Chapter Outline
MAMMALIAN KIDNEY DEVELOPMENT: EMBRYOLOGY , 2
Development of the Urogenital System, 2
Development of the Metanephros, 3
Development of the Nephron, 4
The Nephrogenic Zone, 5
Branching Morphogenesis: Development of the Collecting System, 5
Renal Stroma and Interstitial Populations, 5
Development of the Vasculature, 6
MODEL SYSTEMS TO STUDY KIDNEY DEVELOPMENT , 6
Organ Culture, 6
Transgenic and Knockout Mouse Models, 9
Imaging and Lineage Tracing Studies, 19
Nonmammalian Model Systems for Kidney Development, 19
GENETIC ANALYSIS OF MAMMALIAN KIDNEY DEVELOPMENT , 21
Interaction of the Ureteric Bud and the Metanephric Mesenchyme, 21
Formation of the Collecting System, 25
Positioning of the Ureteric Bud, 27
Molecular Analysis of the Nephrogenic Zone, 28
Molecular Biology of Nephron Development: Tubulogenesis, 30
Molecular Genetics of the Stromal Cell Lineage, 31
Molecular Genetics of Vascular Formation, 32
The Juxtaglomerular Apparatus and the Renin Angiotensin Aldosterone System, 35
Nephron Development and Glomerulogenesis, 36
Maturation of Glomerular Endothelial Cells and Glomerular Basement Membrane, 39
Over the past several decades, the identification of genes and molecular pathways required for normal kidney development has provided insight into our understanding of obvious developmental diseases such as renal agenesis and renal dysplasia. However, many of the genes identified have also been shown to play roles in adult-onset and acquired kidney diseases such as focal segmental glomerulosclerosis. The number of nephrons present in the kidney at birth, which is determined during fetal life, predicts the risk of kidney disease and hypertension later in life; a lower number is associated with greater risk. Discovery of novel therapeutic targets and strategies to slow and reverse kidney diseases requires an understanding of the molecular mechanisms that underlie kidney development.
Mammalian Kidney Development: Embryology
Development of the Urogenital System
The vertebrate kidney derives from the intermediate mesoderm of the urogenital ridge, a structure found along the posterior wall of the abdomen in the developing fetus. It develops in three successive stages known as the pronephros, the mesonephros, and the metanephros ( Figure 1.1 ), although only the metanephros gives rise to the definitive adult kidney. However, earlier stages are required for development of other organs, such as the adrenal gland and gonad, that also develop within the urogenital ridge. Furthermore, many of the signaling pathways and genes that play important roles in the metanephric kidney appear to play parallel roles during earlier stages of renal development, in the pronephros and mesonephros. The pronephros consists of pronephric tubules and the pronephric duct (also known as the precursor to the wolffian duct) and develops from the rostralmost region of the urogenital ridge at 22 days of gestation (humans) and 8 days post coitum (dpc; mouse). It functions in the larval stages of amphibians and fish, but not in mammals. The mesonephros develops caudal to the pronephric tubules in the midsection of the urogenital ridge. The mesonephros becomes the functional excretory apparatus in lower vertebrates and may perform a filtering function during embryonic life in mammals. However, it largely degenerates before birth. Prior to its degeneration, endothelial, peritubular myoid, and steroidogenic cells from the mesonephros migrate into the adjacent adrenogonadal primordia, which ultimately form the adrenal gland and gonads. Abnormal mesonephric migration leads to gonadal dysgenesis, a fact that underscores the intricate association between these organ systems during development and explains the common association of gonadal and renal defects in congenital syndromes. In males, production of testosterone also induces the formation of seminal vesicles, tubules of the epididymis, and portions of the vas deferens from the wolffian duct.

Development of the Metanephros
The metanephros, the third and final stage, gives rise to the definitive adult kidney of higher vertebrates; it results from a series of reciprocal inductive interactions that occur between the metanephric mesenchyme (MM) and the epithelial ureteric bud (UB) at the caudal end of the urogenital ridge. The UB is first visible as an outgrowth at the distal end of the wolffian duct at approximately 5 weeks of gestation in humans or 10.5 dpc in mice. The MM becomes histologically distinct from the surrounding mesenchyme and is found adjacent to the UB. Upon invasion of the MM by the UB, signals from the MM cause the UB to branch into a T-tubule (at around 11.5 dpc in mice) and then to undergo iterative dichotomous branching, giving rise to the urinary collecting duct system ( Figure 1.2 ). Simultaneously, the UB sends reciprocal signals to the MM, which is induced to condense along the surface of the bud. Following condensation, a subset of MM cells aggregates adjacent and inferior to the tips of the branching UB. These collections of cells, known as pretubular aggregates, undergo mesenchymal-to-epithelial conversion to become the renal vesicle ( Figure 1.3 ).


Development of the Nephron
The renal vesicle undergoes patterned segmentation and proceeds through a series of morphologic changes to form the glomerulus and components of the nephrogenic tubules from the proximal convoluted tubule, the loop of Henle, and the distal tubule. The renal vesicles undergo differentiation, passing through morphologically distinct stages starting from the comma-shaped body and proceeding to the S-shaped body, capillary loop, and mature stage, each step involving precise proximal-to-distal patterning and structural transformations (see Figure 1.3 ). Remarkably, this process is repeated 600,000 to 1 million times in each developing human kidney as new nephrons are sequentially born at the tips of the UB throughout fetal life.
The glomerulus develops from the most proximal end of the renal vesicle that is farthest from the UB tip. Distinct cell types of the glomerulus can first be identified in the S-shaped body stage, in which presumptive podocytes appear as a columnar epithelial cell layer. A vascular cleft develops and separates the presumptive podocyte layer from more distal cells that will form the proximal tubule. Parietal epithelial cells differentiate and flatten to form Bowman’s capsule, a structure that surrounds the urinary space and is continuous with the proximal tubular epithelium. Concurrently, endothelial cells migrate into the vascular cleft. Together with podocytes, the endothelial cells produce the glomerular basement membrane (GBM), a major component of the mature filtration barrier. Initially the podocytes are connected by intercellular tight junctions at their apical surfaces. As glomerulogenesis proceeds, the podocytes revert to a mesenchymal-type phenotype, flatten, and spread out to cover the greater surface area of the growing glomerular capillary bed. They develop microtubule-based primary processes and actin-based secondary foot processes. During this time, the intercellular junctions become restricted to the basal aspect of each podocyte and eventually are replaced by a modified adherens junction–like structure known as the slit diaphragm (SD). At the same time, foot processes from adjacent podocytes become highly interdigitated. The SDs are signaling hubs serving as the final layer of the glomerular filtration barrier. Mesangial cell ingrowth follows the migration of endothelial cells and is required for development and patterning of the capillary loops that are found in normal glomeruli. The endothelial cells also flatten considerably, and capillary lumens are formed owing to apoptosis of a subset of endothelial cells. At the capillary loop stage, glomerular endothelial cells develop fenestrae, which are semipermeable transcellular pores common in capillary beds exposed to high hemodynamic flux. Positioning of the foot processes on the GBM and spreading of podocyte cell bodies are still incompletely understood but share many features of synapse formation and neuronal migration.
In the mature stage, glomerulus, the podocytes, fenestrated endothelial cells, and intervening GBM compose the filtration barrier that separates the urinary from the blood space. Together, these components provide a size- and charge-selective barrier that permits free passage of small solutes and water but prevents the loss of larger molecules such as proteins. The mesangial cells are found between the capillary loops (approximately three per loop); they are required to provide ongoing structural support to the capillaries and possess smooth muscle cell–like characteristics that give them the capacity to contract, which may account for the dynamic properties of the glomerulus. The tubular portion of the nephron becomes segmented in a proximal-to-distal order, into the proximal convoluted tubule, the descending and ascending loops of Henle, and the distal convoluted tubule. The distal tubule is contiguous with the collecting duct, a derivative of the UB. Imaging and fate mapping analysis reveal that this interconnection results from the invasion of the UB by cells from the distal segments of nascent nephrons (around the S-shaped body stage).
Although all segments of the nephron are present at birth and filtration occurs prior to birth, maturation of the tubule continues in the postnatal period. Increased expression levels of transporters, switch in transporter isoforms, alterations in paracellular transport mechanisms, and the development of permeability and biophysical properties of tubular membranes have all been observed to occur postnatally. Although additional studies are needed, these observations emphasize the importance of considering developmental stage of the nephron in interpretation of renal transport and may explain the age of onset of symptoms in inherited transport disorders; some of these issues may be recapitulated in acute kidney injury.
The Nephrogenic Zone
After the first few rounds of branching of the UB and the concomitant induction of nephrons from the MM, the kidney subdivides into an outer cortical region, where nephrons are being induced, and an inner medullary region, where the collecting system will form. As growth continues, successive groups of nephrons are induced at the peripheral regions of the kidney known as the nephrogenic zone ( Figure 1.4 ). Thus, within the developing kidney, the most mature nephrons are found in the innermost layers of the cortex, and the most immature nephrons in the most peripheral regions. At the extreme peripheral lining, under the renal capsule, a process that seems to recapitulate the induction of the original nephrons can be observed, whereby numerous UB-like structures are inducing areas of condensed mesenchyme. Indeed, whether there are significant molecular differences between the induction of the original nephrons and these subsequent inductive events is not known. A subpopulation of self-renewing mesenchymal cells immediately adjacent and inferior to the UB tips at the nephrogenic zone undergoes epithelial transformation, giving rise to new nephrons postnatally.

Branching Morphogenesis: Development of the Collecting System
The collecting system is composed of hundreds of tubules through which the filtrate produced by the nephrons is conducted out of the kidney and to the ureter and then the bladder. Water and salt resorption and excretion, ammonia transport, and H + secretion required for acid-base homeostasis also occur in the collecting ducts, under different regulatory mechanisms and using different transporters and channels from those that are active along tubular portions of the nephron. The collecting ducts are all derived from the original UB ( Figure 1.5 ). Whereas each nephron is an individual unit separately induced and originating from a distinct pretubular aggregate, the collecting ducts are the product of branching morphogenesis from the UB. Considerable remodeling is involved in forming collecting ducts from branches of UB, and how this occurs remains incompletely understood. The branching is highly patterned; the first several rounds are somewhat symmetric, additional rounds of branching are asymmetric, in which a main trunk of the collecting duct continues to extend toward the nephrogenic zone but smaller buds branch as they induce new nephrons within the nephrogenic zone. Originally, the UB derivatives are branching within a surrounding mesenchyme. Ultimately, they form a funnel-shaped structure in which cone-shaped groupings of ducts or papillae sit within a funnel or calyx that drains into the ureter. The mouse kidney has a single papilla and calyx, but a human kidney has 8 to 10 papillae, each of which drains into a minor calyx, with several minor calyces draining into a smaller number of major calyces.

Renal Stroma and Interstitial Populations
For decades in classic embryologic studies of kidney development, emphasis was placed on the reciprocal inductive signals between MM and UB. However, in later years, interest has arisen in the stromal cell as a key regulator of nephrogenesis. Stromal cells also derive from the MM but are not induced to condense by the UB. Two distinct populations of stromal cells have been described: Cortical stromal cells exist as a thin layer beneath the renal capsule and medullary stromal cells populate the interstitial space between the collecting ducts and tubules ( Figure 1.6 ). Cortical stromal cells also surround the condensates and provide signals required for UB branching and patterning of the developing kidney. Disruption or loss of these stromal cells leads to failure of UB branching, a reduction in nephron number, and disrupted patterning of nephric units with failure of cortical-medullary boundary formation. A reciprocal signaling loop from the UB exists to properly pattern stromal cell populations. Loss of these UB-derived signals leads to a buildup of stromal cells beneath the capsule that is several layers thick. As nephrogenesis proceeds, stromal cells differentiate into peritubular interstitial cells and pericytes that are required for vascular remodeling and for production of extracellular matrix responsible for proper nephric formation. These cells migrate from their positions around the condensates to areas between the developing nephrons within the medulla. Although stromal cells are derived from the MM cells, it remains unclear whether stromal cells and nephric lineages arise from a common progenitor MM cell.

Development of the Vasculature
The microcirculations of the kidney include the specialized glomerular capillary system responsible for production of the ultrafiltrate and the vasa recta, peritubular capillaries involved in the countercurrent mechanism. In the adult, each kidney receives 10% of the cardiac output. Vasculogenesis and angiogenesis have been described as two distinct processes in blood vessel formation. Vasculogenesis refers to de novo differentiation of previously nonvascular cells into structures that resemble capillary beds, whereas angiogenesis refers to sprouting from these early beds to form mature vessel structures including arteries, veins, and capillaries. Both processes are involved in development of the renal vasculature. At the time of UB invasion at 11 dpc (all timing given is for mice), the MM is avascular, but by 12 dpc a rich capillary network is present, and by 14 dpc vascularized glomeruli are present.
Transplantation experiments support a model whereby endothelial progenitors within the MM give rise to renal vessels in situ, although the origin of large blood vessels is still debated. At 13 dpc capillaries form networks around the developing nephric tubules, and by 14 dpc the hilar artery and first-order interlobar renal artery branches can be identified. These branches will form the corticomedullary arcades and the interlobular arteries that branch from them. Further branching produces the glomerular afferent arterioles. From 13.5 dpc onward, endothelial cells migrate into the vascular cleft of developing glomeruli, where they undergo differentiation to form the glomerular capillary loops ( Figure 1.7 ). The efferent arterioles carry blood away from the glomerulus to a system of fenestrated peritubular capillaries that are in close contact with the adjacent tubules and receive filtered water and solutes reabsorbed from the filtrate. These capillaries have few pericytes. In comparison, the vasa recta, which surround the medullary tubules and are involved in urinary concentration, are also fenestrated but have more pericytes. They arise from the efferent arterioles of deep glomeruli. The peritubular capillary system surrounding the proximal tubules is well developed in the late fetal period, whereas the vasa recta mature 1 to 3 weeks postnatally.

Model Systems to Study Kidney Development
Organ Culture
The Kidney Organ Culture System: Classic Studies
Metanephric kidney organ culture ( Figures 1.8 and 1.9 ) formed the basis for extensive classic studies of embryonic induction. Parameters of induction such as the temporal and physical constraints on exposure of the inductive tissue to the mesenchyme were determined, as were the times during which various tubular elements of the nephron were first observed in culture.


Mutant Phenotypic Analyses
As originally shown by Grobstein, Saxen, and their colleagues in classic studies of embryonic induction, the two major components of the metanephric kidney, the MM and the UB, could be separated from each other, and the isolated mesenchyme could be induced to form nephron-like tubules by a selected set of other embryonic tissues, the best example of which is embryonic neural tube. When neural tube is used to induce the separated mesenchyme, there is terminal differentiation of the mesenchyme into tubules, but not significant tissue expansion. In contrast, intact metanephric rudiments can grow more extensively, displaying both sustained UB branching and early induction of nephrons even when cultured for a week. The isolated mesenchyme experiment has proved useful in the analysis of renal agenesis phenotypes, in which there is no outgrowth of the UB. In these cases, the mesenchyme can be placed in contact with neural tube to determine whether it has the intrinsic ability to differentiate. Most often, when renal agenesis is due to the mutation of a transcription factor gene, tubular induction is not rescued by neural tube, as could be predicted for transcription factors which would be expected to act in a cell-autonomous fashion. In the converse situation, in which renal agenesis is caused by loss of a gene function in the UB (e.g., Emx2 in the mouse), it is usually possible for embryonic neural tube to induce tubule formation in isolated mesenchymes. Therefore, the organ culture induction assay can be used to test hypotheses concerning whether a particular gene is required in the UB or the MM. As chemical inhibitors specific for various signal transduction pathways have been synthesized and become available, it has been possible to add them to organ cultures and observe effects that are informative about the roles of specific pathways in development of the kidney. Examples are the uses of drugs to block the Erk/MAP kinase, PI3K/Akt, and Notch signaling pathways in renal explant cultures.
Antisense Oligonucleotides and siRNA in Organ Culture
Several studies have described the use of antisense oligonucleotides and of siRNA (small interfering, or silencing, RNA) molecules to inhibit gene expression in kidney organ cultures. Among the earliest of these was the inhibition of the low-affinity nerve growth factor receptor, p75 or NGFR, by antisense oligonucleotides, a treatment that decreased the growth of cultured embryonic kidneys. A subsequent study could not duplicate this phenotype, although there were possible differences in experimental techniques. An additional study using antisense oligonucleotides to Pax2 also showed this gene to be crucial in epithelialization of the MM. Antisense morpholinos modified with an octaguanidine dendrimer moiety to facilitate cell uptake have been used to target Wilms’ tumor-1 gene ( Wt1 ) in kidney explant cultures. This morpholino-based knockdown strategy allowed the identification of WT1 transcriptional targets in nephron progenitors, which was technically impossible in conventional Wt1 knockout mice because of renal agenesis. Co-transported with synthetic delivery peptides, antisense morpholinos have also been used to investigate the negative regulation of ureteric branching morphogenesis by semaphorin3a (Sema3a). Gene knockdown using siRNA has also been used to demonstrate the importance of Wt1 and Pax2 in nephrogenesis in whole organ and dissociated embryonic kidneys. Similar siRNA-based knockdown strategies have been successfully used to demonstrate the importance of fibronectin, Dact2, and estrogen-related receptor γ (Esrrg) in ureteric branching in whole embryonic renal explant cultures.
Organ Culture Microinjection
Microinjection in kidney explant cultures can be used to selectively target gene expression using a variety of reagents (plasmid constructs, viruses, and siRNA) in either the MM or the branching ureteric epithelia, depending on the site of injection. Retroviruses encoding mutants of polycystin-1 were used to demonstrate that polycystin-1 is required for normal ureteric branching patterns. Microinjection followed by electroporation of DNA plasmid constructs has been used to overexpress GDNF (glial cell–derived neurotrophic factor), Wt1, Pax2, Vegfa, and Robo2 in the MM and to assess the role of these genes in ureteric branching and early nephron induction.
Transgenic and Knockout Mouse Models
Over the past two decades, the generation and analysis of knockout and transgenic mice have provided tremendous insight into kidney development ( Table 1.1 ). Although homologous recombination to delete genes within the germline, also known as standard “knockout” technology, has provided information about the biologic functions of many genes in kidney development, it has several disadvantages. Disruption of gene function in embryonic stem (ES) cells may result in embryonic or perinatal death, precluding the functional analysis of the gene in the kidney that develops relatively late in fetal life. Additionally, many genes are expressed in multiple cell types, and the resulting knockout phenotypes can be complex and difficult or impossible to dissect. The ability to limit gene targeting to specific renal cell types overcomes some of these problems, and the temporal control of gene expression permits more precise characterization of a gene’s function. A number of mouse lines may be used to target specific kidney cell lineages ( Table 1.2 ; Figure 1.10 ). As with any experimental procedure, numerous caveats must be taken into account in the interpretation of data. These include determining the completeness of excision at the locus of interest, the timing and extrarenal expression of the promoters, and general toxicity of expressed proteins to the cell of interest. In spite of these issues, tissue-specific conditional gene targeting strategies remain powerful tools to study gene functions. The next generation of targeting includes improved efficiency using bacterial artificial chromosome (BAC) targeting approaches, siRNA and microRNA (miRNA) approaches, and large genomewide targeting efforts already under way at many academic and pharmaceutical institutions.
Gene Mutation or Knockout | Renal and Urogenital Tract Phenotypes, Other Tissues Affected | Associated Human Disease(s) | Reference(s) |
---|---|---|---|
Renal Aplasia (Variable) | |||
CTNNB1 (β-catenin) | Renal agenesis or severe renal hypoplasia, premature differentiation of UB epithelia (UB selective) | Colorectal cancer, hepatoblastoma, hepatocellular cancer | |
Emx2 | Complete absence of urogenital system | ||
Emx2, PAX2 | Duplicated kidneys and ureter, ureteral obstruction | CAKUT, VUR | |
Etv4, Etv5 | Renal agenesis or severe renal hypodysplasia | ||
EYA1 (Eyes absent-1) | Renal agenesis, lack of UB branching and mesenchymal condensation | Branchiootorenal syndrome (brachial fistulas, deafness) | |
Fgf9, Fgf20 | Renal agenesis | ||
Fgf10, GDNF, Gfra1 | Renal agenesis | ||
Fgfr1, Fgfr2 | Renal agenesis (MM selective) | ||
FRAS1, FREM1, FREM2 | UB failure, defect of GDNF expression | Fraser’s syndrome (cryptophthalmos, syndactyly, CAKUT); Manitoba-oculotrichoanal (MOTA) syndrome | |
GATA3 | Renal agenesis, gonad dysgenesis (null mutation); ectopic ureteric budding, kidney, hydroureter (UB selective) | Hypoparathyroidism, sensorineural deafness, and renal dysplasia (HDRS) syndrome; autoimmune disease (rheumatoid arthritis) | |
Gdf11 (growth differentiation factor 11) | UB failure, skeletal defects | ||
GDNF, Gfra1, RET | Renal agenesis or rudimentary kidneys, aganglionic megacolon | Hirschsprung disease, multiple endocrine neoplasm type IIA/B (MEN2A/MEN2B), and familial medullary thyroid carcinoma (FMTC) | |
GLI3 | Renal agenesis, severe renal agenesis, absence of renal medulla and papilla | Pallister-Hall (PH) syndrome (polydactyly, imperforate anus, abnormal kidneys, defects in the gastrointestinal tract, larynx, and epiglottis) | |
Grem1 (Gremlin) | Renal agenesis; apoptosis of the MM | ||
GRIP1 | Renal agenesis | Fraser’s syndrome | |
Hox-A11/D11 | Distal limbs, vas deferens | ||
Hs2st1 (heparan sulfate 2 O-sulfotransferase 1) | Lack of UB branching and mesenchymal condensation | ||
Isl1 (islet1) | Renal agenesis, renal hypoplasia, hydroureter (MM selective) | ||
ITGA8 (integrin α 8 ) | Renal agenesis, renal hypodysplasia | Fraser’s syndrome | |
Itgb1 (integrin β 1 ) | Disrupted UB branching, bilateral renal agenesis, hypoplastic collecting duct system (collecting duct selective); podocyte dedifferentiation (podocyte selective) | Fraser’s syndrome | |
Kif26b | Renal agenesis, failed UB attraction to the MM | ||
Lamc1 | UB failure, delayed nephrogenesis, water transport defects | ||
LHX1/LIM1 | Renal agenesis (null mutant); renal hypoplasia, UB branching defect, hydronephrosis, distal ureter obstruction (UB selective); arrested nephrogenesis, nephron patterning defects (MM selective) | Mayer-Rokitansky-Kuster-Hauser (MRKH) syndrome (müllerian duct agenesis) | |
LRP4 | Delayed UB induction, failed MM induction, syndactyly, oligodactyly | Cenani-Lenz syndrome | |
Npnt (nephronectin) | Delay of UB invasion into MM | ||
Osr1/Odd1 | Lack of MM, adrenal gland, gonads, defects in formation of pericardium and atrial septum | ||
PAX2 | Renal hypoplasia, VUR | CAKUT, VUR, optic nerve colobomas | |
PAX2, PAX8 | Defect in intermediate mesoderm transition, failure of pronephric duct formation | CAKUT, VUR, optic nerve colobomas | |
PTF1α (pancreas transcription factor 1α subunit/Danforth short-tail) | Failure of UB induction; anal atresia, persistent cloaca, skeletal malformation | Pancreatic and cerebellar agenesis; diabetes mellitus | |
Retinoic acid receptors ( Rara, Rarb2 ) | Renal hypoplasia, dysplasia, hydronephrosis, skeletal and multiple visceral abnormalities | ||
SALL1 | Renal agenesis, severe renal hypodysplasia | Townes-Brock syndrome (anal, renal, limb, ear anomalies) | |
SHH (Sonic hedgehog) | Bilateral or unilateral renal agenesis, unilateral ectopic dysplastic kidney, defective ureteral stromal differentiation | Vertebral defects, anal atresia, cardiac defects, tracheoesophageal fistula, renal anomalies, and limb abnormalities (VACTERL) syndrome | |
SIX1 | Lack of UB branching and mesenchymal condensation | Branchiootorenal syndrome | |
SOX8, SOX9 | Renal genesis, renal hypoplasia | Camptomelic dysplasia (limb and skeletal defects, abnormal gonad development) | |
WT1 | Renal and gonadal agenesis, severe lung, heart, spleen, adrenal, and mesothelial abnormalities | Wilms’ tumor, aniridia, genitourinary abnormalities, and retardation (WAGR) syndrome; Denys-Drash syndrome | |
Hypoplasia/Dysplasia/Low Nephron Mass | |||
Adamts1 | Hypoplasia of the renal medulla, hydronephrosis | ||
Adamts1, Adamts4 | Hypoplasia of the renal medulla, hydronephrosis | ||
Agtr2 (angiotensin II type-2 receptor) | Various collecting system defects | CAKUT | |
Ald1a2/Raldh2 (retinal dehydrogenase) | Renal hypoplasia, hydronephrosis, ectopic ureter | ||
BMP1RA/Alk3 | Hypoplasia of renal medulla, fewer UB branches (UB selective) | Juvenile polyposis syndrome | |
Bmp7 | Reduced MM survival | ||
Cdc42, Yap | Renal hypoplasia, oligonephronia, defects in mesenchyme to epithelial transition (CM selective) | ||
Cfl1, Dstn (cofilin1, destrin) | Renal hypodysplasia, ureter duplication | ||
CTNNB1 (β-catenin) | Severely hypoplastic kidney, lack of nephrogenic zone and S-shaped body (CM selective) | Colorectal cancer, hepatoblastoma | |
DICER1 | Renal hypoplasia, dysplasia, cysts (UB selective); renal hypoplasia characterized by premature termination of nephrogenesis (MM selective) | Pleopulmonary blastoma | |
Dkk1 (Dickkopf 1) | Overgrown renal papilla (renal tubule and collecting duct restricted) | ||
Dlg1, Cask | Renal hypoplasia and dysplasia, premature depletion of nephrogenic precursor cells | ||
Egfr (epidermal growth factor receptor) | Hypoplasia of the renal papilla, moderate polyuria, and urine concentration defects | ||
Esrrg | Agenesis of renal papilla | ||
Fat4 | Failed nephrogenesis (mesenchyme-to-epithelial transition), expansion of nephrogenic precursor zone (stroma selective) | ||
Fgf7 | Small kidneys, reduction in nephron number | ||
FGF8 | Renal dysplasia, arrested nephrogenesis at pretubular aggregate stage (MM selective) | Kallmann’s syndrome, hypogonadism | |
Fgf10 | Renal hypoplasia, multiorgan developmental defects including the lungs, limb, thyroid, pituitary, and salivary glands | ||
Fgfr1, Fgfr2 | Renal agenesis (MM selective) | ||
Fgfr2 | Renal hypoplasia, hydronephrosis (UB selective) | ||
FOXC2 | Renal hypoplasia | AD lymphedema-distichiasis syndrome | |
Foxd1 (BF-2) | Accumulation of undifferentiated CM, attenuated UB branching, stromal patterning defects | ||
Foxd1 | Mild renal hypoplasia (UB selective) | ||
Fzd4, Fzd8 (frizzled 4/8) | Impaired UB branching, renal hypoplasia | ||
LGR4 | Severe renal hypoplasia and oligonephronia; renal cysts | Aniridia–genitourinary anomalies–mental retardation syndrome | |
LMX1B | Renal dysplasia, skeletal abnormalities | Nail-patella syndrome | |
Mdm2 (murine double minute 2) | Renal hypoplasia and dysplasia, severely impaired UB branching and nephrogenesis (UB selective); depletion of nephrogenic precursors (MM selective) | ||
Mf2 | Renal hypoplasia, oligonephronia | ||
Pbx1 | Reduced UB branching, delayed mesenchyme-to-epithelial transformation, dysgenesis of adrenal gland and gonads | ||
Plxnb2 (plexin B2) | Renal hypoplasia and ureter duplication | ||
Pou3f3 ( Brn1 ) | Impaired development of distal tubules, loop of Henle, and macula densa; distal nephron patterning defect | ||
Prr (prorenin receptor) | Renal hypoplasia, renal dysplasia (UB selective) | ||
Psen1, Psen2 (presenilins 1/2) | Severe renal hypoplasia, severe defects in nephrogenesis | ||
Ptgs2 (prostaglandin endoperoxide synthase 2/cyclooxygenase-2) | Oligonephronia | ||
Rbpj | Severe renal hypoplasia, oligonephronia, loss of proximal nephron segments, tubular cysts (MM selective) | ||
Shp2 | Severe impairment of UB branching, renal hypoplasia | ||
Six1 | Hydronephrosis, hydroureter, abnormal development of ureteral smooth muscle | ||
Six2 | Renal hypoplasia, premature depletion of nephrogenic precursors | ||
Tbx18 | Hydronephrosis, hydroureter, abnormal development of ureteral smooth muscle | ||
Tfap2b | MM failure, craniofacial and skeletal defects | ||
TRPS1 | Impaired UB branching, renal hypoplasia | Trichorhinophalangeal syndrome (skeletal defects) | |
Wnt4 | Failure of MM induction | ||
Wnt7b | Complete absence of medulla and renal papilla (UB selective) | ||
Wnt9b | Vestigial kidney, failure of MM induction Cystic kidney (collecting duct selective) | ||
Wnt11 | Impaired ureteric branching, renal hypoplasia | ||
Mislocalized or Ectopic UB/Increased UB Branching | |||
Bmp4, Bmp7 | Ectopic UB, renal hypodysplasia, hydroureter, defective ureterovesical junction | ||
Cer1 | Increased ureteric branching, altered spatial organization of ureteric branches | ||
Foxc1 | Duplex kidneys, ectopic ureters, hydronephrosis, hydroureter | ||
HNF1B, PAX2 | Renal hypoplasia, duplex kidneys, ectopic ureters, megaureter, hydronephrosis | CAKUT | |
Lzts2 (leucine-zipper putative tumor suppressor 2) | Duplex kidneys/ureters, hydronephrosis, hydroureter | ||
Plxnb1 (plexin B1) | Increased ureteric branching | ||
Plxnb2 (plexin B2) | Renal hypoplasia and ureter duplication | ||
Sema3a | Increased ureteric branching (UB selective) | ||
Slit2, ROBO2 | Increased UB branching | CAKUT, VUR | |
Spry1 (sprouty 1) | Supernumerary UBs, multiple ureters | ||
Cysts | |||
Aqp11 (aquaporin-11) | Abnormal vacuolization of proximal tubules; polycystic kidneys | ||
Bcl2 | Renal hypoplasia and cysts | ||
Bicc1 | Polycystic kidneys | ||
Bpck/TMEM67 | Polycystic kidneys, hydrocephalus | Meckel’s syndrome (multicystic renal dysplasia, neural tube defects) | |
Erbb4 | Renal cysts (overexpression in renal tubules) Dilated and mispolarized tubules, increased renal fibrosis (renal tubule deletion) | ||
FAT4 | Renal cysts, disrupted hair cell organization in inner ear | Van Maldergem’s syndrome (mental retardation, abnormal craniofacial features, deafness, skeletal and limb malformations, renal hypoplasia) | |
GLIS3 | Polycystic kidney, neonatal diabetes | Congenital hypothyroidism, diabetes mellitus, hepatic fibrosis, congenital glaucoma | |
GPC3 (glypican-3) | Disorganized tubules and medullary cysts | Simpson-Golabi-Behmel syndrome | |
HNF1B | Polycystic kidney disease (tubular-selective) | Maturity-onset diabetes of the young type 5 (MODY5) | |
Ift88/Orpk (intraflagellar transport 88/Oak Ridge Polycystic Kidney Disease) | Polycystic kidneys; defective left-right asymmetric patterning | ||
Invs (inversin) | Polycystic kidneys, inverted viscera | ||
Kif3A | Polycystic kidney disease (tubular-selective) | ||
MAFB (Kreisler) | Decreased glomeruli, cysts, and tubular dysgenesis | Musculoaponeurotic fibrosarcoma | |
MKS1 | Renal hypoplasia and cysts | Meckel’s syndrome (multicystic renal dysplasia, neural tube defect) | |
PKD1, PKD2 | Renal cysts | ADPKD, ARPKD | |
PTEN | Abnormal ureteric bud branching, cysts (UB selective) | Cowden’s disease, Bannayan-Riley-Ruvalcaba syndrome, various tumors | |
Taz/Wwtr1 | Polycystic kidneys, emphysema | ||
VHL | Renal cysts (tubular-selective) | Von Hippel–Lindau syndrome | |
Xylt2 (xylosyltransferase 2) | Polycystic kidneys and liver | ||
Later Phenotypes (Glomerular, Vascular, and Glomerular Basement Membrane) | |||
ACE (angiotensin-converting enzyme) | Atrophy of renal papillae, vascular thickening and hypertrophy, perivascular inflammation | Chronic systemic hypotension | |
ACTN4 (α-actinin 4) | Glomerular developmental defects, FSGS | SRNS | |
AGT (angiotensinogen) | Atrophy of renal papillae, vascular thickening and hypertrophy, perivascular inflammation | Essential hypertension, renal tubular dysgenesis | |
AGTR1A (AT1A) | Hypertrophy of juxtaglomerular apparatus and expansion of renin cell progenitors, mesangial cell hypertrophy | Essential hypertension, renal tubular dysgenesis | |
AGTR1A, AGTR1B ( AT1A, AT1B ) | Atrophy of renal papillae, vascular thickening and hypertrophy, perivascular inflammation | Essential hypertension, renal tubular dysgenesis | |
AMPD (AMP [adenosine monophosphate] deaminase) | Podocyte foot process effacement, proteinuria | Minimal change nephrotic disease | |
Angpt1/ANG1 (angiopoietin 1) | Simplification and dilation of glomerular capillaries; detachment of glomerular endothelium from the GBM; loss of mesangial cells | ||
Angpt2/ANG2 (angiopoietin 2) | Cortical peritubular capillary abnormalities (null allele) Apoptosis of glomerular capillaries, proteinuria (transgenic overexpression) | ||
ARHGDIA/RhoGDIα | FSGS | SRNS | |
Bmp7 | Hypoplastic kidney, impaired maturation of nephron, reduced proximal tubules (podocyte selective) | ||
CD151 | Podocyte foot process effacement, disorganized GBM, tubular cystic dilation | Nephropathy (FSGS) associated with pretibial epidermolysis bullosa and deafness | |
CD2AP | Podocyte foot process effacement, immunotactoid nephropathy | FSGS | |
Cdc42 | Congenital nephrosis; impaired formation of podocyte foot processes (podocyte selective) | ||
Cmas | Congenital nephrosis; impaired formation of podocyte foot processes, defective sialylation | ||
COL4A1, COL4A3, COL4A4, COL4A5 | Disorganized GBM, proteinuria | Alport’s syndrome | |
Crk1/2, CrkL | Albuminuria, altered podocyte cytoarchitecture (podocyte selective) | ||
Cxcl12/SDF1 (stroma-derived factor 1), CXCR4, Cxcr7 | Petechial hemorrhage in the kidneys, glomerular aneurysm, fewer glomerular fenestrations, reduced mesangial cells, podocyte foot process effacement, mild renal hypoplasia | WHIM (warts, hypogammaglobulinemia, infections, and myelokathexis) syndrome | |
DICER1 | Podocyte damage, albuminuria, end-stage kidney failure (podocyte selective); reduced renin production, renal vascular abnormalities, striped fibrosis (renin cell selective) | Pleuropulmonary blastoma | |
Dnm1, Dnm2 (dynamin 1/2) | Podocyte foot process effacement and proteinuria (podocyte selective) | ||
EphB4 | Aberrant development of vascular shunts in glomerular arterioles (transgenic overexpression in renal tubules and parietal cells of Bowman’s capsule) | ||
Ephrin-B2 | Dilation of glomerular capillaries | ||
Fat1 | Foot process fusion, failure of foot process formation | ||
Flt1/VEGFR1 | Nephrotic syndrome | ||
Foxc2 | Impaired podocyte differentiation, dilated glomerular capillary loop, poor mesangial migration | ||
Foxi1 | Distal renal tubule acidosis; absence of collecting duct intercalated cells | ||
Fyn | Podocyte foot process effacement, abnormal slit diaphragms, proteinuria | ||
Gne/Mnk (glucosamine-2-epimerae/ N -acetylmannosamine kinase) | Hyposialylation defect, foot process effacement, GBM splitting, proteinuria and hematuria | ||
Ilk (integrin-like kinase) | Nephrotic syndrome (podocyte selective) | ||
INSR (insulin receptor) | Podocyte effacement, GBM alteration, proteinuria (podocyte selective) | Diabetic nephropathy | |
Itga3 (integrin α 3 ) | Reduced UB branching, glomerular defects, poor foot process formation | ||
Itgb1 (integrin β 1 ) | Podocyte loss, capillary and mesangial degeneration, glomerulosclerosis (podocyte selective) | ||
Kirrel (Neph1) | Abnormal slit diaphragm function, FSGS | ||
Lama5 | Defective glomerulogenesis, abnormal GBM, poor podocyte adhesion, loss of mesangial cells | ||
LAMB2 | Proteinuria prior to the onset of foot process effacement | Pierson’s syndrome | |
LMX1B | Impaired differentiation of podocytes, cytoskeletal disruption in podocytes | Nail-patella syndrome | |
Mafb (Kreisler) | Abnormal podocyte differentiation | ||
Mpv17 (mitochondrial inner membrane protein 17) | Nephrotic syndrome | ||
Mtor/mTOR (mechanistic target of rapamycin) | Proteinuria, podocyte autophagy defects (podocyte selective) | ||
MYO1E | Podocyte foot process effacement and proteinuria | SRNS | |
Nck1, Nck2 | Failure of foot process formation (podocyte selective) | ||
Nid1 (nidogen-1/entactin-1) | Abnormal GBM | ||
NPHS1 (nephrin) | Absent slit diaphragms, congenital nephrotic syndrome | Congenital nephrosis of the Finnish type, childhood-onset steroid-resistant nephritic syndrome, childhood- and adult-onset FSGS | |
NPHS2 (podocin) | Congenital nephrosis, FSGS, vascular defects | SRNS, congenital nephritic syndrome | |
NOTCH1, NOTCH2 | Lack of glomerular endothelial and mesangial cells (standard knockout) Lack of podocytes and proximal tubular cells (MM selective); impaired nephrogenesis (cap mesenchyme selective) | Alagille’s syndrome (cholestatic liver disease, cardiac disease, kidney dysplasia, renal cysts, renal tubular acidosis) | |
Pdgfb/PDGFR-β | Lack of mesangial cells, ballooned glomerular capillary loop | ||
Pik3c3/Vps34 | FSGS, defects in vesicular trafficking (podocyte selective) | ||
Prkci/aPKCλ/ι (atypical protein kinase C λ/ι) | Defect of podocyte foot processes, nephrotic syndrome (podocyte selective) | ||
PTPRO/GLEPP1 (glomerular epithelial protein phosphatase 1) | Broadened podocyte foot processes with altered interdigitation patterns | SRNS | |
Rab3A | Albuminuria, disorganization of podocyte foot process structure | ||
Rbpj | Decreased renal arterioles, absence of mesangial cells, and depletion of renin cells (stromal cell selective) Reduction in juxtaglomerular cells, impaired renin synthesis (renin cell selective) | ||
Rhpn1 (rhophilin-1) | FSGS, podocyte foot process effacement, GBM thickening | ||
ROBO2 | Abnormal pattern of podocyte foot process interdigitation, focal effacement of foot processes, proteinuria | CAKUT, VUR | |
SLC5A2/SGLT2 (sodium-glucose transporter 2) | Elevated urinary excretion of glucose, calcium, and magnesium | Glucosuria | |
Sh3gl1/2/3 (endophilin 1/2/3) | Podocyte foot process effacement and proteinuria, neuronal defects | ||
Sirpa/SIRPα | Irregular podocyte foot process interdigitation; mild proteinuria | ||
Sox4 | Oligonephronia, podocyte effacement, GBM defects (MM selective) | ||
SOX17, SOX18 | Vascular insufficiency in kidneys and liver; ischemic atrophy of renal and hepatic parenchyma; defective postnatal angiogenesis | HLT (hypotrichosis-lymphedema-telangiectasia) syndrome (hair, vascular and lymphatic disorder) | |
Synj1 (synaptojanin 1) | Podocyte foot process effacement and proteinuria; neuronal defects | ||
Tcf21 (Pod1/capsulin/epicardin) | Lung and cardiac defects, sex reversal and gonadal dysgenesis, vascular defects, disruption in UB branching, impaired podocyte differentiation, dilated glomerular capillary, poor mesangial migration | ||
Tie1 | Tie1 –null cells fail to contribute to the glomerular endothelium | ||
TRPC6 | Protected from angiotensin-mediated or proteinuria or complement-dependent glomerular injury (null mutation); podocyte foot process effacement and proteinuria (transgenic overexpression in the podocyte lineage) | SRNS, FSGS | |
Vegfa | Endotheliosis, disruption of glomerular filtration barrier formation, nephrotic syndrome (podocyte selective) | ||
VHL | Rapidly progressive glomerulonephritis (podocyte selective) | Von Hippel–Lindau syndrome |
Promoter | Renal Expression | Extrarenal Expression | Reference(s) |
---|---|---|---|
11Hsd2 (11β-hydroxysteroid dehydrogenase-2) | Principal cells of collecting duct, connecting tubules | Amygdala, cerebellum, colon, ovary, uterus, epididymis, salivary glands | |
Aqp2 (aquaporin-2) | Principal cells of collecting duct | Testis, vas deferens | |
Atp6v1b1 (V-ATPase-B1) | Collecting ducts (intercalated cells), connecting tubule | ||
Bmp7 | Cap mesenchyme | ||
Cdh16 /Ksp-cadherin | Renal tubules, collecting ducts, ureteric bud, wolffian duct, mesonephros | Müllerian duct | |
Cited1 | Cap mesenchyme | ||
Emx1 | Renal tubules (proximal and distal tubules) | Cerebral cortex, thymus | |
Foxd1/BF2 | Stromal cells | ||
Ggt1 (gamma-glutamyl transferase 1) | Cortical tubules | ||
HoxB6 | Metanephric mesenchyme | Lateral mesoderm, limb buds | |
HoxB7 | Ureteric bud, wolffian duct, collecting ducts, distal ureter | Spinal cord, dorsal root ganglia | |
Kap (kidney androgen regulated protein) | Proximal tubules | Brain | |
Klf3 | Collecting ducts | Gonads | |
Nphs1 (nephrin) | Podocytes | Brain | |
Nphs2 (podocin) | Podocytes | ||
Osr2 | Condensing metanephric mesenchyme; glomeruli | Palatal mesenchyme | |
Pax2 | Pronephric duct, wolffian duct, ureteric bud, cap mesenchyme | Inner ear, midbrain, cerebellum, olfactory bulb | |
Pax3 | Metanephric mesenchyme | Neural tube, neural crest | |
Pax8 | Renal tubules (proximal and distal tubules) and collecting ducts (Tet-On inducible system) | ||
Pdgfrb (PDGFR-β) | Mesangial cells, vascular smooth muscles | Pericytes, vascular smooth muscles | |
Pepck | Proximal tubules | Liver | |
Rarb2 | Metanephric mesenchyme | ||
Ren1 (Renin) | Juxtaglomerular cells, afferent arterioles, mesangial cells | Adrenal gland, testis, sympathetic ganglia, etc. | |
Ret | Ureteric bud, collecting ducts | Dorsal root ganglion, neural crest | |
Sall1 | Metanephric mesenchyme (tamoxifen-inducible system) | Limb buds, central nervous system, heart | |
Slc5a2 /SGLT2 (sodium-glucose transporter 2) | Proximal tubules | ||
Six2 | Cap mesenchyme | ||
Sox18 | Cortical and medullary vasculature | Blood vessel and precursor of lymphatic endothelial cells | |
Spink3 | Medullary tubules (distal or connecting tubules?) | Mesonephric tubules, pancreas, lung, liver, gastrointestinal tract | |
T (brachyury) | Whole kidney (both ureteric bud and metanephric mesenchyme) | Panmesodermal | |
Tcf21 (Pod1) | Metanephric mesenchyme, cap mesenchyme, podocytes, stromal cells | Epicardium, lung mesenchyme, gonad, spleen, adrenal gland | |
Umod (uromodulin/Tamm-Horsfall protein) | Thick ascending loops of Henle | Testis, brain | |
Wnt4 | Renal vesicles, nascent nephrons (comma- and S-shaped bodies) |

In contrast to gene targeting experiments in which the gene is known at the beginning of the experiment ( reverse genetics ), random mutagenesis represents a complimentary phenotype-driven approach ( forward genetics ) to study the physiologic relevance of certain genes. Random mutations are introduced into the genome at high efficiency by chemical or gene trap mutagenesis. Consecutively, large numbers of animals are screened systematically for specific phenotypes of interest. As soon as a phenotype is identified, test breeding is used to confirm the genetic nature of the trait. Chromosomal mapping and positional cloning are then used to determine the identity of the culprit mutant gene. There are two major advantages of genomewide approaches over reverse genetics. First, most knockouts lead to major gene disruptions, which may not be relevant to the subtle gene alterations that underlie human renal disease. Second, many of the complex traits underlying congenital anomalies and acquired diseases of the kidney are unknown, making predictions about the nature of the genes that are involved in these diseases difficult.
One of the most powerful and well-characterized mutagens in the mouse is the chemical mutagen N -ethyl- N -nitrosourea (ENU). It acts through random alkylation of nucleic acids, inducing point mutations in spermatogonial stem cells of injected male mice. ENU mutagenesis introduces multiple point mutations within the spermatogonia of the male, which is then bred to a female mouse of different genetic background. Resultant offspring are screened for renal phenotypes of interest (e.g., dysplastic, cystic) and heritability. Mutations may be complete or partial loss of function, gain of function, or altered function and can have either dominant or recessive effect. The specific locus mutation frequency of ENU is 1 in 1000. Assuming a total number of 25,000 to 40,000 genes in the mouse genome, a single treated male mouse should have between 25 and 40 different heterozygous mutagenized genes. In the case of multigenic phenotypes, segregation of the mutations in the next generation allows the researcher to focus on monogenic traits. In each generation, 50% of the mutations are lost, and only the mutation underlying the selected phenotype is maintained in the colony. A breeding strategy that includes backcrossing to the female genetic strain enables rapid mapping of the ENU mutation that occurred on the male genetic background.
The screening in ENU mutagenesis experiments can focus on dominant or recessive renal mutations. Screening for dominant phenotypes is popular because breeding schemes are simple and a great number of mutants can be recovered through this approach. About 2% of all first-generation offspring mice display a heritable phenotypic abnormality. One of the fruitful results obtained with this approach is the identification of a mutation in the aquaporin 11 gene (Aqp11) that causes severe proximal tubule injury and vacuolation of the renal cortex resulting in renal failure and perinatal death. It is possible to design “sensitized screens” on a smaller scale, thereby improving the ability to identify genes in a pathway of interest. For example, in renal glomerular development, the phenotype of a genetic mouse strain with a tendency to development of congenital nephrosis (e.g., CD2AP haploinsufficiency) may be enhanced or suppressed by breeding a female of the strain to a mutagenized male. The modifier gene may then be mapped using the approach outlined earlier. This approach has been successfully used to identify genes involved in neural development.
Other genomewide approaches that have led to the discovery of novel genes in kidney development and disease are gene trap consortia and genomewide transcriptome and proteome projects. The interested reader is referred to the websites for the Centre for Modeling Human Disease ( www.cmhd.ca ), the International Gene Trap Consortium ( www.genetrap.org ), Knockout Resources to Conquer Human Disease ( www.tigm.org ), and the Human Kidney & Urine Proteome Project ( www.hkupp.org ).
Imaging and Lineage Tracing Studies
Detailed imaging of renal structures and morphogenetic processes has benefited significantly from the availability and development of multiple fluorescent proteins. The advent of genetically modified mice that express fluorescent proteins revolutionized cell lineage and mapping studies allowing high-resolution live visualization of morphogenetic events both in situ and in cultured organ explants. Targeted labeling of cells with fluorescent proteins can be achieved by driving expression of fluorescent proteins under direct control of a cell-specific promoter. Alternatively, a Cre driver mouse can be crossed with a fluorescent reporter animal, whereby Cre recombinase (an enzyme that triggers swapping, or recombination, of stretches of DNA in chromosomes) turns on the constitutive expression of a fluorescent protein. This Cre-driven strategy is particularly valuable in cell lineage tracking and fate mapping analysis because both the progenitor and its subsequent derivatives become fluorescently labeled. A third method involves spatiotemporal induction of fluorescent protein expression, allowing for the fluorescence to be turned on or off through administration of doxycycline or tamoxifen by either the tetracycline (Tet)- or estrogen receptor (ERT2)–dependent inducible system, respectively. This third method allows for the incomplete and pulse labeling of certain cell lineages, permitting the tracking of the fate and migratory behavior of individual cells in real time.
HoxB7-EGFP is the first fluorescent transgene developed to visualize renal development. Enhanced green fluorescent protein (EGFP), placed under the control of the HoxB7 promoter, specifically labels the wolffian duct and the ureteric epithelial lineage. HoxB7-EGFP has therefore proved to be invaluable in studying the rates and pattern of ureteric branching morphogenesis and ureteral development, including disruption of these events in the context of particular mutant backgrounds. The HoxB7–myr-Venus transgene, designed to express a membrane-bound myristoylated variant of EGFP (myr-Venus), allows for the visualization of individual ureteric epithelial cells by confocal microscopy, thus facilitating observation of changes in cell shape and position. Other fluorescent transgenes for imaging of ureteric epithelia are Ret-EGFP and Ksp-cadherin ( Cdh16-EGFP ). In Ret-EGFP mice, EGFP expression is most prominent in the ampullary tips of the UB. In contrast, fluorophore expression is restricted in the UB trunk and stalk, and absent in the UB tips, in Cdh16-EGFP mice. An ingenious strategy involving the creation of chimeric animals with wild-type epithelial cells expressing HoxB7-EGFP that are intermingled with cells derived from mutant ES cells engineered to express CFP (cyan fluorescent protein) under the control of HoxB7-Cre unraveled the distinctive dependence on genes such as Ret, Etv4, Etv5, and Spry1 in the cellular sorting and rearrangement needed for ureteric branching ( Figure 1.11 ). Inducible transgene expression systems can be very useful in labeling a small subset of cells to enable the fate of the cells to be monitored temporally. A tamoxifen-inducible strategy to mark ureteric epithelial cells with myr-Venus has been cleverly used to observe the unique manner in which proliferating UB cells delaminate into the UB lumen and reposition themselves within the expanding UB ampullary tip.

Lgr5-EGFP, Cited1-EGFP, and a variety of Six2-EGFP transgenes have been employed to characterize the self-renewing capacity and multipotency of nephron progenitor cells within the cap mesenchyme. The mechanism by which nephrogenic and ureteric epithelia are physically conjoined via the invasion of the UB tip by distal nephron precursors has been imaged through the targeted expression of myr-Venus under the control of a Six2-Cre driver. A wide variety of fluorescent protein transgenes and Cre transgenes are now available to characterize the development and organization of multiple compartments of the kidney (see Table 1.2 ).
Nonmammalian Model Systems for Kidney Development
Organisms separated by millions of years of evolution from humans still provide useful models to study the genetic basis and function of mammalian kidney development. This continuing feature stems from the facts that all of these organisms possess excretory organs designed to remove metabolic wastes from the body and that genetic pathways involved in other aspects of invertebrate development may serve as templates to dissect pathways in mammalian kidney development. In support of the latter argument, elucidation of the genetic interactions and molecular mechanism of the Neph1 ortholog and nephrin-like molecules SYG-1 and SYG-2 in synapse formation in the soil nematode Caenorhabditis elegans is providing major clues to the function of their corresponding genes in glomerular and slit diaphragm formation and function in mammals.
The excretory organs of invertebrates, which differ greatly in their structure and complexity, range in size from a few cells in C. elegans to several hundred cells in the malpighian tubules of the fly Drosophila to the more recognizable kidneys in amphibians, birds, and mammals. In C. elegans , the excretory system consists of a single large H-shaped excretory cell, a pore cell, a duct cell, and a gland cell. C. elegans provides many benefits as a model system: the availability of powerful genetic tools including “mutants by mail,” short life and reproductive cycle, publicly available genome sequence and resource database ( www.wormbase.org ), the ease of performing genetic enhancer-suppressor screens in worms, and the fact that they share many genetic pathways with mammals. Major contributions to our understanding of the function of polycystic and cilia-related genes have been made from studying C. elegans. The PKD1 and PKD2 homologs in C. elegans, lov-1 and lov-2 , are involved in cilia development and function of the mating organ required for mating behavior. Strides in understanding the function of the slit diaphragm have also been made from studies of C. elegans, as described earlier.
In Drosophila , the “kidney” consists of malpighian tubules that develop from the hindgut and perform a combination of secretory, resorption, and filtering functions. They express a number of mammalian gene homologs (e.g., Cut, members of the Wingless pathway) that have subsequently been shown to play major roles in mammalian kidney development. Furthermore, studies on myoblast fusion and neural development in Drosophila —two processes that may not appear to be related to kidney development at first glance—have provided major clues to the development and function of slit diaphragms. Mutations in the fly Neph ortholog, irregular chiasm C-roughest ( irreC-rst ), are associated with neuronal defects and abnormal patterning of the eye.
The pronephros, which is only the first of three stages of kidney development in mammals, is the final and only kidney of jawless fishes, whereas the mesonephros is the definitive kidney in amphibians. The pronephros found in larval stage zebrafish ( Dario rerio ) consists of two tubules connected to a fused, single, midline glomerulus. The zebrafish pronephric glomerulus expresses many of the same genes found in mammalian glomeruli (e.g., Vegfa, Nphs1, Nphs2, and Wt1 ) and contains podocytes and fenestrated endothelial cells. Advantages of the zebrafish as a model system include its short reproductive cycle, transparency of the larvae with easy visualization of defects in pronephric development without sacrifice of the organism, availability of the genome sequence, the ability to rapidly knock down gene function with morpholino oligonucleotides, and the ability to perform functional studies of filtration using fluorescently tagged labels of varying sizes. These features make zebrafish amenable to both forward and reverse genetic screens. Currently, multiple laboratories perform knockdown screens of mammalian homologs and genomewide mutagenesis screens in zebrafish in order to study renal function.
The pronephros of the clawed frog Xenopus laevis has also been used as a simple model to study early events in nephrogenesis. As in the fish, the pronephros consists of a single glomus, paired tubules, and a duct. The fact that X. laevis embryos develop rapidly outside the body (all major organ systems are formed by 6 days of age), the ease of injecting DNA, messenger RNA, and protein, and the ability to perform grafting and in vitro culture experiments establish the frog as a valuable model system for dissection of early inductive and patterning cues. In addition, insights emerging from the use of the chick embryo as a model for mesonephros development have highlighted the role of the Vg1/Nodal signaling pathway in formation of the intermediate mesoderm as the embryonic source of all kidney tissue in vertebrates.
Genetic Analysis of Mammalian Kidney Development
Much has been learned about the molecular genetic basis of kidney development over the past 15 years. This understanding has been gained primarily through the phenotypic analysis of mice carrying targeted mutations that affect kidney development. Additional information has been gained by identification and study of genes expressed in the developing kidney, even though the targeted mutation, or knockout, either has not yet been performed or has not affected kidney development or function. This section categorizes the genetic defects on the basis of the major phenotype and stage of disrupted development. It must be emphasized that many genes are expressed at multiple points of renal development and may play pleiotropic roles that are not entirely clear.
Interaction of the Ureteric Bud and the Metanephric Mesenchyme
The molecular analysis of the initiation of metanephric kidney development has included a series of classic experiments using organ culture systems that allow separation of the UB and the MM as well as a later analysis of many gene-targeted mice with phenotypes that included various degrees of renal agenesis. As previously mentioned, the organ culture system has been in use since the seminal experiments, beginning in the 1950s, of Grobstein, Saxen, and their colleagues. These experiments showed that the induction of the mesenchymal-to-epithelial transformation within the MM required the presence of an inducing agent provided by the UB. The embryonic neural tube was found to be able to substitute for the epithelial bud, and experiments involving the placement of the inducing agent on the opposite side of a porous filter from the mesenchyme provided information about the degree of contact required between them. A large series of experiments using organ cultures provided information about the timing of appearance of different proteins normally observed during the induction of nephrons and about the intervals that were crucial in maintaining contact between the inducing agent and the mesenchyme to obtain induction of tubules.
The work with the organ culture system provided an extensive framework on which to base further studies of organ development, and the system remains in extensive use to this day. However, the modern era of studies on the early development of the kidney began with the observation of renal agenesis phenotypes in gene-targeted or knockout mice, the earliest among these being the knockout of several transcription factors, including the WT1, Pax2, Eya1, Osr1/Odd1, Six1, Sall1, Lhx1/Lim1, and Emx2. The knockout of several secreted signaling molecules, such as GDNF, GDF11, gremlin (Grem1), and the receptors Ret and GFRα1 (GDNF family receptor alpha1), also resulted in renal agenesis, at least in the majority of embryos.
Early Lineage Determination of the Metanephric Mesenchyme
In most embryos exhibiting renal agenesis, an appropriately localized putative MM is often uninvaded by a UB outgrowth. Two exceptions are the Osr1/Odd1 and Eya1 mutant embryos, in which this distinct patch of MM is absent, suggesting that Osr1 and Eya1 represent the earliest determinants of the MM yet identified ( Figure 1.12 ). Together, the phenotypes of these knockout mice have provided an initial molecular hierarchy of early kidney development. Osr1 is localized to mesenchymal cells within the mesonephric and metanephric kidney and is subsequently downregulated upon epithelial differentiation. Mice lacking Osr1 do not form the MM and do not express several other factors required for metanephric kidney formation, including Eya1, Six2, Pax2, Sall1, and GDNF. Other factors implicated in the earliest stages of MM cell fate determination are the Eya1/Six1 pathway. Eya1 and Six1 mutations are found in humans with branchiootorenal (BOR) syndrome. It is now known through in vitro experiments that the proteins Eya1 and Six1 form a regulatory complex that appears to be involved in transcriptional regulation. Interestingly, Eya1 was shown to have an intrinsic phosphatase activity that regulates the activation of the Eya1/Six1 complex. Moreover, Eya and Six family genes are co-expressed in several tissues in mammals, Xenopus, and Drosophila , further supporting a functional interaction between these genes. Direct transcriptional targets of this complex appear to include the pro-proliferative factor c-Myc. In the Eya1 -deficient urogenital ridge the putative MM is completely absent. Consistent with this finding, Six1 is either absent or poorly expressed in the presumptive location of the MM of Eya1 -null embryos. These findings may identify Eya1 as a gene involved in early commitment of this group of cells to the metanephric lineage. Although Six1 and Eya1 may act in a complex together, the Six1 phenotype is somewhat different, in that a histologically distinct mesenchyme is present at 11.5 dpc, without an invading UB, similar to the other renal agenesis phenotypes. Eya1 is expressed in the Six1 -null mesenchyme, suggesting that Eya1 is upstream of Six1 . Additionally, Sall1 and Pax2 are not expressed in the Six1 mutant mesenchyme even though WT1 is expressed. There are discrepancies in the literature about Pax2 expression in Six1 mutant embryos, which may reflect the exact position along the anterior-posterior axis of the urogenital ridges of Six1 mutant embryos from which sections are obtained.

Ureteric Bud Induction: Transcriptional Regulation of GDNF
In many cases of renal agenesis, a failure of the GDNF-Ret signaling axis has been identified. GDNF, a member of the tumor growth factor-β (TGF-β) superfamily and secreted by the MM, activates the Ret-GFRα1 receptor complex that is expressed by cells of the nephric duct and the UB. Activation of the Ret tyrosine kinase is of central importance to UB induction. Most mutant embryos lacking Gdnf, Ret, or Gfrα1 exhibit partial or complete renal agenesis owing to severe impairment of UB induction, whereas exogenous GDNF is suffice to induce sprouting of ectopic buds from the nephric duct. Consistently, other genes linked to renal agenesis are known to regulate the normal expression of GDNF. These include genes encoding for transcription factors (e.g., Eya1, Pax2, Six1, Hox11 paralogs, and Sall1) and proteins required to stimulate or maintain GDNF expression (e.g., GDF11, Kif26b, nephronectin, α 8 β 1 -integrin, and Fras1) (see Figure 1.12 ).
As described earlier, Eya1 mutants fail to form the MM. Pax2, a transcriptional regulator of the paired box (Pax) gene family, is expressed widely during the development of both UB and mesenchymal components of the urogenital system. In Pax2 -null embryos, Eya1, Six1, and Sal11 are expressed, suggesting that the Eya1/Six1 is likely upstream of Pax2. Through a combination of molecular and in vivo studies, it has been demonstrated that Pax2 appears to act as a transcriptional activator of GDNF and regulates the expression of Ret. Pax2 also appears to regulate kidney formation through epigenetic control because it is involved in the assembly of a histone H3, lysine 4 methyltansferase complex through the ubiquitously expressed nuclear factor PTIP (pax transcription activation domain interacting protein), which regulates histone methylation. The Hox genes are conserved in all metazoans and specify positional information along the body axis. Hox11 paralogs include Hoxa11, Hoxc11, and Hoxd11 . Mice carrying mutations in any one of these genes do not have kidney abnormalities; however, triple-mutant mice for these genes demonstrate a complete absence of metanephric kidney induction. Interestingly, in these mutants, the formation of condensing MM and the expressions of Eya1, Pax2, and WT1 remain unperturbed, suggesting that Hox11 is not upstream of these factors. Although there seems to be some hierarchy, Eya1, Pax2, and Hox11 appear to form a complex to coordinately regulate the expression of GDNF.
Sall1 indirectly controls the expression of GDNF. Sall1 is necessary for the expression of the kinesin Kif26b by the MM cells. In the absence of either Sall1 or Kif26b, the nephronectin receptor α 8 β 1 -integrin expressed by the MM mesenchyme is downregulated. The loss of Sall1, Kif26b, α 8 β 1 -integrin, and nephronectin compromises the adhesion of the MM cells to the UB tips, ultimately causing loss of GDNF expression and failure of UB outgrowth. , Loss of the extracellular matrix protein Fras1—the gene which is linked to Fraser’s syndrome and which is expressed selectively in the UB epithelium and nascent epithelialized nephrons but not the MM—causes loss of GDNF expression. Fras1 likely regulates MM induction and GDNF expression via multiple signaling pathways. Fras1 deficiency results in downregulation of GDF11, Hox11, Six2, and α 8 -integrin, and an increase in bone morphogenetic protein 4 (BMP4), which cooperatively controls GDNF expression.
Non-GDNF Pathways in the Metanephric Mesenchyme
Another pathway in early development of the MM involves WT1 and vascular endothelial growth factor A (VEGF-A). Induction of the UB does not occur in Wt1 mutants, although GDNF is expressed in the MM, indicating the existence of a GDNF-independent UB induction mechanism. However, details of this pathway still remain to be clarified. A novel approach to the organ culture system involving microinjection and electroporation has also yielded insights as to a possible function of the Wt1 gene in early kidney development. Overexpression of WT1 from an expression construct led to high-level expression of VEGF-A. The target of VEGF-A appeared to be Flk1 (VEGF receptor 2 [VEGFR2])–expressing angioblasts at the periphery of the mesenchyme. Blocking signaling through Flk1, if done when the metanephric rudiment was placed in culture, blocked expression of Pax2 and GDNF and, consequently, of the continued branching of the UB and induction of nephrons by the bud. Blockade of Flk1 after the organ had been in culture for 48 hours had no effect, indicating that the angioblast-derived signal was required to initiate kidney development but not to maintain continued development. The signal provided by the angioblasts is not yet known, nor is it known whether WT1 is a direct transcriptional activator of VEGF-A. Flk1 signaling is also required to initiate hepatocyte differentiation during liver development. Numerous targets of WT1 in nephron progenitors have been identified though chromatin immunoprecipitation, providing a comprehensive catalog of genes particularly enriched for functions relating to transcription, multiorgan development, and cell cycle regulation. In addition, a number of these WT1 targets have special roles in remodeling of the actin cytoskeleton.
Genes Required by the Ureteric Bud in Early Kidney Development
Several components of the genetic network supporting the development of the nephric duct and the UB have been identified (see Figure 1.12 ). Pax2 and Pax8 are required to maintain the expression of Lhx1 . Pax2, Pax8, and Lhx1 altogether likely coordinate the expression of Gata3, which is necessary for elongation of the nephric duct. Gata3 and Emx2, which are required for the expression of Ret in the nephric duct, are both regulated by β-catenin, an effector of the canonical Wnt signaling pathway (for a discussion of Wnt, see section “Molecular Analysis of the Nephrogenic Zone”). Acting likely in parallel with Gata3 to maintain Ret expression in the UB is Aldh1a2 (Raldh2) , a gene in the retinoic acid synthesis pathway. Surprisingly, this genetic regulatory hierarchy cannot fully account for the distinctive phenotypes arising from the mutations of individual genes, suggesting that additional important components of the nephric duct genetic network have yet to be identified. Nephric duct specification fails in Pax2/Pax8 mutants but not in the case of Lhx1 deficiency, in which only the caudal portion of the nephric duct degenerates. The absence of Gata3 or Raldh2 causes misguided elongation of the nephric duct, which terminates into either blind-ended ureters or abnormal connections between the bladder and urethra. The curtailed caudal growth of the nephric duct when either Lhx1 or Gata3 is lost prevents the formation of the first UB and consequently causes renal agenesis. The absence of Aldh1a2 leads to the formation of ectopic ureters and hydronephrotic kidneys. Emx2 deficiency does not prevent caudal extension of the nephric duct toward the presumptive MM, but the evagination of the UB is aborted, thereby resulting in renal agenesis. Without β-catenin, nephric duct cells undergo precocious differentiation into collecting duct epithelia. Ret does not affect the nephric duct fate but has importance in later UB development and insertion of the nephric duct into the cloaca. Identification of additional targets of Pax2, Pax8, Lhx1, Gata3, and β-catenin are necessary in order to fully understand these seemingly disparate mutant phenotypes.
UB induction and subsequent branching require a unique spatial organization of Ret signaling. The bulbous UB tip is a region enriched with proliferative ureteric epithelial cells, in contrast to the emerging stalk regions of the developing ureteric tree. It is now well appreciated that receptor tyrosine kinase (RTK) signaling primarily through Ret is key to the proliferation of UB tip epithelia. Exogenous GDNF supplemented in explanted embryonic kidneys can cause expansion of the UB tip region toward the source of the ligand. Erk kinase activation is prominent within the ampullary UB terminals, where Ret expression is elevated. Consistently, chimera analysis in mice reveals that Ret-deficient cells do not contribute to the formation of the UB tips. All together, these studies underscore the importance of strategic levels of Ret expression and activation of proliferative signaling pathways in the stereotypical sculpting of the nascent collecting duct network.
A ligand-receptor complex formed by GDNF, GFRα1, and Ret is necessary for autophosphorylation of Ret on its intracellular tyrosines ( Figure 1.13 ). A number of downstream adaptor molecules and effectors have been identified to interact with active phosphorylated Ret, including the growth factor receptor–bound proteins Grb2, Grb7, and Grb10, ShcA, Frs2, phospholipase C γ1 (PLC γ1 ), Shp2, Src, and Dok adaptor family members (Dok4/5/6). These downstream Ret effectors together are likely contributors to the activation of the Ras/SOS/Erk and PI3K/Akt pathways supporting the proliferation, survival, and migratory behavior of the UB epithelium. Knock-in mutations of the interaction site for Shc/Frs2/Dok adaptors on the short isoform of Ret lead to the formation of rudimentary kidneys. Specific mutation of the PLC γ1 docking site on Ret leads to renal dysplasia and ureter duplications. The loss of Shp2 in the UB lineage also causes severe renal hypoplasia, phenocopying that is observed in occasional Ret-deficient kidneys. UB-specific inactivation of Pten , a target of the PI3K/Akt pathway, disrupts UB branching. Taken together, these findings underscore the significance of Ret signaling in normal UB branching.

A number of transcriptional targets of Ret activation in microdissected UB stimulated with GDNF have been elucidated (see Figure 1.13 ). Among these are Ret itself and Wnt11, which stimulates GDNF expression in the MM, suggesting that a positive feedback loop exists for the GDNF-Ret signaling pathway. Ret activation also positively regulates the ETS (E26 transformation-specific) transcription factors Etv4 and Etv5, which are also necessary for normal UB branching morphogenesis. Etv4 -null homozygous mutants and compound heterozygous mutants for Etv4 and Etv5 manifest severe renal hypoplasia or renal agenesis, suggesting that these transcription factors are indispensable targets of Ret for proper UB development. In chimeric animals Etv4/Etv5-deficient cells, just like Ret-deficient cells, fail to integrate within the UB tip domain.
The gene Sprouty was identified as a general antagonist of RTKs and was discovered for inhibiting the fibroplastic growth factor (FGF) and epidermal growth factor (EGF) signaling pathways that pattern the Drosophila airways, wings, and ovarian follicles. Of the four mammalian Sprouty homologs, Spry1, Spry2, and Spry4 are expressed in developing kidneys. Spry1 is expressed strongly at the UB tips, whereas Spry2 and Spry4 are found in both the UB and the MM. Sprouty molecules are thought to uncouple receptor tyrosine kinases with the activation of ERK pathway either through competitive binding with the Grb2/SOS complex or through the kinase Raf, effectively repressing ERK activation. Interestingly, Spry1 expression is distinctively upregulated upon GDNF activation of Ret. This finding suggests that Ret activates a negative feedback mechanism via Spry1 in order to control activated ERK levels and modulate cell proliferation in the UB. Studies on Spry1 -knockout mice reveal some intriguing facets about Ret dependence of UB induction and branching. Spry1 deficiency leads to ectopic UB induction and can rescue renal development in the absence of either GDNF or Ret. Germline inactivation of Spry2 does not overtly affect renal development but can rescue renal hypoplasia in mice engineered to express Ret mutants impaired in activating the Ras/ERK pathway. The transcriptional targets of Ret, such as Etv4, Etv5 and Wnt11, are retained in Gdnf/Spry1 or Ret/Spry1 compound null mutants. These findings indicate that Ret signaling is not absolutely required for UB development. In fact, signaling via FGF10 and the receptor FGFR2 is sufficient for renal development despite the absence of GDNF or Ret, provided that Spry1 is inactivated. Nevertheless, patterns of renal branching are distinctively altered in Gdnf/Spry1 and Gdnf/Ret compound mutants, with UB tips often displaying more heterogeneous shapes and orientation. These findings indicate that there remain some distinctive roles of GDNF-Ret signaling that cannot be fully compensated by FGF10/FGFR2 during UB development.
Adhesion Proteins in Early Kidney Development
A current theme in cell biology is that growth factor signaling often occurs coordinately with signals from the extracellular matrix transduced by adhesion receptors, such as members of the integrin family. α 8 β 1 -integrin is expressed by cells of the MM interacting with the novel ligand nephronectin expressed specifically by UB cells. In most embryos with mutations causing absence of α 8 -integrin, UB outgrowth is arrested upon contact with the MM. In a small portion of embryos, this block is overcome, and a single, usually hypoplastic, kidney develops. Nephronectin gene (Npnt) knockout mice exhibit renal agenesis or severe hypoplasia. Thus, the interaction of α 8 β 1 -integrin with nephronectin must have an important role in the continued growth of the UB toward the MM. Phenotypes of both Itga8 and Npnt knockout mice appear to result from a reduction in GDNF expression. The attraction of the UB to the mesenchyme is also governed by the maintenance of proper cell-cell adhesion within mesenchymal cells. Kif26b, a kinesin specifically expressed in the MM, is important for tight condensation of mesenchymal cells. Genetic inactivation of Kif26b results in renal agenesis due to impaired UB induction. In Kif26b mutant mice, the compact aggregation of mesenchymal cells is compromised, resulting in distinctive loss of polarized expression of α 8 -integrin and severe downregulation of GDNF expression. Hence, dysregulation of mesenchymal cell adhesion causes the failure to attract and induce the ureteric epithelia.
Genetic evidence further shows that nephronectin localization at the basement membrane of the UB is critical for GDNF expression by the MM. Genetic inactivation of basement membrane proteins associated with Fraser’s syndrome (Fras1, Frem1/Qbrick, and Frem2) leads to renal agenesis characterized by severe downregulation of GDNF expression. On the basis of interaction of nephronectin with Fras1, Frem1/Qbrick, and Frem2, it has been proposed that the Fras1/Frem1/Frem2 ternary complex anchors nephronectin to the UB basement membrane, thus stabilizing engagement with α 8 β 1 -integrin expressed by the MM ( Figure 1.14 ). Grip1, a PDZ domain protein known to interact with Fras1, is required to localize the Fras1/Frem1/Frem2 complex on the basal aspect of the UB epithelium. Grip1 mutations phenocopy Fraser’s syndrome, including renal agenesis, thus further highlighting the importance of the strategic localization of nephronectin on the UB surface toward the opposing MM.

The establishment of epithelial basement membranes during metanephric kidney development involves the stage-specific assembly of different laminin α and β subunits with a common laminin γ 1 subunit. The UB-specific inactivation of the gene Lamc1, which encodes for laminin γ 1 , leads to impaired UB induction and branching, ultimately causing either renal agenesis or hypomorphic kidneys with water transport deficits. Lamc1 deficiency prevents formation of basement membranes, causing downregulation of both growth factor (GDNF/Ret, Wnt11, and FGF2)–based and integrin-based signaling. This fact is another example of how signaling through the extracellular matrix intersects with growth factor signaling to influence morphogenesis. The importance of basement membrane assembly in the development of other renal structures is emphasized by genetic studies on the genes Lama5 and Lamb2, which encode for laminins α 5 and β 2 , respectively. Loss of Lama5 causes either renal agenesis or disruption of glomerulogenesis, whereas deficiency of Lamb2 leads to a defective glomerular filtration barrier.
The UB branching program is stereotypically organized so that the proliferative UB epithelial cells are largely confined to the bulbous UB tips but cell division is dampened within the elongated nonbranching UB stalks of the growing ureteric tree. TROP2/Tacstd2, an adhesion molecule related to epithelial cell adhesion molecule (EpCAM), is expressed prominently in the UB stalks, where it colocalizes with collagen-1. TROP2, unlike EpCAM, which is expressed throughout the UB tree, is not expressed at the UB ampullary tips. Consistently, dissociated and sorted UB cells expressing high levels of TROP2 are nonproliferative and express low levels of Ret, GFRα1, and Wnt11, which are notable UB tip markers. Elevated expression of TROP2 is also associated with poor attachment of epithelial cells to collagen matrix and with suppression of cell spreading and motility, thus emphasizing the importance of this adhesion molecule in negative regulation of UB branching and the sculpting of the nascent collecting duct network. The formation of patent lumens within epithelial tubules of the kidney also depends on coordinated cell adhesion. β 1 -integrin is tethered to the actin cytoskeleton via a ternary complex formed between integrin-like kinase (ILK) and parvin. ILK has been shown to be important in mediating cell cycle arrest and cell contact inhibition in the collecting duct epithelia. The targeted ablation of Ilk expression in the UB does not cause remarkable defects in UB branching but does eventually lead to postnatal death due to obstruction of collecting ducts arising from dysregulated intraluminal cell proliferation. Thus, cell adhesion molecules may suppress cell division to regulate distinctive aspects of renal branching and tubulogenesis.
Formation of the Collecting System
The overall shape, structure, and size of the kidneys are largely guided by the stereotypical branching of the UB and the subsequent patterning of the collecting duct system. During late gestation, past embryonic stage 15.5 dpc in the mouse, the trunks of the UB tree undergo extensive elongation to establish the array of collecting ducts found in the renal medulla and papilla. The radial arrangement of elongated collecting ducts together with the loops of Henle (derived from the nephrogenic mesenchyme) establishes the corticomedullary axis by which nephron distributions are patterned. Further elongation of the newly formed collecting duct network after birth is partly responsible for the postnatal growth of the kidney.
Elongation of the collecting ducts is regulated by oriented cell division, a process dependent on Wnt7b and Wnt9b. Oriented cell division is characterized by the parallel alignment of the mitotic spindle of proliferating ductal epithelia with the longitudinal axis of the duct. Oriented cytokinesis, therefore, guarantees that the daughter cells contribute to lengthening of the duct with minimal effect on tubular lumen diameter. The renal medulla and pelvis are nonexistent in mice lacking Wnt7b. Notably, the collecting ducts and loops of Henle are stubbier, likely through disruption of oriented cell division. Wnt7b expression is restricted within the UB trunks and is absent in the ampullary UB tips. Oriented cell division of the collecting duct epithelia therefore requires reciprocal signaling with the surrounding interstitial stromal mesenchyme. Conditional inactivation of Cttnb1 (β-catenin) using a Tcf21-Cre transgene (which is expressed in the interstitial stroma) results in hypoplastic kidneys lacking medullary and papillary regions. This is consistent with the possibility that the UB-stromal interaction via Wnt7b activates the canonical β-catenin–dependent Wnt signaling pathway. Wnt9b, another ligand expressed along the UB trunk region, has been identified as required for oriented cell division in collecting duct cells. In contrast, Wnt9b signals through a noncanonical Wnt pathway involving the activation of the small guanosine triphosphatase (GTPase) RhoA and the kinase Jnk. Another mechanism that could contribute to elongation of the collecting ducts is convergent extension. Convergent extension involves the coordinated intercalation of elongated epithelial cells that thereby narrows and effectively lengthens the ducts. This mechanism was proposed on the basis of the reconfigured orientation of elongated cells in Wnt9b mutant collecting ducts. How the interstitial stroma signals back to the UB to modulate oriented cell division and convergent-extension remains unknown.
The normal development of the collecting ducts also depends on cell survival cues provided by diverse ligands such as Wnt7b, EGF, and hepatocyte growth factor (HGF) and on interactions with the extracellular matrix. Papillary collecting ducts display higher incidence of apoptosis in mice lacking Wnt7b or EGF receptor (EGFR). Conversely, loss of Dkk1 (Dickkopf1), a secreted antagonist of Wnt7b, results in overgrowth of the renal papilla. Conditional inactivation of Dkk1 using the Pax8-Cre transgene (expressed in renal tubules and the collecting ducts) causes increased proliferation of papillary epithelial cells. The HGF receptor Met, α 3 β 1 -integrin (Itga3/Itgb1) , and laminin α 5 (Lama5) are all required to maintain the expression of Wnt7b and thus are likely to support the viability of collecting duct cells.
Poor development of the renal medulla and papilla are also observed in mutant mice lacking FGF7, FGF10, FGFR2, BMPR1A (ALK3), the components of the renin angiotensin aldosterone system (RAAS), Shh (Sonic hedgehog), or the orphan nuclear steroid hormone receptor Esrrg. FGF7 and FGF10 are the cognate ligands of FGFR2. Renal hypoplasia observed when Fgfr2 is conditionally removed from the ureteric lineage is more severe than in mutants lacking Fgf7 or Fgf10, suggesting that these related ligands may have some functional redundancy in the development of the UB and collecting ducts. Kidneys lacking Bmp1ra show an attenuated phosphorylation of SMAD1, an effector of the BMP and TGFβ ligands, and a concomitant increase in expression of c-Myc and β-catenin. Although the significance of these results are not clear, the elevation of β-catenin indicates a novel crosstalk between BMP and Wnt signaling pathways in collecting ducts. Signaling through angiotensin is relevant to both early UB branching and the morphogenesis of medullary collecting ducts. Genetic inactivation of angiotensinogen, its processing enzyme angiotensin-converting enzyme (ACE), and its target angiotensin-II AT1R receptors (Agtr1a and Agtr1b) results in similar phenotypes characterized by hypoplastic kidneys with modestly sized renal papillae. Furthermore, the postnatal growth and survival of renal papilla grown ex vivo depend on the presence of AT1R. Interestingly, in cultures of renal papilla explants, angiotensin appears to regulate the Wnt7b, FGF7, and α 3 β 1 -integrin signaling pathways such that the loss of endogenous angiotensin or pharmacologic inhibition of AT1R causes significant dampening of the expression of Wnt7b, Fgf7, Cttnb1, and Itga3/Itgb1 . Shh is expressed in the more distal derivatives of the UB, the medullary collecting ducts and the ureter. The germline deletion of Shh results in either bilateral renal agenesis or a single ectopic dysplastic kidney. It has been shown that Shh controls the expression of early inductive and patterning genes ( Pax2 and Sall1 ), cell cycle regulators (N-myc and cyclin D1), and signaling effectors of the Hedgehog pathway ( Gli1 and Gli2 ). Interestingly, genetic removal of Gli3 on an Shh -null background restores the expression of Pax2, Sall1, cyclin D1, N-Myc, Gli1 , and Gli2, providing physiologic proof for the role of Gli3 as a repressor of the Shh pathway in renal development. Frameshift mutations resulting in truncation of the expressed Gli3 protein is linked to Pallister-Hall syndrome and the presence of hydronephrosis and hydroureter in both humans and mice. Esrrg has a strong and localized expression within collecting duct epithelia later in gestation, and its inactivation in mice causes complete aplasia of the renal medulla and papillae. However, the ligand of Esrrg remains to be identified, and little is known about its downstream targets.
Positioning of the Ureteric Bud
A crucial aspect of kidney development that is of great relevance to renal and urologic congenital defects in humans relates to the positioning of the UB (see Figure 1.12 A ). Incorrect positioning or duplication of the bud leads to abnormally shaped kidneys and incorrect insertion of the ureter into the bladder, with resultant ureteral reflux that can predispose to infection and scarring of the kidneys and urologic tract.
Foxc1 (Forkhead box C1) is a transcription factor of the Forkhead family, expressed in the intermediate mesoderm and the MM adjacent to the wolffian duct. In the absence of Foxc1 , the expression of GDNF adjacent to the wolffian duct is less restricted than in wild-type embryos. Foxc1 deficiency results in ectopic UBs, hypoplastic kidneys, and duplicated ureters. Additional molecules that regulate the location of UB outgrowth are Slit2 and Robo2, signaling molecules best known for their role in axon guidance in the developing nervous system. Slit2 is a secreted factor, and Robo2 is its cognate receptor. Slit2 is mainly expressed in the Wolffian duct, whereas Robo2 is expressed in the mesenchyme. In one study, UBs formed ectopically in embryos deficient in either Slit2 or Robo2 similar to those in the Foxc1 mutant. However, in contrast to the Foxc1 phenotype, ureters in the Slit2/Robo2 mutants undergo remodeling allowing their insertion into the bladder. Instead, the ureters remained connected to the nephric duct in Slit2 or Robo2 mutants. The domain of GDNF expression is expanded anteriorly in the absence of either Slit2 or Robo2 . Indeed, mutations in Robo2 have been identified in patients with vesicoureteral junction defects and vesicoureteral reflux. The expressions of Pax2, Eya1, and Foxc1, all thought to regulate GDNF expression, were not dramatically different in the absence of Slit2 or Robo2, suggesting that Slit/Robo signaling is not upstream of these genes. It is possible that Slit/Robo signaling is regulating the point of UB initiation by regulating the GDNF expression domain downstream of Pax2 or Eya1 . An alternative explanation is that Slit2 and Robo2 act independently of GDNF and that the expanded GDNF domain is a response to rather than a cause of ectopic UBs.
Spry1, as described earlier, negatively regulates the Ras/Erk signaling pathway and is expressed strongly in the posterior wolffian duct and the UB tips. Embryos lacking Spry1 develop supernumerary UBs, but unlike mutants of Foxc1, Slit2, or Robo2, they do not display changes in GDNF expression. The phenotype of Spry1 mutants can be rescued by reducing the GDNF expression dosage. Spry1 deletion also rescues the renal agenesis defect in mice lacking either Ret or GDNF. Consistently, renal agenesis and severe renal hypoplasia, in mice expressing Ret specifically mutated on a tyrosine phosphorylation site known to couple with the Ras/ERK pathway, can be reversed in the absence of Spry1 . Thus, Spry1 appears to regulate UB induction site by dampening RTK-dependent proliferative signaling.
Another negative regulator of branching is BMP4, which is expressed in the mesenchyme surrounding the wolffian duct. Bmp4 heterozygous mutants have duplicated ureters, and in organ culture, BMP4 blocks the induction of ectopic UBs by GDNF-soaked beads. Furthermore, knockout of gremlin, a secreted BMP inhibitor, causes renal agenesis, supporting a role for BMP in the suppression of UB formation.
Molecular Analysis of the Nephrogenic Zone
The continued replenishment of the reservoir of nephron progenitors during kidney development is crucial to guarantee generation of a sufficient number of nephrons. Fate mapping studies in mice using Cre driven by Cited1 and Six2 promoters demonstrate that the condensed mesenchyme, which aggregates around the UB, represents a pool of multipotent progenitors that replenishes itself and differentiates to give rise to all epithelial components of the nephron from podocytes to distal tubules. Signaling through Wnt, FGF, and the BMP family of ligands is critical to maintain the delicate balance between progenitor self-renewal and differentiation toward a nephrogenic fate.
Wnt11 and Wnt9b, two ligands belonging to the Wnt family of signaling molecules, are expressed by the UB. The Wnt family was originally discovered as the wingless mutation in Drosophila and, in mammals, as genes found at retroviral integration sites in mammary tumors in mice. Wnt11 is highly expressed at the UB tips and decreased branching occurs in its absence, although it has no known specific effect on the induction of the epithelial transformation of the MM. Wnt11 is a downstream target of Ret and is necessary to sustain GDNF expression in the MM. Hence, Wnt11 participates in an autoregulatory feedback loop that maintains GDNF-Ret signaling to promote UB branching. In contrast, Wnt9b, which is expressed in the entire UB except the very tips, appears to be the vital molecule expressed by the UB that induces the MM. Wnt9b is not essential for the early induction of the UB or for the initial condensation of the MM. Further UB branching fails beyond the initial branching step resulting in T-shaped tubule (T-stage), however, likely because of downregulation of GDNF in the MM. The MM condenses up to the T-stage but the expressions of Pax2, Eya1, WT1, Bmp7, and Six2 are distinctively diminished by 12.5 dpc in Wnt9b mutant mouse embryos. This loss of MM markers leads to failed induction of renal vesicles and tubulogenesis. Thus, Wnt9b is the closest candidate identified to date, which is likely to be the crucial molecule produced by the bud that stimulates induction of the nephrons.
A third member of the Wnt family, Wnt4, is expressed in pretubular aggregates and is additionally required for the epithelial transformation of the MM. In Wnt4 mutant embryos, pretubular aggregates failed to epithelialize into the tubular precursor of the mature nephron. Wnt9b -deficient MM could be sufficiently induced in vitro to undergo tubulogenesis when grown with Wnt4-expressing fibroblasts. In contrast, another study using the same co-culture assay showed that Wnt9b could not compensate for the loss of Wnt4. These findings suggest that Wnt9b and Wnt4 likely bind distinctive receptor complexes, with Wnt4 acting downstream of Wnt9b. Thus, a model has been proposed whereby Wnt9b acts as a paracrine factor, priming the MM to develop into renal vesicles expressing Wnt4. Wnt4 in this model functions as an autocrine factor required for commitment to a tubulogenesis program ( Figure 1.15 ).

Two major Wnt signaling branches exist downstream of the Frizzled receptor (Fz): a canonical β-catenin–dependent pathway and a noncanonical β-catenin–independent pathway. In the canonical pathway, Wnt-mediated signaling suppresses a phosphorylation-triggered pathway of proteosomal degradation, enabling the stabilization of β-catenin, which results in the formation of a complex between β-catenin and TCF/LEF (T-cell factor/lymphoid-enhancing factor) DNA-binding proteins that directly regulates transcriptional targets. Numerous studies demonstrate the importance of the canonical Wnt pathway for renal development: Conditional deletion of β-catenin from the cap mesenchyme completely blocks renal vesicle formation as well as expression of markers of induction such as Wnt4, Fgf8, and Pax8. By contrast, activation of stabilized β-catenin in the same cell population causes ectopic expression of mesenchymal induction markers in vitro and functionally rescues the defects observed in Wnt4- or Wnt9b-deficient mesenchymes. Inhibition of the kinase GSK3, a member of the β-catenin degradation complex, results in the ectopic differentiation of the MM.
BMP7 is expressed in the UB and in the condensed MM. Loss of BMP7 causes untimely depletion of the cap mesenchyme and nephrogenesis arrest. BMP7 is thought to be a survival and proliferative factor for the cap mesenchyme, on the basis of organ culture experiments and the increased incidence of apoptosis observed within the presumptive nephrogenic zone of Bmp7 -null kidneys. The proliferative effect of BMP7 on nephron progenitors has been shown to depend on specific activation of the kinase Jnk leading to phosphorylation and activation of Jun and Atf2. However, the cell-survival promoting functions of BMP7 are unlikely specific since BMP4 can functionally substitute for loss of BMP7 (based on phenotypic rescue in “knocked-in” mutants where Bmp4 cDNA was inserted next to the endogenous Bmp7 promoter). The exact role of BMP4 in nephrogenesis is not known, although it has been described as important specifically within the UB lineage. The transcription factor Trps1, an atypical member of the GATA family of transcription factors implicated in trichorhinophalangeal (TRP) syndrome, has been identified as a novel target of BMP7. Trps1 expression is absent in Bmp7 -null kidneys. Trps1 -null mutant kidneys are hypoplastic and distinctively lacking glomeruli and renal tubules. Renal vesicle formation is distinctively compromised in the absence of Trps1, with a concomitant depletion of the cap mesenchyme. In cultured MM cells, the increased expression of E-cadherin following BMP7 stimulation is inhibited upon RNA interference–mediated knockdown of Trps1. Altogether these studies suggest that BMP7 acting through Trps1 is important for epithelialization of the cap mesenchyme.
The more primitive progenitors within the condensed mesenchyme express high levels of Cited1 and proliferate in a BMP7-dependent manner. In response to BMP7, these Cited1-positive cells begin expressing Six2 and acquire responsiveness to Wnt9b. The exact role of Cited1 in the condensing mesenchyme remains poorly understood because Cited1 – and compound Cited1/Cited2 -knockout kidneys have apparently intact mesenchyme-to-epithelial transitions. It is not clear, however, whether the closely related Cited4 is upregulated and functionally compensates in the absence of Cited1 and Cited2. Genetic inactivation of Six2 causes premature and ectopic nephrogenesis. The precocious epithelialization combined with increased incidence of apoptosis in Six2 -deficient cap mesenchyme rapidly depletes the pool of nephrogenic precursors. The defective maintenance of nephrogenic precursors impairs reciprocal inductive interactions between the cap mesenchyme and the UB, causing overall stunting of kidney growth. Overexpression of Six2, on the other hand, prevented epithelial differentiation of the cap mesenchyme. Six2, therefore, is required to maintain the undifferentiated, self-renewing progenitor states of nephron precursors. Nevertheless, epithelialization in Six2 -null mutants remains dependent on Wnt9b induction. In the absence of Wnt signaling, Six2 constitutively represses expression of renal vesicle markers within nephron progenitors. In response to Wnt induction, Six2 forms a complex with β-catenin and Lef/Tcf factors that regulate the expression of multiple genes required to coordinate mesenchyme-to-epithelial transition, including the upregulation of Pax8, Fgf8, Wnt4, and Lhx1 and the attenuation of Six2 expression. A fine-tuned activity of Six2 is therefore required to balance the maintenance of a pool of self-renewing nephron progenitors and to prime these progenitors for commitment to an epithelial fate via a canonical Wnt-dependent pathway.
The multidomain scaffolding proteins Dlg1 and CASK, members of the MAGUK (membrane-associated guanylate kinase) family of proteins, have been shown to be important in maintenance of nephron progenitor cells. Dlg1 and CASK prominently localize at the plasma membranes of polarized cells, where they coordinate cell junction formation and assembly of protein complexes that regulate cell polarity. In neurons, they are known to be important for organization of synapses. The global deletion of Dlg1 and Cask in mice led to severe renal hypoplasia and dysplasia with notable loss of the nephrogenic zone. This renal phenotype was fully recapitulated when Dlg1 and Cask were removed conditionally using either Pax3-Cre or Six2-Cre, suggesting that the defects are inherent within the MM compartment, particularly the nephrogenic precursors. Although UB branching was also decreased in the global and MM double-knockout mice, this defect proved to be secondary to depletion of the nephrogenic zone, because targeted ablation of Dlg1 and Cask in the ureteric lineage using HoxB7-Cre did not cause renal hypoplasia or abnormal renal histology. Significantly diminished cell proliferation and increased apoptosis were observed in the nephrogenic zone in the absence of Dlg1 and Cask . Consistent with the loss of the nephrogenic zone is the decreased expression of BMP7, Cited1, Six2, and FGF8. GDNF expression is also notably decreased, a finding that could explain the secondary impairment in ureteric branching. The concomitant reduction in BMP7 and FGF8 levels correlates with the dampening of signaling events downstream of Ras, including Erk, Jnk, and p38 MAPK pathways, possibly accounting for the loss of cell proliferation in the nephrogenic zone of Dlg1/Cask double-knockout mice.
The extracellular cues regulating Dlg1 and CASK functions in the nephrogenic mesenchyme are not yet clear. One possibility invoked is the interaction between Dlg1 and CASK with the FGF pathway via syndecan-2. FGF2 is known to mediate condensation of the MM, whereas FGF8 is important for transition to Wnt4-expressing pretubular aggregates and renal vesicles. FGF9 and FGF20, on the other hand, are important to maintain the stemness of nephron progenitors. The corresponding receptors, FGFR1 and FGFR2, are crucial for the survival of the MM without which renal agenesis ensues. Dlg1 and CASK are also likely to mitigate the proper migration and condensation of the nephron precursors around the UB. In compound heterozygous/homozygous Dlg1/Cask knockout subjects, kidneys were only modestly hypoplastic but showed a distinctively loose aggregation of Six2-expressing condensing mesenchyme. This result is consistent with those of other studies showing that Dlg1 is important for directed cell migration of Schwann cells.
Molecular Biology of Nephron Development: Tubulogenesis
Gene targeting and other analyses have identified many genes involved in the initial induction of the metanephric kidney and the formation of the pretubular aggregate, but much less is currently known about how the pretubular aggregate develops into a mature nephron, a process through which a simple tubule elongates, convolutes, and differentiates into multiple distinct segments with different functions. Discussions of how this segmentation occurs have considered whether similarities will be found to other aspects of development, such as the limb or neural tube, where there is segmentation along various axes.
The Notch group of signaling molecules has been implicated in directing segmentation of the nephron. Notch family members are transmembrane proteins, the cytoplasmic domains of which are cleaved by the γ-secretase enzyme upon the interaction of the extracellular domain with transmembrane ligand proteins of the Delta and Jagged families, found on adjacent cells. Thus, Notch signaling occurs between adjacent cells, in contrast to signaling by secreted growth factors, which may occur at a distance from the growth factor–expressing cells. The cleaved portion of the Notch cytoplasmic domain translocates to the nucleus, where it has a role in directing gene expression. Mice homozygous for a hypomorphic allele of Notch2 have abnormal glomeruli, with a failure to form a mature capillary tuft. Because null mutants of Notch family members usually result in early embryonic death, further analysis of Notch family function in kidney development has made use of the organ culture model.
When metanephric rudiments were cultured in the presence of a γ-secretase inhibitor, expression of podocyte and proximal tubule markers was diminished in comparison with expression of distal tubule markers and branching of the UB. When the γ-secretase inhibitor was removed, there seemed to be a better recovery of expression of proximal tubule markers than of podocyte differentiation markers. Similar results were observed in mice carrying targeted mutation of the Psen1 and Psen2 genes that encode a component of the γ-secretase complex. Conditional deletion of Notch2 in the MM resulted in hypoplastic kidneys that did not develop glomeruli and proximal tubules, despite the presence of distal tubules and collecting ducts. Interestingly, the condensed mesenchyme and pretubular aggregates initiated epithelialization expressing Pax2 and E-cadherin but did not proceed to form S-shaped bodies. By contrast, Notch1-deficient metanephroi are phenotypically wild type, suggesting that Notch1 is not critical for cell fate determination during early nephron formation. Taken together, these studies seem to indicate that local activation of Notch2 during tubule morphogenesis is critical to determining the proximal cell fate after the epithelialization of renal vesicle. The transcription factor Rbpj, the homolog of the Drosophila gene Suppressor of Hairless, is a transducer of canonical Notch signaling. Genetic inactivation of Rbpj in the MM leads to pronounced renal hypoplasia characterized by significant paucity in nephrons and the development of tubular cysts. Fate mapping analyses reveal that Rbpj-deficient nephrogenic precursors develop into podocytes and distal tubules but not proximal tubules. These findings further reiterate the crucial importance of canonical Notch signaling via Rbpj in the specification of the proximal segment of nephrons and the likelihood that Notch signaling independent of Rbpj arbitrates the determination of podocyte fate. Consistently, overexpression of the constitutively active Notch1 intracellular domain (N1ICD) drives the acquisition of proximal tubule fate in nephron precursors but inhibits the development of podocytes.
The specification of the distal nephron fate requires the POU domain–containing transcription factor Pou3f3 (Brn1) and the metalloprotease genes Adamts1 and Adamts4 . The proneural basic helix-loop-helix (bHLH) factor Ascl1 (MASH1) binds cooperatively with Pou3f3 and the related Pou3f2 (Brn2) to the promoter of the Notch ligand Delta1 to synergistically activate the transcription of Delta1 and stimulate neurogenesis. Whether Pou3f3 is involved in regulation of Notch signaling in renal development is not clear. Germline deletion of Pou3f3 results in defective patterning of the distal nephron segments. Pou3f3 expression is first detectable in renal vesicles and becomes localized to the distal aspects of the comma- and S-shaped bodies, regions destined to become the distal convoluted tubules, the macula densa, and the loop of Henle. Without Pou3f3, elongation of prospective loop of Henle and overall maturation of distal nephron segments are arrested. Although the development of glomeruli, proximal tubules, and collecting ducts is seemingly not affected by the absence of Pou3f3, the severity of the distal nephron abnormalities causes renal insufficiency and perinatal death. The products of Adamts1 and Adamts4 are secreted thrombospondin domain–containing metalloproteases known to cleave a class of proteoglycans called lecticans . Null mutation of Adamts1 in mice leads to hydronephrosis and is characterized by the thinning of the renal medulla and a distinctive paucity in the loops of Henle. Lack of Adamts4 appears benign but can exacerbate the simplification of the renal medulla due to loss of Adamts1 . As a consequence, mice with a compound null mutation of Adamts1 and Adamts4 mostly perish perinatally. This finding suggests that Adamts1 and Adamts4 have overlapping importance in the development of the distal nephron segment by a mechanism yet to be identified.
There is one example so far of a transcription factor involved in the differentiation of a specific cell type in the kidney. The phenotype is actually found in the collecting ducts, rather than in the nephron itself, but is discussed in this section because it is demonstrative of the kinds of phenotypes expected to be found as additional mutant mice are examined. Two cell types are normally found in the collecting ducts—principal cells, which mediate water and salt reabsorption, and intercalated cells, which mediate acid-base transport. In the absence of the Foxi1 transcription factor, only one cell type is present in collecting ducts, and many acid-base transport proteins normally expressed by intercalated cells are absent.
In addition to cell differentiation, spatial orientation of cells is essential for tubule elongation and morphogenesis. In epithelia, cells are uniformly organized along an apical-basal plane of polarity. However, in addition, cells in most tissues require positional information in the plane perpendicular to the apical-basal axis. This type of polarization, referred to as planar cell polarity, is critical for morphogenesis of metazoans. A study using cell lineage analysis and close examination of the mitotic axis of dividing cells has shown that lengthening of renal tubules is associated with mitotic orientation of cells along the tubule axis, demonstrating intrinsic planar cell polarity. Dysregulation of oriented cell division can give rise to cysts as a result of abnormal widening of tubule diameters. To date, molecules implicated in planar cell polarity and tubule elongation include HNF1β-PKHD axis, Fat4, and Wnt9b.
Molecular Genetics of the Stromal Cell Lineage
The maintenance of reiterative ureteric branching and concomitant nephron induction largely accounts for the growth and enlargement of embryonic kidneys. Genetic studies reveal that interstitial stroma provides additional inductive cues that regulate UB branching and nephrogenesis (see Figure 1.15 ). These studies also underscore the pivotal role played by the stroma in establishing the stereotypical radial patterning of the kidney. In embryonic kidneys, the stroma is organized into two distinct zones, an outer stromal region within the nephrogenic zone expressing the winged helix transcription factor Foxd1/BF-2, and a deeper region expressing the basic helix-loop-helix transcription factor Tcf21 (Pod1/capsulin/epicardin). Without either Foxd1 or Tcf21, UB branching and nephrogenesis are notably impaired, resulting in a distinctive perturbation of the corticomedullary renal histoarchitecture.
The most prominent features of the genetic loss of Foxd1 include the thickening of the renal capsule and the formation of large metanephric mesenchymal condensates. The morphologically altered renal capsule in Foxd1 mutant kidneys has notably lost expression of Aldh1a2/Raldh2 and Sfrp1 (a regulator of Wnt signaling) and is abnormally interspersed with endothelial cells and Bmp4-positive cells. The identity of these Bmp4-expressing cells populating the renal capsule in Foxd1-deficient kidneys is unknown, although on the basis of lineage tracing for Foxd1-promoter expression, the cells are clearly distinct from the presumptive medullary stroma. Bmp4 is a known chemotactic agent for endothelial cells, so it is very likely that the ectopic Bmp4-positive cells account for the presence of endothelial cells within the broadened renal capsule of Foxd1 mutant kidneys. The accumulation of the cap mesenchyme is also likely contributed in part by ectopic Bmp4 signaling in the absence of Foxd1, because Bmp4 has been shown to antagonize epithelialization of the cap mesenchyme. Transcriptome analysis shows that the gene Dcn, which encodes for the collagen-binding proteoglycan decorin, is a specific target that is repressed by Foxd1 in the cortical interstitium. Dcn expression is normally localized within the medullary stroma but is normally absent in the cortical stroma of wild-type kidneys. In the absence of Foxd1, decorin becomes abundantly expressed in the presumptive cortical stromal region. Functional cell-culture–based assays and epithelialization assays of mesenchymal aggregates demonstrate that decorin inhibits Bmp7 signaling and mesenchyme-to-epithelial transformation. The antagonistic effect of decorin on epithelial differentiation is further enhanced in vitro when the mesenchymal aggregates are grown in collagen IV, thus recapitulating the persistence of the cap mesenchyme as seen in Foxd1 mutant kidneys, in which both decorin and collagen IV are upregulated in the cortical interstitium. These findings are corroborated by the partial rescue of the Foxd1 -null phenotype through genetic inactivation of Dcn .
Tcf21 (also called Pod1) is expressed in the medullary stroma as well as in the condensing MM. Tcf21 is also expressed in a number of differentiated renal cell types that derive from these mesenchymal cells and include developing and mature podocytes of the renal glomerulus, cortical and medullary peritubular interstitial cells, pericytes surrounding small renal vessels, and adventitial cells surrounding larger blood vessels (see Figure 1.6 ). The defect in nephrogenesis observed in Tcf21 -null mice is similar to the defect seen in Foxd1 -knockout mice, consisting of disruption of branching morphogenesis with associated arrest and delay in nephrogenesis. Analysis of chimeric mice derived from Tcf21 mutant embryonic stem cells and EGFP-expressing embryos demonstrated both cell-autonomous and non–cell-autonomous roles for Tcf21 in nephrogenesis. Most strikingly, the glomerulogenesis defect was rescued by the presence of wild-type stromal cells (i.e., mutant cells will epithelialize and form nephrons normally as long as they are surrounded by wild-type stromal cells). In addition, there is a cell-autonomous requirement for Tcf21 in stromal mesenchymal cells to allow differentiation into interstitial and pericyte cell lineages of the cortex and medulla, because Tcf21-null ES cells were unable to contribute to these populations.
Although many of the defects in the Tcf21 mutant kidneys phenocopy those seen in the Foxd1 mutant kidneys, there are important differences. Kidneys from Tcf21 -null mice have vascular anomalies and defective pericyte differentiation that were not reported in Foxd1 mutant mice. These differences might result from the broader domain of Tcf21 expression, which includes the condensing mesenchyme, podocytes, and medullary stromal cells in addition to the stromal cells that surround the condensates. In contrast to Foxd1, Tcf21 is not highly expressed in the thin rim of stromal cells found immediately beneath the capsule, suggesting that Foxd1 and Tcf21 might mark early and late stromal cell lineages, respectively, with overlap in the stroma that surrounds the condensates. However, definitive co-labeling studies to address this issue have not been performed. Both Tcf21 and Foxd1 are transcription factors so it is interesting to speculate that they might interact or regulate the expression of a common stromal “inducing factor.”
Retinoids secreted by the renal stroma are also recognized as important for the maintenance of a high level of Ret receptor expression in the UB tip, promoting the proliferation of UB epithelial cells and the growth of the ureteric tree. One study concluded that the defective UB branching seen in Foxd1 -null mutants is most likely a direct consequence of the loss of cortical expression of Aldh1a2, a gene involved in retinol (vitamin A) synthesis. A later study has shown that renal stroma immediately around the UB tips is also important in regulating the bifurcation of the tips and the creation of new UB branches. Autocrine retinoid signaling in the stromal cells juxtaposed to the UB tips stimulates the expression of extracellular matrix 1 (Ecm1). Ecm1 is specifically expressed at the UB cleft, where it suppresses and restricts Ret expression domains within the UB tips. In the absence of Ecm1, Ret expression in the UB tips broadens, effectively attenuating UB branching through impaired formation of UB bifurcation clefts. Thus, stromal retinoids promote and confine Ret expression domains and, more likely, cell proliferation patterns within the UB tips.
A 2013 study has provided valuable insight into how stroma-based signaling intersects with UB-derived inductive cues to promote proper differentiation of the nephrogenic mesenchyme. When the stromal lineage is selectively annihilated by Foxd1-Cre–driven expression of diphtheria toxin, the zone of condensing mesenchymal cells capping the UBs is abnormally broadened but the development of pretubular aggregates is strongly hindered. These findings reiterate those previously described in Foxd1 -null mice, suggesting that regulation of nephrogenesis involves a crosstalk between stroma and UB-derived inductive signals. In particular, it was shown that Fat4-dependent Hippo signaling initiated by the stroma integrates with canonical Wnt signaling derived from the ureteric lineage in order to balance nephron precursor propagation and differentiation. The absence of Fat4 in the stromal compartment phenocopies the expansion of the nephrogenic precursor domain and failed epithelial differentiation of nephron progenitors seen in stroma-deficient kidneys. It was postulated that Fat4 acting through the Hippo pathway promotes the differentiation of the epithelial transition of nephrogenic precursors. This possibility was further reiterated by the rescue of the depletion of nephrogenic precursors by Fat4 deficiency in Wnt9b -knockout mice. Interestingly, the ablation of Vangl2, a signaling partner of Fat4 known to regulate renal tubular diameter, fails to rescue the loss of nephron progenitors in Wnt9b -knockout animals, suggesting that Fat4-mediated signaling during early differentiation of nephrogenic precursors is independent of the planar cell polarity pathway.
Molecular Genetics of Vascular Formation
Vasculogenesis and angiogenesis both contribute to vascular development within the kidney. Endothelial cells may be identified through the expression of the tyrosine kinase receptor, VEGFR2 (Flk1/KDR). Reporter mouse strains that carry β-galactosidase (lacZ) or GFP cDNA cassettes “knocked into” the Vegfr2 locus permit precise snapshots of vessel development, because all the vascular progenitor and differentiated cells in these organs can be visualized either colorimetrically (with a β-galactosidase substrate) or by fluorescence ( Figure 1.16 ). Use of other knock-in strains allows identification of endothelial cells lining arteriolar or venous vessels.

Over the past decade, a number of growth factors and their receptors have been identified that are required for vasculogenesis and angiogenesis. Gene deletion studies in mice have shown that VEGF-A and its cognate receptor VEGFR2 are essential for vasculogenesis. Mice that are null for the Vegfa gene die at 9.5 dpc from a failure of vasculogenesis, whereas mice lacking a single Vegfa allele (i.e., they are heterozygous for the Vegfa gene) die at 11.5 dpc, also from vascular defects. These data demonstrate gene dosage sensitivity to VEGF-A during development. In the developing kidney, podocytes and renal tubular epithelial cells express VEGF-A and continue to express it constitutively in the adult kidney, whereas the cognate tyrosine kinase receptors for VEGF-A, VEGFR1 (Flt1), and VEGFR2 (Flk1/KDR) are predominantly expressed by all endothelial cells. Which non-endothelial cells might also express the VEGF receptors in the kidney in vivo is still debated, although renal cell lines clearly do and MM cells express VEGFR2 in organ culture as outlined earlier.
Conditional gene targeting experiments and cell-selective deletion of Vegfa from podocytes demonstrated that VEGF-A signaling is required for formation and maintenance of the glomerular filtration barrier. Glomerular endothelial cells express VEGFR2 as they migrate into the vascular cleft. Although a few endothelia migrated into the developing glomeruli of Vegfa podocyte conditional knockout mutants (likely because of a small amount of VEGF-A produced by presumptive podocytes at the S-shaped stage of glomerular development prior to Cre-mediated genetic deletion), the endothelia failed to develop fenestrations and rapidly disappeared, leaving capillary “ghosts” ( Figure 1.17 ). Similar to the dosage sensitivity observed in the whole embryo, deletion of a single Vegfa allele from podocytes also led to glomerular endothelial defects known as endotheliosis that progressed to end-stage kidney failure at 3 months of age. As the dose of VEGF-A decreased, the associated endothelial phenotypes became more severe ( Figure 1.18 ). Upregulation of the major angiogenic VEGF-A isoform (VEGFA 164 ) in developing podocytes of transgenic mice led to massive proteinuria and collapse of the glomerular tuft by 5 days of age. Taken together, these results show a requirement for VEGF-A for development and maintenance of the specialized glomerular endothelia and demonstrate a major paracrine signaling function for VEGF-A in the glomerulus. Furthermore, tight regulation of the dose of VEGF-A is essential for proper formation of the glomerular capillary system. The molecular basis and mechanism of dosage sensitivity are unclear at present and are particularly intriguing, given the documented inducible regulation of VEGF-A by hypoxia-inducible factors (HIFs) at a transcriptional level. Nevertheless, it is clear that in vivo, a single Vegfa allele is unable to compensate for loss of the other. Immortalized podocyte cell lines express a variety of VEGF receptors, opening up the possibility that VEGF-A also plays an autocrine role in the developing glomerulus. However, the functional relevance of these findings for the glomerulus in vivo is unclear at present.


A second major RTK signaling pathway required for maturation of developing blood vessels is the angiopoietin-Tie signaling system. Angiopoietin 1 (Angpt1) stabilizes newly formed blood vessels and is associated with loss of vessel plasticity and concurrent recruitment of pericytes or vascular support cells to the vascular wall. The molecular switch or pathway leading to vessel maturation through activation of Tie2 (previously known as Tek), the major receptor for Angpt1, is not known and appears to be independent of the PDGF (platelet-derived growth factor) signaling system that is also required for pericyte recruitment. The importance of Angpt1 in promoting the development of the renal microvasculature was first suggested on the basis of observations that exogenous Angpt1 enhanced the growth of interstitial capillaries in mouse metanephric organ cultures. Because Angpt1 -null mice perish embryonically at around 12.5 dpc, the in vivo role of Angpt1 during renal development was gleaned through the use of an inducible knockout strategy. Ablation of Angpt1 at around 10.5 dpc resulted in general dilation of renal blood vessels, including the glomerular capillaries sometimes observed as simplified single enlarged loops. A marked reduction of mesangial cells was also observed in Angpt1 -deficient mutants. Without Angpt1, a few endothelial cells are seen to detach from the GBM, although the GBM itself appears otherwise normal directly beneath the podocytes, suggesting that the endothelium is primarily affected by loss of Angpt1.
In contrast, it is proposed that angiopoietin 2 (Angpt2) functions as a natural antagonist of the Tie2 receptor, because Angpt2 can bind to this receptor but fails to induce Tie2 phosphorylation in endothelial cultures. Consistent with this hypothesis is the fact that overexpression of Angpt2 in transgenic mice resulted in a phenotype similar to the Angpt1 or Tie2 knockout mice. Angpt1, Angpt2, Tie2, and the orphan receptor Tie1 have all been shown to be expressed in the developing kidney. Whereas Angpt1 is quite broadly expressed in condensing mesenchyme, podocytes, and tubular epithelial cells, Angpt2 is more restricted to pericytes and smooth muscle cells surrounding cortical and large vessels as well as in the mesangium. In one study, Angpt2 -null mice were viable but exhibited defects in peritubular cortical capillary development; the mice died prior to differentiation of vasa recta, precluding analysis of the role of Angpt2 in these other capillary beds. Podocyte-specific overexpression of Angpt2 has been found to cause proteinuria and increased apoptosis in glomerular capillaries. Both angiopoietin ligands function in concert with VEGF, although the precise degree of crosstalk between these pathways is still under investigation. VEGF and Angpt2, for example, have been shown to cooperate in promoting endothelial sprouting. Chimeric studies showed that Tie1 is required for the development of the glomerular capillary system because Tie1 -null cells failed to incorporate into the glomerular endothelium.
The Ephrin-Eph family is a third tyrosine kinase–dependent growth factor signaling system that is expressed in the developing kidney; in the whole embryo, it is involved in neural sprouting and axon finding as well as in the specification of arterial and venous components of the vasculature. Ephrins and their cognate receptors are expressed widely during renal development. Overexpression of EphB4 leads to defects in glomerular arteriolar formation, whereas conditional deletion of EphrinB2 from perivascular smooth muscle cells and mesangial cells results in glomerular vascular abnormalities. How this latter effect occurs is not entirely clear because EphrinB2 has a dynamic pattern of expression in the developing glomerulus, beginning in podocyte precursors and rapidly switching to glomerular endothelial cells and mesangial cells.
Dysregulation of BMP within the podocyte compartment also results in glomerular vascular defects. Overexpression of BMP4 leads to defects in endothelial and mesangial recruitment, and overexpression of noggin, a natural BMP2 antagonist, results in collapse of the glomerular tuft. Bmp4 haploinsufficiency, on the other hand, leads to dysplastic kidneys and glomerular cysts with collapsed capillary tufts. Additional studies are required to fully understand the role of this family of growth factors in glomeruli.
An additional pathway that is likely to play a role in glomerular endothelial development and perhaps in development of the entire renal vasculature is the SDF1-CXCR4 axis. CXCR4, a G protein–coupled chemokine receptor, is expressed by bone marrow–derived cells but also by endothelial cells. SDF1 (CXCL12), the only known ligand for CXCR4, is expressed in a dynamic segmental pattern in podocytes and later in the mesangial cells of the glomerulus. Embryonic deletion of either Cxcl12 or Cxcr4 does not preclude nephrogenesis but results in defective formation of blood vessels, notably an abnormal patterning of the renal vasculature and the development of a simplified and dilated glomerular capillary tuft. Interestingly, genetic loss of CXCR7, which is thought to act as a decoy receptor for SDF1, phenocopies defective development seen in Cxcl12 and Cxcr4 mutant mice. Unlike CXCR4, CXCR7 is specifically expressed by podocytes and not endothelial cells. It has been proposed that CXCR7, acting as a scavenger receptor, establishes an SDF1 morphogen gradient, preventing feedback inhibition of CXCR4 receptor expression in target cells such as the endothelium. 306 Consistent with this proposal, inactivation of Cxcr7 distinctively causes downregulation of CXCR4 expression in the renal cap mesenchyme and the glomerular tuft. Thus, the spatial regulation of SDF1-CXCR4 signaling appears to be important for normal development of the glomerular vasculature.
The T-box transcription factor Tbx18 is strongly expressed during early urogenital tract development in the ureteral mesenchyme and in a subset of kidney stromal mesenchyme originating from Foxd1-positive precursor cells. Later in renal development, Tbx18 expression is also found in the renal capsule, vascular smooth muscle cells, pericytes, mesangial cells, and the mesenchyme surrounding the renal papillae and calyces. The most overt phenotype of Tbx18 inactivation is the onset of hydronephrosis and hydroureter due to impaired development of the ureteral smooth muscle cells. This finding underscores the importance of Tbx18 in the normal differentiation of the ureteral mesenchyme. A detailed phenotypic characterization of Tbx18 -null mutant kidneys reveals an additional significance of this transcription factor in the overall development of the renal vasculature. The branching and overall density of the renal vasculature are notably reduced in the absence of Tbx18. Tbx18 is also specifically required in the normal development of the glomerular microvasculature. Loss of Tbx18 causes significant oligonephronia and dilation of glomerular capillaries. These vascular phenotypes likely result from the degeneration of the vascular mesenchyme and the failure to sustain the proliferation of mesangial precursors.
Mice carrying a hypomorphic allele of Notch2 that is missing two epidermal growth factor (EGF) motifs are born with a reduced number of glomeruli that also lack both endothelial and mesangial cells, as discussed in the section on nephron segmentation. The transcription factor Rbpj, a common downstream target of Notch signaling pathways, has also been described as crucial to the proper development of the renal vasculature and the glomerular mesangium. Conditional inactivation of Rbpj in the Foxd1-expressing stromal lineage leads to profound renal maldevelopment and early postnatal death. Rbpj deficiency in the renal stroma results in poor branching and simplification of the renal vascular network. Rbpj conditional mutant kidneys have a greater proportion of larger vessels and a concomitant reduction in microvascular density. Glomeruli are dilated and lack mesangial cells in Rbpj conditional knockout animal. Furthermore, loss of Rbpj results in loss of renin cells, abnormal thickening of blood vessels, and renal fibrosis. Altogether, these studies highlight the distinctive significance of Notch signaling in the establishment and organization of the renal vasculature.
Two transcription factors belonging to the large Sry-related HMG box (Sox) gene family, named Sox17 and Sox18, have distinctive and overlapping expressions in vascular endothelial cells. Complete loss of Sox17 is embryonically lethal in mice owing to endodermal dysmorphogenesis. In mice, Sox18 ablation results in a mild coat defect but does not cause cardiovascular abnormalities. Nevertheless, a point mutation in SOX18 in humans has been implicated in HLT (hypotrichosis-lymphedema-telangiectasia) syndrome, which affects hair, lymph, and blood vessel vasculature. The more severe consequence of the human SOX18 mutation, in comparison with the null mutation in mice, has been suggested to be due to a dominant-negative effect. Sox17 , however, shows haploinsufficiency in a homozygous Sox18 mutant background, affecting neovascularization in kidneys, liver, and the reproductive system and causing early postnatal death. Kidneys from Sox17 (−/+) : Sox18 (−/−) mice have hypoplastic and atrophying medullary regions. In these compound Sox17/Sox18 mutants, the radiating outer medullary vascular bundles of the vasa recta are completely absent without apparent abnormalities in the inner medullary or cortical regions. These defects within the outer medullary region result in variable degrees of hydronephrosis. In livers from these mice, Angpt2 expression is elevated whereas Angpt1 expression is significantly attenuated, although Tie2 receptor expression in unchanged. Because Angpt1 and Angpt2 have distinctive roles in endothelial sprouting, vascular stabilization, and remodeling, it can be argued that Sox17 and Sox18 play redundant or synergistic roles in coordinating normal postnatal angiogenesis.
There is evidence from other model systems that vascular development is required for patterning and terminal differentiation of adjacent tissues. For example, vascular signals and basement membrane produced by adjacent endothelial cells are required for differentiation of the islet cells of the pancreas. In the kidney, it is possible that vascular signals are required for branching morphogenesis and patterning of the nephron and that this requirement may explain some of the defects observed in mice such as the Tcf21 mutants. Understanding co-patterning between the vasculature and its immediate neighbors will be a challenging task, given the complex nature of various tissue-tissue communications involved, although progress in this effort will likely be facilitated by a growing arsenal of genetic tools.
The Juxtaglomerular Apparatus and the Renin Angiotensin Aldosterone System
The juxtaglomerular apparatus consists of juxtaglomerular cells that line the afferent arteriole, the macula densa cells of the distal tubule, and the extraglomerular mesangial cells that are in contact with intraglomerular mesangium. Renin-expressing cells may be seen in arterioles in early mesonephric kidneys in 5-week human fetuses and in metanephric kidneys by week 8, at a stage prior to hemodynamic flow changes within the kidney. Gomez and colleagues generated a Ren1 –knock-in mouse that expresses Cre recombinase in the renin locus. Offspring of matings between the Ren1-Cre and a reporter strain that expresses β-galactosidase or GFP showed that renin-expressing cells reside within the MM and give rise not only to juxtaglomerular cells but also to mesangial cells, epithelial cells, and extrarenal cells, including interstitial Leydig cells of the XY gonad and cells within the adrenal gland. Although most of these cells cease to express renin in the adult, they appear to reexpress renin in stress conditions and are recruited to the afferent arteriole.
The only known substrate for renin, angiotensinogen, is converted to angiotensin I and angiotensin II by ACE. The renin angiotensin aldosterone axis is required for normal renal development. In humans, the use of ACE inhibitors during pregnancy has been associated with congenital defects including renal anomalies. Two subtypes of angiotensin receptors exist: AT1 receptors are responsible for most of the classically recognized functions of the RAAS including pressor effects and aldosterone release mediated through angiotensin; functions of the AT2 receptors have been more difficult to characterize but generally seem to oppose the actions of the AT1 receptors. Genetic deletion of angiotensinogen or ACE results in hypotension and defects in formation of the renal papilla and pelvis. Humans have one AT1 gene whereas mice have two, Agtr1a and Agtr1b . Mice carrying a knockout for either AT1 receptor alone exhibit no major defects, but the combined deficiency phenocopies the angiotensinogen and ACE phenotypes. Although AT2 receptor expression is markedly upregulated in the embryonic kidney, genetic deletion of the AT2 receptor does not cause major impairment of renal development. However, an association between AT2 receptor deficiency and malformations of the collecting system, including vesicoureteral reflux and ureteropelvic junction obstruction, has been reported.
MiRNAs are regulatory RNAs that act as antisense posttranscriptional repressors by binding the 3′ untranslated region of target mRNAs. In eukaryotes, hundreds of miRNAs regulate the expression of thousands of mRNAs, thus implicating miRNAs in a myriad of events during development and disease including cell proliferation, differentiation, apoptosis, signaling and tumorigenicity. Dicer1 is an endoribonuclease that processes precursor miRNAs. Deletion of Dicer1 from renin-expressing cells results in severe reduction in the number of juxtaglomerular cells, reduced renin production, and lower blood pressure. The kidney develops severe vascular abnormalities and striped fibrosis along the affected blood vessels, suggesting that miRNAs are required for normal morphogenesis and function of the kidney.
Gene promoter analysis indicates that renin expression depends on the Notch signaling pathway. The intracellular domain of Notch (NIC) and the transcription factor Rbpj bind and cooperatively stimulate reporter gene expression from the renin promoter. Genetic studies, however, indicate that Notch signaling has a broader role in the juxtaglomerular apparatus. The conditional ablation of Rbpj in renin cells results in severe paucity of juxtaglomerular cells with consequential decrease in overall renin expression and the development of lower blood pressure. Lack of increase in apoptosis in Rbpj conditional mutant kidneys suggests that Rbpj may have altered the cell fate specification of renin cell precursors.
Nephron Development and Glomerulogenesis
Mesangial Cell Ingrowth
Mesangial cells grow into the developing glomerulus and come to sit between the capillary loops. Gene deletion studies have demonstrated a critical role for PDGF-B/PDGFR-β signaling in this process. The absence of PDGF-B, which is expressed by glomerular endothelia, or of the receptor PDGFR-β, which is expressed by mesangial cells, results in glomeruli with single balloon-like capillary loops, instead of the intricately folded glomerular endothelial capillaries of wild-type kidneys. Furthermore, the glomeruli contain no mesangial cells. Endothelial cell–specific deletion of Pdgfb results in the same glomerular phenotype and shows that production of PDGF-B by the endothelium is required for mesangial migration. In turn, mesangial cells and the matrix they produce are required to pattern the glomerular capillary system. Loss of podocyte-derived factors such as VEGF-A also leads to failure of mesangial cell ingrowth, likely through primary loss of endothelial cells and failure of PDGF-B signaling.
A number of other knockouts demonstrate defects in both vascular development and mesangial cell ingrowth. Loss of the transcription factors Tcf21 and Foxc2 causes defective migration of mesangial cells ( Figure 1.19 ). Mesangial abnormalities in Tcf21 – and Foxc2 -deficient mice are poorly understood in terms of the mesangium-specific transcriptional targets of Tcf21 and Foxc2. Nevertheless, these mutant phenotypes highlight the importance of crosstalk between cell compartments within the glomerulus.
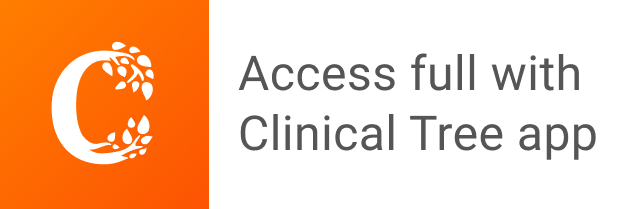