the distributions of the entire population of the plasma membrane. In order to target the sodium pump to the apical surface, choroid plexus epithelial cells would be forced to target basement membrane receptors there as well. This would obviously constitute a wasteful compromise. In order to endow each epithelial cell type with the capacity to select individualized complements of proteins for its apical and basolateral domains, a dizzying multitude of sorting signals has evolved. Cells can thus customize the distributions of proteins among their plasmalemmal domains, without the constraints that would be imposed by a limited number of sorting signals. According to this interpretation, sorting signals do not specify a specific destination such as apical or basolateral. Instead, they specify classes of proteins whose members are always sorted together. The membrane domain to which any one of these classes is sorted will depend upon the cellular context in which it is expressed, and will be determined by the idiosyncratic array of sorting machinery and pathways present in each individual epithelial cell type.
Sorting: Mechanisms
Extensive progress has been made in illuminating the mechanisms through which the sorting signals discussed above exert their effects and ensure the polarized delivery of newly synthesized plasma membrane proteins. The strong evidence for the existence of sorting signals leads quite naturally to the postulate that sorting receptors must exist that are capable both of recognizing these signals, and of transducing their messages to the relevant cellular machinery. Such receptors have, in fact, been demonstrated in the case of lysosomal enzyme sorting. Targeting of a newly synthesized hydrolase to the lysosome is mediated by the interaction between the enzyme’s mannose-6-phosphate (man-6-p) recognition marker, and one of two receptors which bind man-6-p bearing ligands in the Golgi and mediate their segregation to pre-lysosomal endosomes. Binding of newly synthesized lysosomal enzymes to the man-6-p receptors is pH-dependent. At the relatively neutral pH of the Golgi ligands are tightly bound, whereas in the acid environment of the pre-lysosomal endosome they are rapidly released. No such well-characterized receptor systems have yet emerged to explain the sorting behavior of secretory and membrane proteins in polarized cells. While sorting receptors for secretory proteins remain to be identified definitively, some progress has been made in understanding how such receptors might function. Lysosomotropic amines, such as NH 4 Cl and chloroquine, elevate the lumenal pH of acidic organelles. The resulting neutralization of acidic compartments can have profound effects on sorting. In the case of lysosomal enzyme targeting, addition of NH 4 Cl raises the pH of the pre-lysosomal endosome, and thus prevents the acid-dependent unbinding of newly synthesized hydrolases from the man-6-p receptor. In the continued presence of the drug, the Golgi becomes depleted of receptors available to complex with free ligand. Newly synthesized enzymes bearing the man-6-p recognition marker are thus secreted constitutively and by default. Experiments on cultured polarized epithelial cells suggest that a similar pH-dependent mechanism may function in the sorting of basolateral secretory proteins.
Laminin and heparan sulfate proteoglycan are constituents of epithelial basement membranes. Studies of MDCK cells grown on permeable filter supports revealed that both of these proteins are normally secreted predominantly into the basolateral medium compartment. When secretion from cells treated with NH 4 Cl was monitored, it was found that both proteins were released into both media compartments in roughly equal proportions. Removal of the drug reversed this effect and restored normal basolateral secretion. As mentioned above, studies have demonstrated that the secretory default pathway for MDCK cells – that is the route pursued by soluble proteins which lack any means of interacting with the cellular sorting machinery – is apical and basolateral. It appears, therefore, that targeting of these two basolateral secretory proteins requires the participation of an intracellular acidic compartment. Elevation of the lumenal pH of this compartment reversibly blocks laminin and HSPG sorting, and results in their apical and basolateral default secretion.
Although the nature of the dependence of this sorting event on acidic compartments remains unknown, it is interesting to speculate that a mechanism similar to that which functions in lysosomal enzyme sorting may also be involved in routing basolateral secretory proteins. In such a model, binding or unbinding of laminin and HSPG from a sorting receptor would require the participation of an acidic organellar pH. Confirmation of this hypothesis will await the identification of such a pH-dependent binding protein with affinity for these and other basolaterally targeted proteins. Finally, it is worth noting that the basolateral sorting of the Na + /K + -ATPase, and the apical sorting of the influenza HA protein and a complex of secretory polypeptides, occurs normally in the presence and absence of NH 4 C1. It would appear, therefore, that different mechanisms are brought to bear in ushering different classes of proteins to their sites of ultimate functional residence.
Tyrosine-Based Motifs and Adaptors
Recent studies suggest that several different classes of soluble proteins may regulate the subcellular distributions of proteins bearing tyrosine-based signals. Perhaps the best understood of these are the adaptins. The adaptins comprise a group of peripheral membrane proteins that mediate the interaction between transmembrane proteins and the clathrin skeletons of coated pits and vesicles. Adaptins recognize and bind to tyrosine-containing coated pit localization sequences and link the proteins bearing these motifs to the clathrin coat. Adaptins can thus be considered to be among the most proximal elements of the endocytic sorting machinery – they recognize polypeptides endowed with endocytosis signals and ensure that they are incorporated into the specified internalization pathway. Distinct classes of adaptins function in the segregation of proteins into the coated structures associated with the trans-Golgi network, and into cell surface coated pits. While AP2 adaptors mediate internalization of proteins from the cell surface, AP1 adaptor complexes participate in trafficking proteins out of the TGN. The μ-subunits of adaptor complexes appear to be responsible for interacting with tyrosine-based motifs. Two isoforms of μ-subunits are found in AP1 complexes. The μ1a protein is ubiquitously expressed, and is found in both polarized and non-polar cell types. The μ1b protein is instead found in only a subset of polarized cell types. Proteins bearing tyrosine-based motifs are basolaterally sorted in MDCK cells, but accumulate apically in LLC-PK cells. It was noted that MDCK cells express μ1b, whereas this protein is absent from LLC-PK cells. Remarkably, expression of μ1b in LLC-PK cells at least partially “normalizes” their sorting properties, so that many (but not all) membrane proteins containing tyrosine-based signals are directed to the basolateral surface. Thus, μ1b constitutes perhaps the best characterized component of the sorting machinery. It is clearly capable of recognizing a class of sorting signals and acting upon the instructions that they convey. Consistent with the idea that clathrin adaptors play an important role in the sorting of at least some basolateral proteins, it has been demonstrated that knockdown of clathrin expression in cultured renal epithelial cells perturbs basolateral, but not apical, protein delivery.
It is interesting to note that recent studies demonstrate that different proteins bind to and interpret the messages encoded by tyrosine-based and di-leucine endocytosis motifs. Overexpression of tyrosine-motif containing proteins can inhibit the endocytosis of other proteins carrying a similar endocytosis signal, presumably by competing for limited quantities of the adaptor proteins that cluster proteins bearing these signals into clathrin-coated pits. This intervention does not affect, however, the internalization of proteins endowed with di-leucine motifs, indicating that they must be recognized and interpreted by a different class of polypeptides. It appears that the β-subunits of adaptor complexes interact with di-leucine motifs. Finally, a very different type of protein has been shown to interact with a tyrosine-based proline-rich sequence in the C-terminal tails of epithelial sodium channel (ENaC) subunits. The Nedd-4 protein possesses a ubiquitin ligase domain, and through its interaction with the ENaC tails may lead to these channels’ downregulation through degradation.
The association of basolateral membrane proteins, such as the Na + /K + -ATPase with elements of the subcortical cytoskeleton, has led to the speculation that this interaction may play a role in targeting. Evidence in support of this proposition was found in the studies, described above, which suggested that, at least in one MDCK cell clone, the Na + /K + -ATPase may be delivered in equal proportions to the apical and basolateral surface. Apically delivered material may be rapidly degraded, whereas basolateral sodium pump would be stabilized through interaction with the cytoskeleton and consequently would turn over very slowly. The pump’s polarized distribution would thus be the product of differential susceptibility to degradation, rather than sorting at the level of the Golgi. The degree to which stabilization through interaction with the cytoskeleton contributes to the polarized distribution of the sodium pump or any other proteins remains to be established.
Glycosphingolipid-Rich Membrane Domains
The observation that all of the glycolipids and GPI-linked proteins associated with epithelial cells tend to be found in the apical plasmalemmal domain led to the proposal that lipids may play a role in membrane protein sorting. Since glycolipids and GPI-linked proteins are only associated with the outer leaflet of the plasma membrane, these molecules will be exposed at the lumenal face of the organelles of the biosynthetic pathway. Any sorting machinery that interacts with glycolipids, therefore, must do so either at the lumenal surface or within the plane of the membrane itself. These constraints have suggested to some investigators the possibility that lipid–lipid interactions are sufficient to segregate apically directed glycolipids and GPI-linked proteins into distinct patches during their residence in the Golgi. These self-assembled patches could then serve as the nuclei from which apically directed vesicles would bud. The biophysical properties of these patches might be involved in ensnaring other apically directed proteins, as well as the components necessary to appropriately target the resultant transit vesicle. While evidence of lipid patches exists in both in vitro and in vivo systems, their precise role in the sorting process remains to be elucidated. Independent of its applicability, however, this model is extremely interesting. It is a useful reminder that forces other than simple receptor–ligand interactions are likely to be involved in generating and maintaining the anisotropic protein distributions that define the polarized state.
As noted above, several proteins are targeted to the apical membrane by virtue of signals embedded within their transmembrane domains. The fact that the amino acid residues of a transmembrane domain may be in direct contact with lipid molecules suggests the possibility that they may mediate apical sorting through interactions with glycosphingolipid-rich membrane domains. According to this hypothesis, the composition of its transmembrane domain may permit a protein to partition into glycosphingolipid rich patches, and thus to become concentrated in a region of the membrane that will give rise to an apically directed transport vesicle. GPI-linked proteins which have become associated with glycosphingolipid-rich membrane domains are insoluble in 1% TritonX-100 at 4°C. When a cell lysate prepared in this fashion is fractionated on a sucrose gradient, insoluble proteins are found near the top of the gradient, whereas soluble proteins remain in the heavier fractions. Interestingly, the transmembrane domain of the apical protein influenza neuraminidase carries apical sorting information, and also enables the protein to incorporate into insoluble material.
PDZ Domain-containing Proteins
As discussed above, the C-terminus of GABA transporter GAT-3 appears to be important for its apical localization in MDCK cells. The final residues of this C-terminal tail, threonine, histidine, and phenylalanine (THF), are reminiscent of the sequences present at the extreme C-terminal tails of proteins known to associate with members of the membrane-associated guanylate kinase (MAGUK) family. The MAGUK proteins incorporate one or more copies of the PDZ domain, which is named for three of the proteins in which the sequence homology defining this protein–protein interaction motif were first identified; PSD-95/SAP90, Dlg, and ZO-1. Interactions between the PDZ domain of a MAGUK protein and the extreme cytoplasmic tail of an integral membrane polypeptide appear to be important in organizing the surface distributions of intrinsic membrane proteins.
Observations obtained from a number of experimental systems provide further evidence for the involvement of PDZ domain-containing polypeptides in epithelial membrane protein sorting. The LET-23 receptor tyrosine kinase is localized to the basolateral cell surfaces of vulvar epithelial cells in C. elegans . Genetic studies reveal that at least three proteins contribute to the generation or maintenance of this distribution. Mutation of the lin2, lin7 or lin10 genes leads to loss of LET-23 basolateral polarity. Each of the proteins encoded by these genes includes one or more PDZ domains. A mutation in the Drosophila discs lost protein, which contains multiple PDZ domains, also leads to the mis-localization of several apical and basolateral proteins in the epithelial structures of affected embryos. It would appear, therefore, that PDZ domain-containing proteins may play a direct role in the polarized sorting of at least some membrane proteins or may be required for the generation or definition of polarized domains. These observations may be especially relevant to physiologic function of polarized renal epithelial cells, since a number of important ion transport proteins, including CFTR and NHE3, appear to interact with cytoplasmic proteins containing PDZ domains. It seems likely that these interactions may play a role in establishing these proteins distributions, and hence in determining their capacity to participate in vectorial ion transport.
Finally, it is important to note that once proteins have been sorted into the vesicles that will carry them to the appropriate cell surface domain, these vesicles themselves need to be targeted appropriately. Presumably, the vesicular membranes include proteins that ensure that the vesicles will interact and fuse with only the proper domain of the epithelial plasmalemma. This recognition machinery is likely to include components of the membrane fusion machinery, such as vesicular SNARE (soluble NSF attachment receptor) proteins. SNARE proteins present in both vesicular and target membranes form complexes that appear to be necessary for most normal cellular fusion processes. The extent to which different members of the SNARE family impart specificity to intracellular vesicular fusion events remains to be established. Interestingly, however, a component of the machinery involved in vesicular targeting in yeast was identified in mammalian cells. This Sec 6/8 “exocyst” complex appears to play a role specifically in the fusion of basolaterally-directed, but not apically-directed, post-Golgi carrier vesicles in epithelial cells. It is likely that the number of “destination-specific” vesicular and plasma membrane proteins important for directing vesicular traffic in polarized cells will continue to grow.
Epithelial Cell Polarity and Renal Disease
Because kidney function is dependent on the polarity of tubular epithelial cells, any condition that compromises this polarity will lead to renal failure. In general, this may occur through neoplastic processes, cell injury due to ischemia or nephrotoxicity, or through inherited genetic effects. Each of these may affect the tubular epithelial cells, their surrounding environment including the basal lamina and interstitial compartment, or both.
Carcinogenesis
During neoplastic growth it can be appreciated on the basis of morphology alone that the changes in cell and tissue organization wrought by tumorigenesis are likely to affect cell polarity. Model studies confirm this suspicion. When MDCK cells, which are not normally tumorigenic, are oncogenically transformed by introduction of the v-Ki- ras oncogene, they are converted from a simple epithelium to a multilayer, with great heterogeneity in overall cell morphology. Ultrastructural examination of these cells suggests that apical–basal polarity is severely compromised. Microvilli are diminished from the cells at the top layer, and organization of the cytoplasm is scrambled. Golgi complexes and centrosomes, which normally reside in an apical supranuclear location, are now randomly positioned. Despite this apparent high degree of disorganization, immunocytochemical localization of specific antigens and physiological measurements suggests that polarity is not totally disrupted. Basolateral proteins, including Na + /K + -ATPase and the cell-adhesion molecule E-cadherin, are restricted to regions of cell–cell contact, as in normal polarized MDCK cells. Apical proteins, on the other hand, are randomly localized to the free surface of the multilayered epithelia, as well as to areas of cell–cell contact in cells throughout the multilayer. The tight junctional antigen ZO-1 is found typically at the point where the free and adherent surfaces of the uppermost cell layer meet, as well as at a number of sites within the multilayer. The latter may be intercellular lumina or canaliculi connected to the upper surface. This localization probably reflects the presence of functional tight junctions, because the multilayer is both electrically tight and impermeant to inulin.
It is interesting to note that recent studies demonstrate that proteins encoded by tumor suppressor genes may function as key regulators of polarity. Mutations in the von Hippel–Lindau tumor suppressor gene can lead to renal cell carcinoma. While the primary function of the von Hippel–Lindau gene product (VHL) relates to the negative regulation of the activity of the hypoxia inducible factor (HIF) transcription factor, the VHL protein also appears to participate in the formation or stabilization of intercellular adhesive junctions. Mutations in the gene encoding the LKB1 protein kinase are responsible for Peutz–Jaeger syndrome, an inherited form of tumor susceptibility associated with the development of numerous hamartomas. Epithelial cells expressing LKB1 that is constitutively activated are able to form polarized domains in the absence of cell–cell and cell–substratum contact. Thus, proteins that participate in epithelial polarization may function as tumor suppressors by virtue of their capacity to control the growth and morphogenesis of the cells in which they are expressed.
Ischemic Injury
Other alterations in cell polarity may come about through the effect of renal ischemia on the tubular epithelium. Ischemic episodes of less than 1 hour often do not lead to tubular necrosis but may, nevertheless, cause diminished sodium and water uptake by the proximal tubule. Such brief ischemia compromises the polarity of tubular cells, resulting in the redistribution of a fraction of the Na + /K + -ATPase from the basolateral domain to the apical domain, preventing net sodium uptake by the tubule. At the same time, leucine aminopeptidase moves from the apical to the basolateral domain, and also becomes intracellular, presumably through endocytosis . At later times, Na + /K + -ATPase and leucine aminopeptidase are randomly distributed on the plasma membrane of tubular epithelial cells, remaining attached to the basement membrane or exfoliated into the lumenal space. The mechanism leading to this loss of polarity is not known. It is possible that ischemia, which is known to affect mitochondria and other organelles and to possibly alter the permeability of the plasma membrane, may result in increased cytoplasmic calcium concentrations. This, in turn, could disrupt elements of the cytoskeleton, perhaps affecting the maintenance of polarity. These may represent disruption or perturbation of the cortical actin cytoskeleton. In fact, in vitro studies with renal epithelial cell lines demonstrate that ATP depletion causes disassembly of the cytoskeleton and redistribution of actin from its normal locations in the cell cortex, terminal web, and microvilli to perinuclear cytoplasmic aggregates. In addition, energy depletion can lead to the endocytic internalization and proteolytic cleavage of cell adhesion molecules such as E-cadherin. Such alterations might affect transduction of spatial signals from the extracellular matrix to the polarization machinery along the lines previously discussed.
During reperfusion following renal ischemia, tubular epithelial cells detach from the basement membrane and accumulate in the lumen. It has been postulated that ischemia-induced depolarization of integrins from basal to apical domains of the plasma membrane contributes not only to cell detachment, but also to cell aggregation and tubular obstruction. According to this hypothesis, at early times post-ischemia, redistribution of integrins would loosen attachment of cells from the basal lamina, allowing some of them to detach. Released cells would then aggregate and adhere to remaining tubular epithelial cells via their integrins. These would either bind directly to each other by homotypic interactions or associate through bridging matrix molecules. Collections of such aggregates would obstruct the tubules, causing oliguria and destruction of renal tissue. In support of this hypothesis, integrins were observed to redistribute apically in oxidatively injured epithelial cell lines. Even more compelling was the observation that infusion of RGD peptides, which block some integrin–matrix interactions, appeared to ameliorate the effects of ischemia-induced by clamping of the renal artery. More recent in vivo findings utilizing a rat model of renal ischemia do not, however, support this hypothesis, at least with regard to β1 integrins. Soon after reperfusion, β1 integrins redistributed from a strictly basal to basolateral location in cells of the S3 segment of the proximal tubule, but did not appear on the apical plasma membrane at this time. Surprisingly, β1 integrins could not be detected by immunofluorescence in cells released from the basal lamina into the tubular lumen, precluding the possibility that they were mediating either cell aggregation or attachment of exfoliated cells to the residual tubular epithelium. Apical β1 integrins only appeared at later times post-ischemia, as cells lost polarity in the process of regeneration.
Genetic Diseases of Proximal Tubule Apical Endocytosis: Dent’s Disease and the Oculo Cerebral Renal Syndrome of Lowe
It is interesting to note that at least two genetic diseases with overlapping constellations of symptoms lead to perturbations of the proximal tubule’s megalin-mediated scavenging activity that normally prevents the urinary loss of low molecular weight filtered proteins. Dent’s disease is caused by inactivating mutations in the gene encoding the ClC5 protein, which functions as a chloride:proton exchanger in the membranes of proximal tubule epithelial cell endosomes. ClC5 activity leads to the accumulation of chloride ions in these endosomes, which appears to be required for steps in the internalization or recycling of the proximal tubule endocytic machinery. The Oculo Cerebral Renal Syndrome of Lowe is caused by mutations in an inositol lipid phosphatase that participates in controlling the inventory of inositol phospholipids in subcellular membranes. These inositol phospholipids help to establish the compartmental identity of subcellular membranes, and to facilitate the assembly of trafficking machinery on their cytosolic surfaces. While the precise mechanisms through which intra-endosomal chloride concentrations and intramembranous inositol phospholipid levels participate in the process of megalin endocytosis remain to be elucidated, it is clear that both of these parameters collaborate in generating or maintaining the proximal tubule epithelial cell’s unusual active apical endocytic machinery.
Polycystic Kidney Disease
The progressive formation of renal cysts, which characterizes autosomal dominant polycystic kidney disease (ADPKD), has also been suggested to occur as a result of polarity defects. ADPKD is the most common potentially lethal dominant genetic human disease. Approximately 85% of all cases are linked to mutations in the PKDl gene, with another 10% linked to PKD2. While the specific functions of the proteins encoded by these genes are the focus of intense study, the behavior of cyst epithelial cells in situ and in culture is consistent with a role for the PKD proteins in directing epithelial differentiation. Whereas renal tubular epithelial cells normally mediate fluid and electrolyte absorption, cyst epithelial cells carry out net secretion. It has been suggested that the proximal cause of renal cyst formation in polycystic kidney disease may be the mis-targeting of Na + /K + -ATPase to the apical plasmalemma.
According to this model, the presence of sodium pump at the apical surface leads to active apical ion secretion and the accumulation of lumenal cyst fluid. Other studies suggest that mis-localization of Na + /K + -ATPase cannot be the primary driving force for cyst fluid accumulation. When examined in cyst cells in culture or in situ , the Na + /K + -ATPase was found to be exclusively basolateral. Instead, the secretion appears to be driven by intracellular chloride accumulation via a basolateral Na + /K/ + 2Cl − co-transporter and apical chloride exit through the CFTR protein. A similar mechanism is responsible for fluid secretion by the poorly differentiated epithelial cells lining the crypts of the small intestine. As these crypt cells migrate up the intestinal villus they mature functionally, metamorphosing from secretory into resorptive epithelial cells. It has been suggested that the secretory phenotype is characteristic of immature epithelial cells, while more highly developed epithelial cells acquire the capacity to absorb fluid and electrolytes. The physiologic similarities relating cyst and crypt epithelial cells has prompted the hypothesis that loss of appropriate PKD function results in the dedifferentiation of mature resorptive renal tubular epithelial cells into more primitive secretory cells. The precise mechanisms through the PKDl and PKD2 mutations produce the dramatic pathology associated with ADPKD, and the potential role of epithelial differentiation and sorting pathways, remain to be determined.
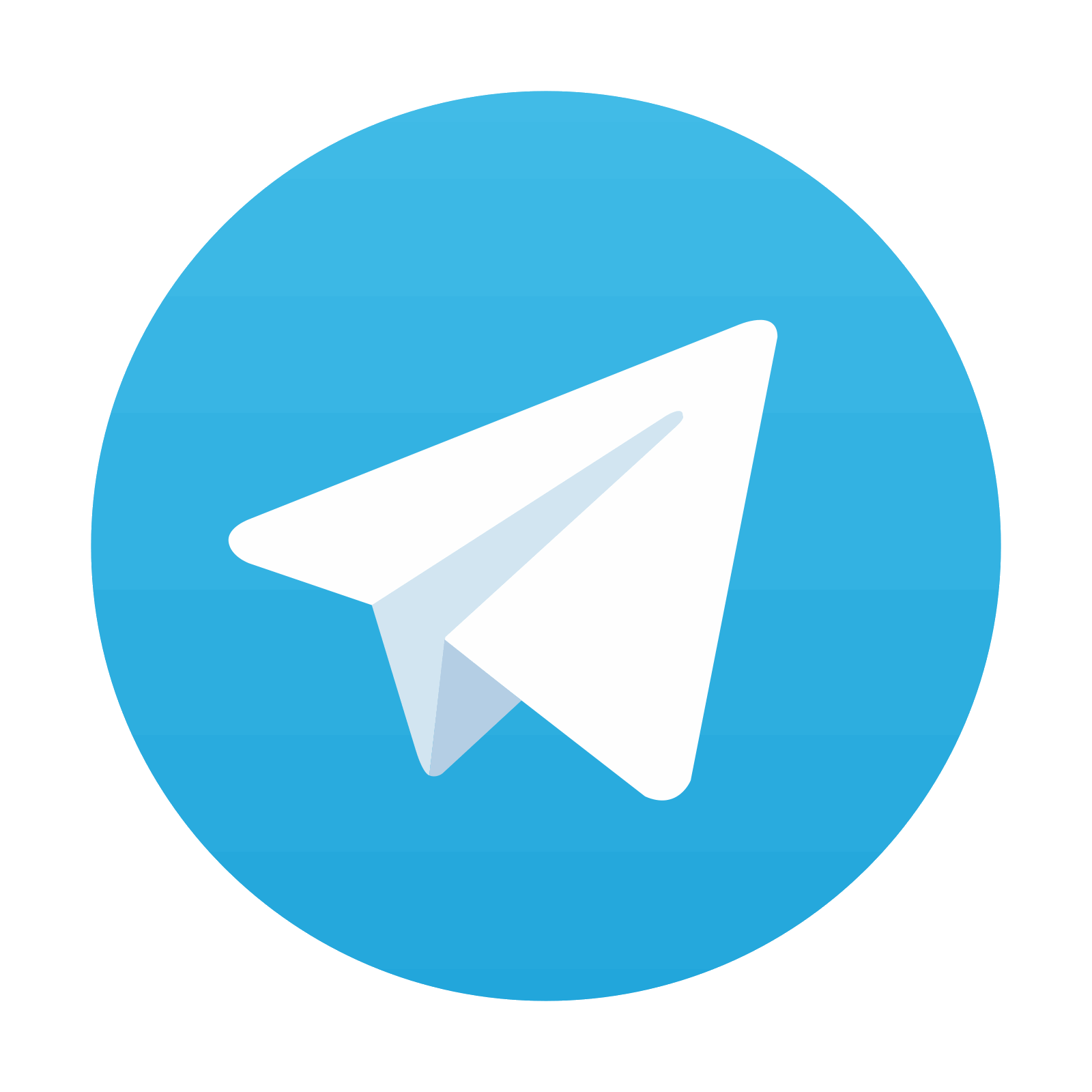
Stay updated, free articles. Join our Telegram channel
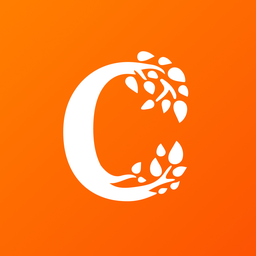
Full access? Get Clinical Tree
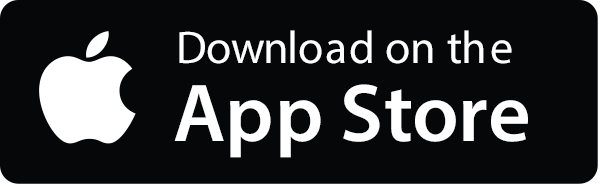
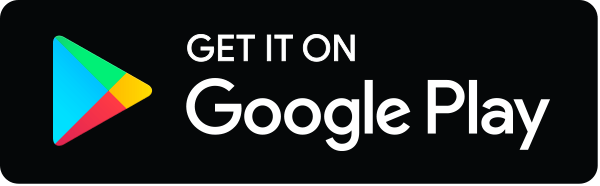