Chapter Outline
BODY FLUIDS: COMPARTMENTALIZATION, COMPOSITION, AND TURNOVER, 460
WATER METABOLISM, 462
Vasopressin Synthesis and Secretion, 462
Thirst, 471
Integration of Vasopressin Secretion and Thirst, 472
DISORDERS OF INSUFFICIENT VASOPRESSIN OR VASOPRESSIN EFFECT, 473
Central Diabetes Insipidus, 473
Osmoreceptor Dysfunction, 477
Gestational Diabetes Insipidus, 480
Nephrogenic Diabetes Insipidus, 480
Primary Polydipsia, 482
Clinical Manifestations of Diabetes Insipidus, 483
Differential Diagnosis of Polyuria, 483
Treatment of Diabetes Insipidus, 486
DISORDERS OF EXCESS VASOPRESSIN OR VASOPRESSIN EFFECT, 490
Relationship Between Hypo-Osmolality and Hyponatremia, 490
Variables That Influence Renal Water Excretion, 491
PATHOGENESIS AND CAUSES OF HYPONATREMIA, 491
Hyponatremia with Extracellular Fluid Volume Depletion, 492
Hyponatremia with Excess Extracellular Fluid Volume, 493
Hyponatremia with Normal Extracellular Fluid Volume, 495
Syndrome of Inappropriate Antidiuretic Hormone Secretion, 497
HYPONATREMIA SYMPTOMS, MORBIDITY, AND MORTALITY, 500
HYPONATREMIA TREATMENT, 503
Current Therapies, 503
Treatment Guidelines, 506
Monitoring Serum Sodium Concentration in Hyponatremic Patients, 508
Long-Term Treatment of Chronic Hyponatremia, 508
Future of Hyponatremia Treatment, 508
Disorders of body fluids are among the most commonly encountered problems in clinical medicine. This is in large part because many different disease states can potentially disrupt the finely balanced mechanisms that control the intake and output of water and solute. Because body water is the primary determinant of the osmolality of the extracellular fluid, disorders of water metabolism can be broadly divided into hyperosmolar disorders, in which there is a deficiency of body water relative to body solute, and hypo-osmolar disorders, in which there is an excess of body water relative to body solute. Because sodium is the main constituent of plasma osmolality, these disorders are typically characterized by hypernatremia and hyponatremia, respectively. Before discussing specific aspects of these disorders, this chapter will first review the regulatory mechanisms underlying water metabolism, which, in concert with sodium metabolism, maintains body fluid homeostasis.
Body Fluids: Compartmentalization, Composition, and Turnover
Water constitutes approximately 55% to 65% of body weight, varying with age, gender, and amount of body fat, and therefore constitutes the largest single constituent of the body. Total body water (TBW) is distributed between the intracellular fluid (ICF) and extracellular fluid (ECF) compartments. Estimates of the relative sizes of these two pools differ significantly, depending on the tracer used to measure the ECF volume, but most studies in animals and humans have indicated that 55% to 65% of TBW resides in the ICF and 35% to 45% is in the ECF. Approximately 75% of the ECF compartment is interstitial fluid and only 25% is intravascular fluid (blood volume). Figure 16.1 summarizes the estimated body fluid spaces of an average weight adult.

The solute composition of the ICF and ECF differs considerably because most cell membranes possess multiple transport systems that actively accumulate or expel specific solutes. Thus, membrane-bound Na + -K + -ATPase maintains Na + in a primarily extracellular location and K + in a primarily intracellular location. Similar transporters effectively result in confining Cl − largely to the ECF, and Mg 2+ , organic acids, and phosphates to the ICF. Glucose, which requires an insulin-activated transport system to enter most cells, is present in significant amounts only in the ECF because it is rapidly converted intracellularly to glycogen or metabolites. HCO 3 − is present in both compartments but is approximately three times more concentrated in the ECF. Urea is unique among the major naturally occurring solutes in that it diffuses freely across most cell membranes ; therefore, it is present in similar concentrations in almost all body fluids, except in the renal medulla, where it is concentrated by urea transporters (see Chapter 10 ).
Despite very different solute compositions, both the ICF and ECF have an equivalent osmotic pressure, which is a function of the total concentration of all solutes in a fluid compartment, because most biologic membranes are semipermeable (i.e., freely permeable to water but not to aqueous solutes). Thus, water will flow across membranes into a compartment with a higher solute concentration until a steady state is reached and the osmotic pressures have equalized on both sides of the cell membrane. An important consequence of this thermodynamic law is that the volume of distribution of body Na + and K + is actually the TBW rather than just the ECF or ICF volume, respectively. For example, any increase in ECF sodium concentration ([Na + ]) will cause water to shift from the ICF to ECF until the ICF and ECF osmotic pressures are equal, thereby effectively distributing the Na + across extracellular and intracellular water.
Osmolality is defined as the concentration of all of the solutes in a given weight of fluid. The total solute concentration of a fluid can be determined and expressed in several different ways. The most common method is to measure its freezing point or vapor pressure because these are colligative properties of the number of free solute particles in a volume of fluid. The result is expressed relative to a standard solution of known concentration using units of osmolality (milliosmoles of solute per kilogram of water, mOsm/kg H 2 O), or osmolarity (milliosmoles of solute per liter of water, mOsm/L H 2 O). Plasma osmolality (P osm ) can be measured directly, as described above, or calculated by summing the concentrations of the major solutes present in the plasma:
P osm ( mOsm / kg H 2 O ) = 2 × plasma [ Na + ] ( mEq / L ) + glucose ( mg / dL ) / 18 + BUN ( mg / dL ) / 2.8
Both methods produce comparable results under most conditions (the value obtained using this formula is generally within 1% to 2% of that obtained by direct osmometry), as will simply doubling the plasma [Na + ], because sodium and its accompanying anions are the predominant solutes present in plasma. However, the total osmolality of plasma is not always equivalent to the effective osmolality, often referred to as the tonicity of the plasma, because the latter is a function of the relative solute permeability properties of the membranes separating the two compartments. Solutes that are impermeable to cell membranes (e.g., Na + , mannitol) are restricted to the ECF compartment. They are effective solutes because they create osmotic pressure gradients across cell membranes, leading to the osmotic movement of water from the ICF to ECF compartments. Solutes that are permeable to cell membranes (e.g., urea, ethanol, methanol) are ineffective solutes because they do not create osmotic pressure gradients across cell membranes and therefore are not associated with such water shifts. Glucose is a unique solute because, at normal physiologic plasma concentrations, it is taken up by cells via active transport mechanisms and therefore acts as an ineffective solute but, under conditions of impaired cellular uptake (e.g., insulin deficiency), it becomes an effective extracellular solute.
The importance of this distinction between total and effective osmolality is that only the effective solutes in plasma are determinants of whether clinically significant hyperosmolality or hypo-osmolality is present. An example of this is uremia; a patient with a blood urea nitrogen (BUN) concentration that has increased by 56 mg/dL will have a corresponding 20-mOsm/kg H 2 O elevation in plasma osmolality, but the effective osmolality will remain normal because the increased urea is proportionally distributed across the ECF and ICF. In contrast, a patient whose plasma [Na + ] has increased by 10 mEq/L will also have a 20-mOsm/kg H 2 O elevation of plasma osmolality because the increased cation must be balanced by an equivalent increase in plasma anions. However, in this case the effective osmolality will also be elevated by 20 mOsm/kg H 2 O because the Na + and accompanying anions will largely remain restricted to the ECF due to the relative impermeability of cell membranes to Na + and other ions. Thus, elevations of solutes such as urea, unlike elevations of sodium, do not cause cellular dehydration and consequently do not activate mechanisms that defend body fluid homeostasis by increasing body water stores.
Both body water and solutes are in a state of continuous exchange with the environment. The magnitude of the turnover varies considerably, depending on physical, social, and environmental factors, but in healthy adults it averages 5% to 10% of the total body content each day. For the most part, daily intake of water and electrolytes is not determined by physiologic requirements but is more a function of dietary preferences and cultural influences. Healthy adults have an average daily fluid ingestion of approximately 2 to 3 L, but with considerable individual variation; approximately one third of this is derived from food or the metabolism of fat and the rest from discretionary ingestion of fluids. Similarly, of the 1000 mOsm of solute ingested or generated by the metabolism of nutrients each day, nearly 40% is intrinsic to food, another 35% is added to food as a preservative or flavoring, and the rest is mostly urea. In contrast to the largely unregulated nature of basal intakes, the urinary excretion of water and solute is highly regulated to preserve body fluid homeostasis. Thus, under normal circumstances, almost all ingested Na + , Cl − , and K + , as well as ingested and metabolically generated urea, are excreted in the urine under the control of specific regulatory mechanisms. Other ingested solutes, such as divalent minerals, are excreted primarily by the gastrointestinal tract. Urinary excretion of water is also tightly regulated by the secretion and renal effects of arginine vasopressin (AVP; vasopressin, antidiuretic hormone), discussed in greater detail in Chapters 10 and 11 and in the following section (“Water Metabolism”).
Water Metabolism
Water metabolism is responsible for the balance between the intake and excretion of water. Each side of this balance equation can be considered to consist of a regulated and unregulated component, the magnitudes of which can vary markedly under different physiologic and pathophysiologic conditions. The unregulated component of water intake consists of the intrinsic water content of ingested foods, consumption of beverages primarily for reasons of palatability or desired secondary effects (e.g., caffeine), or for social or habitual reasons (e.g., alcoholic beverages), whereas the regulated component of water intake consists of fluids consumed in response to a perceived sensation of thirst. Studies of middle-aged subjects have shown mean fluid intakes of 2.1 L/24 hours, and analyses of the fluids consumed have indicated that the vast majority of the fluid ingested is determined by influences such as meal-associated fluid intake, taste, or psychosocial factors rather than true thirst.
The unregulated component of water excretion occurs via insensible water losses from a variety of sources (e.g., cutaneous losses from sweating, evaporative losses in exhaled air, gastrointestinal losses) as well as the obligate amount of water that the kidneys must excrete to eliminate solutes generated by body metabolism, whereas the regulated component of water excretion is comprised of the renal excretion of free water in excess of the obligate amount necessary to excrete metabolic solutes. Unlike solutes, a relatively large proportion of body water is excreted by evaporation from skin and lungs. This amount varies markedly, depending on several factors, including dress, humidity, temperature, and exercise. Under the sedentary and temperature-controlled indoor conditions typical of modern urban life, daily insensible water loss in healthy adults is minimal, approximately 8 to 10 mL/kg body weight (BW; ≈0.5 to 0.7 L in a 70-kg adult man or woman). However, insensible losses can increase to twice this level (20 mL/kg BW) simply under conditions of increased activity and temperature and, if environmental temperature or activity is even higher, such as in an arid environment, the rate of insensible water loss can even approximate the maximal rate of free water excretion by the kidney. Thus, in quantitative terms, insensible loss and the factors that influence it can be just as important to body fluid homeostasis as regulated urine output.
Another major determinant of unregulated water loss is the rate of urine solute excretion, which cannot be reduced below a minimal obligatory level required to excrete the solute load. The volume of urine required depends not only on the solute load, but also on the degree of antidiuresis. At a typical basal level of urinary concentration (urine osmolality = 600 mOsm/kg H 2 O) and a typical solute load of 900 to 1200 mOsm/day, a 70-kg adult would require a total urine volume of 1.5 to 2.0 L (21 to 29 mL/kg BW) to excrete the solute load. However, under conditions of maximal antidiuresis (urine osmolality = 1200 mOsm/kg H 2 O), the same solute load would require a minimal obligatory urine output of only 0.75 to 1.0 L/day and, conversely, a decrease in urine concentration to minimal levels (urine osmolality = 60 mOsm/kg H 2 O) would obligate a proportionately larger urine volume of 15 to 20 L/day to excrete the same solute load.
The above discussion emphasizes that water intake and water excretion have very substantial unregulated components, and these can vary tremendously as a result of factors unrelated to the maintenance of body fluid homeostasis. In effect, the regulated components of water metabolism are those that act to maintain body fluid homeostasis by compensating for whatever perturbations result from unregulated water losses or gains. Within this framework, the major mechanisms responsible for regulating water metabolism are pituitary secretion and the renal effects of vasopressin and thirst, each of which will be discussed in greater detail in the following sections.
Vasopressin Synthesis and Secretion
The primary determinant of free water excretion in animals and humans is the regulation of urinary water excretion by circulating levels of AVP in plasma. The renal effects of AVP are covered extensively in Chapters 10 and 11 . This chapter will focus on the regulation of AVP synthesis and secretion.
Structure and Synthesis
Before AVP was biochemically characterized, early studies used the general term antidiuretic hormone (ADH) to describe this substance. Now that AVP is known to be the only naturally occurring antidiuretic substance, it is more appropriate to refer to it by its correct hormonal designation. AVP is a nine–amino acid peptide that is synthesized in the hypothalamus. It is composed of a six–amino acid, ringlike structure formed by a disulfide bridge, with a three–amino acid tail, at the end of which the terminal carboxyl group is amidated. Substitution of lysine for arginine in position 8 yields lysine vasopressin, the antidiuretic hormone found in pigs and other members of the suborder Suina. Substitution of isoleucine for phenylalanine at position 3 and of leucine for arginine at position 8 yields oxytocin (OT), a hormone found in all mammals and in many submammalian species. OT has weak antidiuretic activity but is a potent constrictor of smooth muscle in mammary glands and uterus. As implied by their names, arginine and lysine vasopressin also cause constriction of blood vessels, which was the property that lead to their original discovery in the late nineteenth century, but this pressor effect occurs only at concentrations many times higher than those required to produce antidiuresis. This is probably of little physiologic or pathologic importance in humans except under conditions of severe hypotension and hypovolemia, where it acts to supplement the vasoconstrictive actions of angiotensin II (Ang II) and the sympathetic nervous system. The multiple actions of AVP are mediated by different G protein–coupled receptors, designated V 1a , V 1b , and V 2 (see Chapter 11 ).
AVP and OT are produced by the neurohypophysis, often referred to as the posterior pituitary gland, because the neural lobe is located centrally and posterior to the adenohypophysis, or anterior pituitary gland, in the sella turcica. However, it is important to understand that the posterior pituitary gland consists only of the distal axons of the magnocellular neurons that comprise the neurohypophysis. The cell bodies of these axons are located in specialized (magnocellular) neural cells located in two discrete areas of the hypothalamus, the paired supraoptic nuclei (SON) and paraventricular nuclei (PVN; Figure 16.2 ). In adults, the posterior pituitary is connected to the brain by a short stalk through the diaphragm sellae. The neurohypophysis is supplied with blood by branches of the superior and inferior hypophysial arteries, which arise from the posterior communicating and intracavernous portion of the internal carotid artery. In the posterior pituitary, the arterioles break up into localized capillary networks that drain directly into the jugular vein via the sellar, cavernous, and lateral venous sinuses. Many of the neurosecretory neurons that terminate higher in the infundibulum and median eminence originate in parvicellular neurons in the PVN; they are functionally distinct from the magnocellular neurons that terminate in the posterior pituitary because they primarily enhance secretion of adrenocorticotropic hormone (ACTH) from the anterior pituitary. AVP-containing neurons also project from parvicellular neurons of the PVN to other areas of the brain, including the limbic system, nucleus tractus solitarius, and lateral gray matter of the spinal cord. The full extent of the functions of these extrahypophysial projections are still under study.

The genes encoding the AVP and OT precursors are located in close proximity on chromosome 20, but are expressed in mutually exclusive populations of neurohypophyseal neurons. The AVP gene consists of approximately 2000 base pairs and contains three exons separated by two intervening sequences ( Figure 16.3 ). Each exon encodes one of the three functional domains of the preprohormone, although small parts of the nonconserved sequences of neurophysin are located in the first and third exons that code for AVP and the C-terminal glycoprotein, called copeptin, respectively. The untranslated 5′-flanking region, which regulates expression of the gene, shows extensive sequence homology across several species but is markedly different from the otherwise closely related gene for OT. This promoter region of the AVP gene in the rat contains several putative regulatory elements, including a glucocorticoid response element, cyclic adenosine monophosphate (cAMP) response element, and four activating protein-2 (AP-2) binding sites. Experimental studies have suggested that the DNA sequences between the AVP and OT genes, the intergenic region, may contain critical sites for cell-specific expression of these two hormones.

The gene for AVP is also expressed in a number of other neurons, including but not limited to the parvicellular neurons of the PVN and SON. AVP and OT genes are also expressed in several peripheral tissues, including the adrenal medulla, ovary, testis, thymus, and certain sensory ganglia. However, the AVP mRNA in these tissues appears to be shorter (620 bases) than its hypothalamic counterpart (720 bases), apparently because of tissue-specific differences in the length of the polyA tails. More importantly, the levels of AVP in peripheral tissues are generally two or three orders of magnitude lower than in the neurohypophysis, suggesting that AVP in these tissues likely has paracrine rather than endocrine functions. This is consistent with the observation that destruction of the neurohypophysis essentially eliminates AVP from the plasma, despite the presence of these multiple peripheral sites of AVP synthesis.
Secretion of AVP and its associated neurophysin and copeptin peptide fragments occurs by a calcium-dependent exocytotic process, similar to that described for other neurosecretory systems. Secretion is triggered by propagation of an electrical impulse along the axon that causes depolarization of the cell membrane, an influx of Ca 2+ , fusion of secretory granules with the cell membrane, and extrusion of their contents. This view is supported by the observation that AVP, neurophysin, and the glycoprotein copeptin are released simultaneously by many stimuli. However, at the physiologic pH of plasma, there is no binding of AVP or OT to their respective neurophysins so, after secretion, each peptide circulates independently in the bloodstream.
Stimuli for secretion of AVP or OT also stimulate transcription and increase the mRNA content of both prohormones in the magnocellular neurons. This has been well documented in rats, in which dehydration, which stimulates secretion of AVP, accelerates transcription and increases the levels of AVP (and OT) mRNA, and hypo-osmolality, which inhibits the secretion of AVP, produces a decrease in the content of AVP mRNA. These and other studies have indicated that the major control of AVP synthesis most likely resides at the level of transcription.
Antidiuresis occurs via interaction of the circulating hormone with AVP V 2 receptors in the kidney, which results in increased water permeability of the collecting duct through the insertion of the aquaporin-2 (AQP2) water channel into the apical membranes of collecting tubule principal cells (see Chapter 10 ). The importance of AVP for maintaining water balance is underscored by the fact that the normal pituitary stores of this hormone are very large, allowing more than 1 week’s supply of hormone for maximal antidiuresis under conditions of sustained dehydration. Knowledge of the different conditions that stimulate pituitary AVP release in humans is therefore essential for understanding water metabolism.
Osmotic Regulation
AVP secretion is influenced by many different stimuli, but since the pioneering studies of ADH secretion by Ernest Basil Verney, it has been clear that the most important stimulus under physiologic conditions is the osmotic pressure of plasma. With further refinement of radioimmunoassays for AVP, the unique sensitivity of this hormone to small changes in osmolality, as well as the corresponding sensitivity of the kidney to small changes in plasma AVP levels, have become apparent. Although the magnocellular neurons themselves have been found to have intrinsic osmoreceptive properties, research over the last several decades has clearly shown that the most sensitive osmoreceptive cells that can sense small changes in plasma osmolality and transduce these changes into AVP secretion are located in the anterior hypothalamus, likely in or near the circumventricular organ termed the organum vasculosum of the lamina terminalis (OVLT; see Figure 16.2 ). Perhaps the strongest evidence for location of the primary osmoreceptors in this area of the brain are the multiple studies that have demonstrated that destruction of this area disrupts osmotically stimulated AVP secretion and thirst, without affecting the neurohypophysis or its response to nonosmotic stimuli.
Although some debate still exists with regard to the exact pattern of osmotically stimulated AVP secretion, most studies to date have supported the concept of a discrete osmotic threshold for AVP secretion, above which there is a linear relationship between plasma osmolality and AVP levels ( Figure 16.4 ). At plasma osmolalities below a threshold level, AVP secretion is suppressed to low or undetectable levels; above this point, AVP secretion increases linearly in direct proportion to plasma osmolality. The slope of the regression line relating AVP secretion to plasma osmolality can vary significantly across individual human subjects, in part because of genetic factors, but also in relation to other factors. In general, each 1-mOsm/kg H 2 O increase in plasma osmolality causes an increase in the plasma AVP level, ranging from 0.4 to 1.0 pg/mL. The renal response to circulating AVP is similarly linear, with urinary concentration that is directly proportional to AVP levels from 0.5 to 4 to 5 pg/mL, after which urinary osmolality is maximal and cannot increase further, despite additional increases in AVP levels ( Figure 16.5 ). Thus, changes of as little as 1% in plasma osmolality are sufficient to cause significant increases in plasma AVP levels, with proportional increases in urine concentration, and maximal antidiuresis is achieved after increases in plasma osmolality of only 5 to 10 mOsm/kg H 2 O (2% to 4%) above the threshold for AVP secretion.


However, even this analysis underestimates the sensitivity of this system to regulate free water excretion. Urinary osmolality is directly proportional to plasma AVP levels as a consequence of the fall in urine flow induced by the AVP, but urine volume is inversely related to urine osmolality (see Figure 16.5 ). An increase in plasma AVP concentration from 0.5 to 2 pg/mL has a much greater relative effect to decrease urine flow than a subsequent increase in AVP concentration from 2 to 5 pg/mL, thereby magnifying the physiologic effects of small changes in lower plasma AVP levels. Furthermore, the rapid response of AVP secretion to changes in plasma osmolality, coupled with the short half-life of AVP in human plasma (10 to 20 minutes), allows the kidneys to respond to changes in plasma osmolality on a minute-to-minute basis. The net result is a finely tuned osmoregulatory system that adjusts the rate of free water excretion accurately to the ambient plasma osmolality, primarily via changes in pituitary AVP secretion.
The set point of the osmoregulatory system also varies from person to person. In healthy adults, the osmotic threshold for AVP secretion ranges from 275 to 290 mOsm/kg H 2 O (averaging ≈280 to 285 mOsm/kg H 2 O). Similar to sensitivity, individual differences in the set point of the osmoregulatory system are relatively constant over time and appear to be genetically determined. However, multiple factors can alter the sensitivity and/or set point of the osmoregulatory system for AVP secretion, in addition to genetic influences. Foremost among these are acute changes in blood pressure, effective blood volume, or both (discussed in the following section). Aging has been found to increase the sensitivity of the osmoregulatory system in multiple studies. Metabolic factors, such as serum Ca 2+ levels and various drugs, can alter the slope of the plasma AVP-osmolality relationship as well. Lesser degrees of shifting of the osmosensitivity and set point for AVP secretion have been noted with alterations in gonadal hormones. Some studies have found increased osmosensitivity in women, particularly during the luteal phase of the menstrual cycle, and in estrogen-treated men, but these effects were relatively minor, and others have found no significant gender differences. The set point of the osmoregulatory system is reduced more dramatically and reproducibly during pregnancy. Evidence has suggested the possible involvement of the placental hormone relaxin, rather than gonadal steroids or human chorionic gonadotropin hormone in pregnancy-associated resetting of the osmostat for AVP secretion. Both the changes in volume and in osmolality have been reproduced by infusion of relaxin into virgin female and normal rats and reversed in pregnant rats by immunoneutralization of relaxin. Increased nitric oxide (NO) production by relaxin has been reported to increase vasodilatation, and estrogens also increase NO synthesis. That multiple factors can influence the set point and sensitivity of osmotically regulated AVP secretion is not surprising because AVP secretion reflects a balance of bimodal inputs—inhibitory and stimulatory —from multiple different afferent inputs to the neurohypophysis ( Figure 16.6 ).

Understanding the osmoregulatory mechanism also requires addressing the observation that AVP secretion is not equally sensitive to all plasma solutes. Sodium and its anions, which normally contribute more than 95% of the osmotic pressure of plasma, are the most potent solutes in terms of their capacity to stimulate AVP secretion and thirst, although certain sugars such as mannitol and sucrose are also equally effective when infused intravenously. In contrast, increases in plasma osmolality caused by noneffective solutes such as urea or glucose result in little or no increase in plasma AVP levels in humans or animals. These differences in response to various plasma solutes are independent of any recognized nonosmotic influence, indicating that they are a property of the osmoregulatory mechanism itself. According to current concepts, the osmoreceptor neuron is stimulated by osmotically induced changes in its water content. In this case, the stimulatory potency of any given solute would be an inverse function of the rate at which it moves from the plasma to the inside of the osmoreceptor neuron. Solutes that penetrate slowly, or not at all, create an osmotic gradient that causes an efflux of water from the osmoreceptor, and the resultant shrinkage of the osmoreceptor neuron activates a stretch-inactivated, noncationic channel that initiates depolarization and firing of the neuron. Conversely, solutes that penetrate the cell readily create no gradient and thus have no effect on the water content and cell volume of the osmoreceptors. This mechanism agrees well with the observed relationship between the effect of certain solutes on AVP secretion, such as Na + , mannitol, and glucose, and the rate at which they penetrate the blood-brain barrier.
Many neurotransmitters have been implicated in mediating the actions of the osmoreceptors on the neurohypophysis. The supraoptic nucleus is richly innervated by multiple pathways, including acetylcholine, catecholamines, glutamate, γ-aminobutyric acid (GABA), histamine, opioids, Ang II, and dopamine. Studies have supported a potential role for all of these and others in the regulation of AVP secretion, as has local secretion of AVP into the hypothalamus from dendrites of the AVP-secreting neurons. Although it remains unclear which of these are involved in the normal physiologic control of AVP secretion, in view of the likelihood that the osmoregulatory system is bimodal and integrated with multiple different afferent pathways (see Figure 16.6 ), it seems likely that magnocellular AVP neurons are influenced by a very complex mixture of neurotransmitter systems, rather than only a few.
Exactly how cells sense volume changes is a critical step for all the mechanisms activated to achieve osmoregulation. Some of the most exciting new data have come from studies of brain osmoreceptors. The cellular osmosensing mechanism used by the OVLT cells is an intrinsic depolarizing receptor potential. This potential is generated in these cells via a molecular transduction complex. Studies have suggested that this likely includes members of the transient receptor potential vanilloid (TRPV) family of cation channel proteins. These channels are generally activated by cell membrane stretch to cause a nonselective conductance of cations, with a preference for Ca 2+ . Multiple studies have characterized various members of the TRPV family as cellular mechanoreceptors in different tissues. Both in vitro and in vivo studies of the TRPV family of cation channel proteins has provided evidence supporting the roles of TRPV1, TRPV2, and TRPV4 proteins in the transduction of osmotic stimuli in mammals that are important for sensing cell volume. Moreover, genetic variation in the TRPV4 gene affects TRPV4 function and may influence water balance on a population-wide basis. The details of exactly how and where various members of the TRPV family of cation channel proteins participate in osmoregulation in different species remains to be ascertained by additional studies. However, a strong case can already be made for their involvement in the transduction of osmotic stimuli in the neural cells in the OVLT and surrounding hypothalamus that regulate osmotic homeostasis, which appears to have been highly conserved throughout evolution.
Nonosmotic Regulation
Hemodynamic Stimuli
Not surprisingly, hypovolemia also is a potent stimulus for AVP secretion in humans because an appropriate response to volume depletion should include renal water conservation. In humans and many animal species, lowering blood pressure suddenly by any of several methods increases plasma AVP levels by an amount that is proportional to the degree of hypotension achieved. This stimulus-response relationship follows an exponential pattern, so that small reductions in blood pressure, of the order of 5% to 10%, usually have little effect on plasma AVP levels, whereas blood pressure decreases of 20% to 30% result in hormone levels many times higher than those required to produce maximal antidiuresis (see Figure 16.4 ). The AVP response to acute reductions in blood volume appears to be quantitatively and qualitatively similar to the response to blood pressure. In rats, plasma AVP increases as an exponential function of the degree of hypovolemia. Thus, little increase in plasma AVP can be detected until blood volume falls by 5% to 8%; beyond that point, plasma AVP increases at an exponential rate in relation to the degree of hypovolemia and usually reaches levels 20 to 30 times normal when blood volume is reduced by 20% to 40%. The volume-AVP relationship has not been as thoroughly characterized in other species, but it appears to follow a similar pattern to that in humans. Conversely, acute increases in blood volume or pressure suppress AVP secretion. This response has been characterized less well than that of hypotension or hypovolemia, but seems to have a similar quantitative relationship (i.e., relatively large changes, ≈10% to 15%, are required to alter hormone secretion appreciably).
The minimal-to-absent effect of small changes in blood volume and pressure on AVP secretion contrasts sharply with the extraordinary sensitivity of the osmoregulatory system (see Figure 16.4 ). Recognition of this difference is essential for understanding the relative contribution of each system to control AVP secretion under physiologic and pathologic conditions. Because daily variations of total body water rarely exceed 2% to 3%, their effect on AVP secretion must be mediated largely, if not exclusively, by the osmoregulatory system. Nonetheless, modest changes in blood volume and pressure do, in fact, influence AVP secretion indirectly, even though they are weak stimuli by themselves. This occurs via shifting the sensitivity of AVP secretion to osmotic stimuli so that a given increase in osmolality will cause a greater secretion of AVP during hypovolemic conditions than during euvolemic states ( Figure 16.7 ). In the presence of a negative hemodynamic stimulus, plasma AVP continues to respond appropriately to small changes in plasma osmolality and can still be fully suppressed if the osmolality falls below the new (lower) set point. The retention of the threshold function is a vital aspect of the interaction because it ensures that the capability to regulate the osmolality of body fluids is not lost, even in the presence of significant hypovolemia or hypotension. Consequently, it is reasonable to conclude that the major effect of moderate degrees of hypovolemia on AVP secretion and thirst is to modulate the gain of the osmoregulatory responses, with direct effects on thirst and AVP secretion occurring only during more severe degrees of hypovolemia (e.g., >10% to 20% reduction in blood pressure or volume).

These hemodynamic influences on AVP secretion are mediated, at least in part, by neural pathways that originate in stretch-sensitive receptors, generally termed baroreceptors, in the cardiac atria, aorta, and carotid sinus (see Figure 16.2 ; reviewed in detail in Chapter 15 ). Afferent nerve fibers from these receptors ascend in the vagus and glossopharyngeal nerves to the nuclei of the tractus solitarius (NTS) in the brainstem. A variety of postsynaptic pathways from the NTS then project, directly and indirectly via the ventrolateral medulla and lateral parabrachial nucleus, to the PVN and SON in the hypothalamus. Early studies suggested that the input from these pathways was predominantly inhibitory under basal conditions because interrupting them acutely resulted in large increases in plasma AVP levels as well as in arterial blood pressure. However, as for most neural systems, including the neurohypophysis, innervation is complex and consists of excitatory and inhibitory inputs. Consequently, different effects have been observed under different experimental conditions.
The baroreceptor mechanism also appears to mediate a large number of pharmacologic and pathologic effects on AVP secretion ( Table 16.1 ). Among them are diuretics, isoproterenol, nicotine, prostaglandins, nitroprusside, trimethaphan, histamine, morphine, and bradykinin, all of which stimulate AVP, at least in part by lowering blood volume or pressure, and norepinephrine, which suppresses AVP by raising blood pressure. In addition, an upright posture, sodium depletion, congestive heart failure, cirrhosis, and nephrosis likely stimulate AVP secretion by reducing the effective circulating blood volume. Symptomatic orthostatic hypotension, vasovagal reactions, and other forms of syncope stimulate AVP secretion more markedly via greater and more acute decreases in blood pressure, with the exception of orthostatic hypotension associated with loss of afferent baroregulatory function. Almost every hormone, drug, or condition that affects blood volume or pressure will also affect AVP secretion but, in most cases, the degree of change of blood pressure or volume is modest and will result in a shift of the set point and/or sensitivity of the osmoregulatory response, rather than marked stimulation of AVP secretion (see Figure 16.7 ).
Stimulatory | Inhibitory |
---|---|
|
|
Drinking
Peripheral neural sensors other than baroreceptors also can affect AVP secretion. In humans, as well as dogs, drinking lowers plasma AVP before there is any appreciable decrease in plasma osmolality or serum [Na + ]. This is clearly a response to the act of drinking itself because it occurs independently of the composition of the fluid ingested, although it may be influenced by the temperature of the fluid because the degree of suppression appears to be greater in response to colder fluids. The pathways responsible for this effect have not been delineated, but likely include sensory afferents originating in the oropharynx and transmitted centrally via the glossopharyngeal nerve.
Nausea
Among other nonosmotic stimuli to AVP secretion in humans, nausea is the most prominent. The sensation of nausea, with or without vomiting, is the most potent stimulus to AVP secretion known in humans. Although 20% increases in osmolality will typically elevate plasma AVP levels to the range of 5 to 20 pg/mL, and 20% decreases in blood pressure to 10 to 100 pg/mL, nausea has been described to cause AVP elevations in excess of 200 to 400 pg/mL. The pathway mediating this effect has been mapped to the chemoreceptor zone in the area postrema of the brainstem in animal studies (see Figure 16.2 ). It can be activated by a variety of drugs and conditions, including apomorphine, morphine, nicotine, alcohol, and motion sickness. Its effect on AVP is instantaneous and extremely potent ( Figure 16.8 ), even when the nausea is transient and not accompanied by vomiting or changes in blood pressure. Pretreatment with fluphenazine, haloperidol, or promethazine in doses sufficient to prevent nausea completely abolishes the AVP response. The inhibitory effect of these dopamine antagonists is specific for emetic stimuli because they do not alter the AVP response to osmotic and hemodynamic stimuli. Water loading blunts, but does not abolish, the effect of nausea on AVP release, suggesting that osmotic and emetic influences interact in a manner similar to that for osmotic and hemodynamic pathways. Species differences also affect emetic stimuli. Whereas dogs and cats appear to be even more sensitive than humans to emetic stimulation of AVP release, rodents have little or no AVP response but release large amounts of OT instead.

The emetic response probably mediates many pharmacologic and pathologic effects on AVP secretion. In addition to the drugs and conditions already noted, it may be responsible at least in part for the increase in AVP secretion that has been observed with vasovagal reactions, diabetic ketoacidosis, acute hypoxia, and motion sickness. Because nausea and vomiting are frequent side effects of many other drugs and diseases, many additional situations likely occur as well. The reason for this profound stimulation is not known (although it has been speculated that the AVP response assists evacuation of stomach contents via contraction of gastric smooth muscle, AVP is not necessary for vomiting to occur), but it is responsible for the intense vasoconstriction that produces the pallor often associated with this state.
Hypoglycemia
Acute hypoglycemia is a less potent but reasonably consistent stimulus for AVP secretion. The receptor and pathway that mediate this effect are unknown; however, they appear separate from those of other recognized stimuli because hypoglycemia stimulates AVP secretion, even in patients who have selectively lost the capacity to respond to hypernatremia, hypotension, or nausea. The factor that actually triggers the release of AVP is likely intracellular deficiency of glucose or ATP because 2-deoxyglucose is also an effective stimulus. Generally, more than 20% decreases in glucose are required to increase plasma AVP levels significantly; the rate of decrease in the glucose level is probably the critical stimulus, however, because the rise in plasma AVP is not sustained with persistent hypoglycemia. However, glucopenic stimuli are of unlikely importance in the physiology or pathology of AVP secretion because there are probably few drugs or conditions that lower plasma glucose rapidly enough to stimulate release of the hormone, and because this effect is transient.
Renin Angiotensin Aldosterone System
The renin angiotensin aldosterone system (RAAS) has also been intimately implicated in the control of AVP secretion. Animal studies have indicated dual sites of action. Bloodborne Ang II stimulates AVP secretion by acting in the brain at the circumventricular subfornical organ (SFO), a small structure located in the dorsal portion of the third cerebral ventricle (see Figure 16.2 ). Because circumventricular organs lack a blood-brain barrier, the densely expressed Ang II receptor type 1 (AT 1 R) of the SFO can detect very small increases in blood levels of Ang II. Neural pathways from the SFO to the hypothalamic SON and PVN mediate AVP secretion, and also appear to use Ang II as a neurotransmitter. This accounts for the observation that the most sensitive site for angiotensin-mediated AVP secretion and thirst is intracerebroventricular injection into the cerebrospinal fluid. Further evidence in support of Ang II as a neurotransmitter is that intraventricular administration of angiotensin receptor antagonists inhibits the AVP response to osmotic and hemodynamic stimuli. The level of plasma Ang II required to stimulate AVP release is quite high, leading some to argue that this stimulus is active only under pharmacologic conditions. This is consistent with observations that even pressor doses of Ang II increase plasma AVP only about two- to fourfold and may account for the failure of some investigators to demonstrate stimulation of thirst by exogenous angiotensin. However, this procedure may underestimate the physiologic effects of angiotensin because the increased blood pressure caused by exogenously administered Ang II appears to blunt the thirst induced via activation of inhibitory baroreceptive pathways.
Stress
Nonspecific stress caused by factors such as pain, emotion, or physical exercise has long been thought to cause AVP secretion, but it has never been determined whether this effect is mediated by a specific pathway or is secondary to the hypotension or nausea that often accompanies stress-induced vasovagal reactions. In rats and humans, a variety of noxious stimuli capable of activating the pituitary-adrenal axis and sympathetic nervous system do not stimulate AVP secretion unless they also lower blood pressure or alter blood volume. The marked rise in plasma AVP levels elicited by manipulation of the abdominal viscera in anesthetized dogs has been attributed to nociceptive influences, but mediation by emetic pathways cannot be excluded in this setting. Endotoxin-induced fever stimulates AVP secretion in rats, and studies have supported the possible mediation of this effect by circulating cytokines, such as interleukin-1 (IL-1) and IL-6. Clarification of the possible role of nociceptive and thermal influences on AVP secretion is particularly important in view of the frequency with which painful or febrile illnesses are associated with osmotically inappropriate secretion of antidiurectic hormone.
Hypoxia and Hypercapnia
Acute hypoxia and hypercapnia also stimulate AVP secretion. In conscious humans, however, the stimulatory effect of moderate hypoxia (arterial partial pressure of oxygen [Pa o 2 ] > 35 mm Hg) is inconsistent and seems to occur mainly in subjects who develop nausea or hypotension. In conscious dogs, more severe hypoxia (Pa o 2 < 35 mm Hg) consistently increases AVP secretion without reducing arterial pressure. Studies of anesthetized dogs have suggested that the AVP response to acute hypoxia depends on the level of hypoxemia achieved. At a Pa o 2 of 35 mm Hg or lower, plasma AVP increases markedly, even though there is no change or even an increase in arterial pressure, but less severe hypoxia (Pa o 2 > 40 mm Hg) has no effect on AVP levels. These results indicate that there is likely a hypoxemic threshold for AVP secretion, and suggest that severe hypoxemia alone may also stimulate AVP secretion in humans. If so, it may be responsible, at least in part, for the osmotically inappropriate AVP elevations noted in some patients with acute respiratory failure. In conscious or anesthetized dogs, acute hypercapnia, independent of hypoxia or hypotension, also increases AVP secretion. It has not been determined whether this response also exhibits threshold characteristics or otherwise depends on the degree of hypercapnia, nor is it known whether hypercapnia has similar effects on AVP secretion in humans or other animals. The mechanisms whereby hypoxia and hypercapnia release AVP remain undefined, but they likely involve peripheral chemoreceptors and/or baroreceptors because cervical vagotomy abolishes the response to hypoxemia in dogs.
Drugs
As will be discussed more extensively in the section on clinical disorders, a variety of drugs also stimulate AVP secretion, including nicotine (see Table 16.1 ). Drugs and hormones can potentially affect AVP secretion at many different sites. As already discussed, many excitatory stimulants such as isoproterenol, nicotine, high doses of morphine, and cholecystokinin act, at least in part, by lowering blood pressure and/or producing nausea. Others, such as substance P, prostaglandin, endorphin, and other opioids, have not been studied sufficiently to define their mechanism of action, but they may also work by one or both of the same mechanisms. Inhibitory stimuli similarly have multiple modes of action. Vasopressor drugs such as norepinephrine inhibit AVP secretion indirectly by raising arterial pressure. In low doses, a variety of opioids of all subtypes, including morphine, met-enkephalin and κ-agonists, inhibit AVP secretion in rats and humans. Endogenous opioid peptides interact with the magnocellular neurosecretory system at several levels to inhibit basal and stimulated secretion of AVP and oxytocin. Opioid inhibition of AVP secretion has been found to occur in isolated posterior pituitary tissue, and the action of morphine and of several opioid agonists such as butorphanol and oxilorphan likely occurs via activation of κ-opioid receptors located on nerve terminals of the posterior pituitary. The well-known inhibitory effect of ethanol on AVP secretion may be mediated, at least in part, by endogenous opiates because it is due to an elevation in the osmotic threshold for AVP release and can be partially blocked by treatment with naloxone. Carbamazepine inhibits AVP secretion by diminishing the sensitivity of the osmoregulatory system; this effect occurs independently of changes in blood volume, blood pressure, and/or blood glucose. Other drugs that inhibit AVP secretion include clonidine, which appears to act via central and peripheral adrenoreceptors, muscimol, which acts as a GABA antagonist, and phencyclidine, which probably acts by raising blood pressure. However, despite the importance of these stimuli during pathologic conditions, none of them is a significant determinant of the physiologic regulation of AVP secretion in humans.
Distribution and Clearance
Plasma AVP concentration is determined by the difference between the rates of secretion from the posterior pituitary gland and removal of the hormone from the vascular compartment via metabolism and urinary clearance. In healthy adults, intravenously injected AVP distributes rapidly into a space equivalent in size to the ECF compartment. This initial, or mixing, phase has a half-life of 4 to 8 minutes and is virtually complete in 10 to 15 minutes. The rapid mixing phase is followed by a second, slower decline that corresponds to the metabolic clearance of AVP. Most studies of this phase have yielded mean values of 10 to 20 minutes by steady-state and non–steady-state techniques, consistent with the observed rates of change in urine osmolality after water loading and injection of AVP, which also support a short half-life. In pregnant women, the metabolic clearance rate of AVP increases nearly fourfold, which becomes significant in the pathophysiology of gestational diabetes insipidus (see later discussion). Smaller animals such as rats clear AVP much more rapidly than humans because their cardiac output is higher relative to their body weight and surface area.
Although many tissues have the capacity to inactivate AVP, metabolism in vivo appears to occur largely in the liver and kidney. The enzymatic processes whereby the liver and kidney inactivate AVP involve an initial reduction of the disulfide bridge, followed by aminopeptidase cleavage of the bond between amino acid residues 1 and 2 and cleavage of the C-terminal glycinamide residue. Some AVP is excreted intact in the urine, but there is disagreement about the amount and factors that affect it. For example, in healthy, normally hydrated adults, the urinary clearance of AVP ranges from 0.1 to 0.6 mL/kg/min under basal conditions and has never been found to exceed 2 mL/kg/min, even in the presence of solute diuresis. The mechanisms involved in the excretion of AVP have not been defined with certainty, but the hormone is probably filtered at the glomerulus and variably reabsorbed at sites along the nephron. The latter process may be linked to the reabsorption of Na + or other solutes in the proximal nephron because the urinary clearance of AVP has been found to vary by as much as 20-fold in direct relation to the solute clearance. Consequently, measurements of urinary AVP excretion in humans do not provide a consistently reliable index of changes in plasma AVP and should be interpreted cautiously when glomerular filtration or solute clearance are inconstant or abnormal.
Thirst
Thirst is the body’s defense mechanism to increase water consumption in response to perceived deficits of body fluids. It can be most easily defined as a consciously perceived desire for water. True thirst must be distinguished from other determinants of fluid intake such as taste, dietary preferences, and social customs, as discussed previously. Thirst can be stimulated in animals and humans by intracellular dehydration caused by increases in the effective osmolality of the ECF or by intravascular hypovolemia caused by losses of ECF. As would be expected, these are many of the same variables that provoke AVP secretion. Of these, hypertonicity is clearly the most potent. Similar to AVP secretion, substantial evidence to date has supported mediation of osmotic thirst by osmoreceptors located in the anterior hypothalamus of the brain, whereas hypovolemic thirst appears to be stimulated via activation of low- and/or high-pressure baroreceptors and circulating Ang II.
Osmotic Thirst
In healthy adults, an increase in effective plasma osmolality of only 2% to 3% above basal levels produces a strong desire to drink. This response is not dependent on changes in ECF or plasma volume because it occurs similarly whether plasma osmolality is raised by infusion of hypertonic solutions or by water deprivation. The absolute level of plasma osmolality at which a person develops a conscious urge to seek and drink water is termed the osmotic thirst threshold . It varies appreciably among individuals, likely as a result of genetic factors, but in healthy adults it averages approximately 295 mOsm/kg H 2 O. Of physiologic significance is the fact that this level is above the osmotic threshold for AVP release and approximates the plasma osmolality at which maximal concentration of the urine is normally achieved (see Figure 16.5 ).
The brain pathways that mediate osmotic thirst have not been well defined, but it is clear that initiation of drinking requires osmoreceptors located in the anteroventral hypothalamus in the same area as the osmoreceptors that control osmotic AVP secretion. Whether the osmoreceptors for AVP and thirst are the same cells or simply located in the same general area remains unknown. However, the properties of the osmoreceptors are very similar. Ineffective plasma solutes such as urea and glucose, which have little or no effect on AVP secretion, are equally ineffective at stimulating thirst, whereas effective solutes such as NaCl and mannitol can stimulate thirst. The sensitivities of the thirst and AVP osmoreceptors cannot be compared precisely, but they are also probably similar. Thus, in healthy adults, the intensity of thirst increases rapidly in direct proportion to serum [Na + ] or plasma osmolality and generally becomes intolerable at levels only 3% to 5% above the threshold level. Water consumption also appears to be proportional to the intensity of thirst in humans and animals and, under conditions of maximal osmotic stimulation, can reach rates as high as 20 to 25 L/day. The dilution of body fluids by ingested water complements the retention of water that occurs during AVP-induced antidiuresis, and both responses occur concurrently when drinking water is available.
As with AVP secretion, the osmoregulation of thirst appears to be bimodal because a modest decline in plasma osmolality induces a sense of satiation and reduces the basal rate of spontaneous fluid intake. This effect is sufficient to prevent hypotonic overhydration, even when antidiuresis is fixed at maximal levels for prolonged periods, suggesting that osmotically inappropriate secretion of AVP (syndrome of inappropriate antidiuretic hormone secretion [SIADH]) should not result in the development of hyponatremia unless the satiety mechanism is impaired or fluid intake is inappropriately high for some other reason, such as the unregulated components of fluid intake discussed earlier. Also similar to AVP secretion, thirst can be influenced by oropharyngeal or upper gastrointestinal receptors that respond to the act of drinking itself. In humans, however, the rapid relief provided by this mechanism lasts only a matter of minutes and thirst quickly recurs until enough water is absorbed to lower plasma osmolality to normal. Therefore, although local oropharyngeal sensations may have a significant short-term influence on thirst, it is the hypothalamic osmoreceptors that ultimately determine the volume of water intake in response to dehydration.
Hypovolemic Thirst
In contrast, the threshold for producing hypovolemic or extracellular thirst is significantly higher in animals and humans. Studies in several species have shown that sustained decreases in plasma volume or blood pressure of at least 4% to 8%, and in some species 10% to 15%, are necessary to stimulate drinking consistently. In humans, the degree of hypovolemia or hypotension required to produce thirst has not been precisely defined, but it has been difficult to demonstrate any effects of mild-to-moderate hypovolemia to stimulate thirst independently of osmotic changes occurring with dehydration. This blunted sensitivity to changes in ECF volume or blood pressure in humans probably represents an adaptation that occurred as a result of the erect posture of primates, which predisposes them to wider fluctuations in blood and atrial filling pressures as a result of orthostatic pooling of blood in the lower body. Stimulation of thirst (and AVP secretion) by such transient postural changes in blood pressure might lead to overdrinking and inappropriate antidiuresis in situations in which the ECF volume was actually normal but only transiently maldistributed. Consistent with a blunted response to baroreceptor activation, studies have also shown that systemic infusion of Ang II to pharmacologic levels is a much less potent stimulus to thirst in humans than in animals, in whom it is one of the most potent dipsogens known. Nonetheless, this response is not completely absent in humans, as demonstrated by rare cases of polydipsia in patients with pathologic causes of hyperreninemia. The pathways whereby hypovolemia or hypotension produces thirst have not been well defined, but probably involve the same brainstem baroreceptive pathways that mediate hemodynamic effects on AVP secretion, as well as a likely contribution from circulating levels of Ang II in some species.
Integration of Vasopressin Secretion and Thirst
A synthesis of what is presently known about the regulation of AVP secretion and thirst in humans leads to a relatively simple but elegant system to maintain water balance. Under normal physiologic conditions, the sensitivity of the osmoregulatory system for AVP secretion accounts for the maintenance of plasma osmolality within narrow limits by adjusting renal water excretion to small changes in osmolality. Stimulated thirst does not represent a major regulatory mechanism under these conditions, and unregulated fluid ingestion supplies adequate water in excess of true “need,” which is then excreted in relation to osmoregulated pituitary AVP secretion. However, when unregulated water intake cannot adequately supply body needs in the presence of plasma AVP levels sufficient to produce maximal antidiuresis, then plasma osmolality rises to levels that stimulate thirst (see Figure 16.5 ), and water intake increases proportional to the elevation of osmolality above this thirst threshold.
In such a system, thirst essentially represents a backup mechanism that becomes active when pituitary and renal mechanisms prove insufficient to maintain plasma osmolality within a few percent of basal levels. This arrangement has the advantage of freeing humans from frequent episodes of thirst. These would require a diversion of activities toward behavior oriented to seeking water when water deficiency is sufficiently mild to be compensated for by renal water conservation, but would stimulate water ingestion once water deficiency reaches potentially harmful levels. Stimulation of AVP secretion at plasma osmolalities below the threshold for subjective thirst acts to maintain an excess of body water sufficient to eliminate the need to drink whenever slight elevations in plasma osmolality occur. This system of differential effective thresholds for thirst and AVP secretion nicely complements many studies that have demonstrated excess unregulated (or need-free) drinking in humans and animals. Only when this mechanism becomes inadequate to maintain body fluid homeostasis does thirst-induced regulated fluid intake become the predominant defense mechanism for the prevention of severe dehydration.
Disorders of Insufficient Vasopressin or Vasopressin Effect
Disorders of insufficient AVP or AVP effect are associated with inadequate urine concentration and increased urine output (polyuria). If thirst mechanisms are intact, this is accompanied by compensatory increases in fluid intake (polydipsia) as a result of stimulated thirst to preserve body fluid homeostasis. The net result is polyuria and polydipsia, with preservation of normal plasma osmolality and serum electrolyte concentrations. However, if thirst is impaired, or if fluid intake is insufficient for any reason to compensate for the increased urine excretion, then hyperosmolality and hypernatremia can result, with the consequent complications associated with these disorders. The quintessential disorder of insufficient AVP is diabetes insipidus (DI), which is a clinical syndrome characterized by excretion of abnormally large volumes of urine (diabetes) that is dilute (hypotonic) and devoid of taste from dissolved solutes (e.g., insipid), in contrast to the hypertonic, sweet-tasting urine characteristic of diabetes mellitus (from the Greek, meaning honey).
Several different pathophysiologic mechanisms can cause hypotonic polyuria ( Table 16.2 ). Central DI (also called hypothalamic, neurogenic, or neurohypophyseal DI) is due to inadequate secretion and usually deficient synthesis of AVP in the hypothalamic neurohypophyseal system. Lack of AVP-stimulated activation of the V 2 subtype of AVP receptors in the kidney collecting tubules (see Chapters 10 and 11 ) causes excretion of large volumes of dilute urine. In most cases, thirst mechanisms are intact, leading to compensatory polydipsia. However, in a variant of central DI, osmoreceptor dysfunction, thirst is also impaired, leading to hypodipsia. DI of pregnancy is a transient disorder due to an accelerated metabolism of AVP as a result of increased activity of the enzyme oxytocinase or vasopressinase in the serum of pregnant women, again leading to polyuria and polydipsia; accelerated metabolism of AVP during pregnancy may also cause a patient with subclinical DI from other causes to shift from a relatively asymptomatic state to a symptomatic state as a result of the more rapid AVP degradation. Nephrogenic DI is due to inappropriate renal responses to AVP. This produces excretion of dilute urine, despite normal pituitary AVP secretion and secondary polydipsia, similar to central DI. The final cause of hypotonic polyuria, primary polydipsia, differs significantly from the other causes because it is not due to deficient AVP secretion or impaired renal responses to AVP, but rather to excessive ingestion of fluids. This can result from an abnormality in the thirst mechanism, in which case it is sometimes called dipsogenic DI, or from psychiatric disorders, in which case it is generally referred to as psychogenic polydipsia.
Central (Neurogenic) Diabetes Insipidus |
|
Osmoreceptor Dysfunction |
|
Increased AVP Metabolism |
Pregnancy |
Nephrogenic Diabetes Insipidus |
|
Primary Polydipsia |
|
Central Diabetes Insipidus
Causes
Central diabetes insipidus (CDI) is caused by inadequate secretion of AVP from the posterior pituitary in response to osmotic stimulation. In most cases, this is due to destruction of the neurohypophysis by a variety of acquired or congenital anatomic lesions that destroy or damage the neurohypophysis by pressure or infiltration (see Table 16.2 ). The severity of the resulting hypotonic diuresis depends on the degree of destruction of the neurohypophysis, leading to complete or partial deficiency of AVP secretion.
Despite the wide variety of lesions that can potentially cause CDI, it is much more common not to have CDI in the presence of such lesions than actually to produce the syndrome. This apparent inconsistency can be understood by considering several common principles of neurohypophyseal physiology and pathophysiology that are relevant to all these causes.
First, the synthesis of AVP occurs in the hypothalamus (see Figure 16.2 ); the posterior pituitary simply represents the site of storage and secretion of the neurosecretory granules that contain AVP. Consequently, lesions contained within the sella turcica that destroy only the posterior pituitary generally do not cause CDI because the cell bodies of the magnocellular neurons that synthesize AVP remain intact, and the site of release of AVP shifts more superiorly, typically into the blood vessels of the median eminence at the base of the brain. Perhaps the best examples of this phenomenon are large pituitary macroadenomas that completely destroy the anterior and posterior pituitary. DI is a distinctly unusual presentation for such pituitary adenomas because destruction of the posterior pituitary by such slowly enlarging intrasellar lesions merely destroys the nerve terminals, but not the cell bodies, of the AVP neurons. As this occurs, the site of release of AVP shifts more superiorly to the pituitary stalk and median eminence. Sometimes this can be detected on noncontrast magnetic resonance imaging (MRI) scans as a shift of the pituitary bright spot more superiorly to the level of the infundibulum or median eminence, but this process is often too diffuse to be detected in this manner. The development of DI from a pituitary adenoma is so uncommon, even with macroadenomas that completely obliterate sellar contents sufficiently to cause panhypopituitarism, that its presence should lead to consideration of alternative diagnoses, such as craniopharyngioma, which often causes damage to the median eminence because of adherence of the capsule to the base of the hypothalamus, more rapidly enlarging sellar or suprasellar masses that do not allow sufficient time for shifting the site of AVP release more superiorly (e.g., metastatic lesions), or granulomatous disease, with more diffuse hypothalamic involvement (e.g., sarcoidosis, histiocytosis). With very large pituitary adenomas that produce adrenocorticotropic hormone (ACTH) deficiency, it is actually more likely that patients will present with hypo-osmolality from an SIADH-like picture as a result of the impaired free water excretion that accompanies hypocortisolism, as will be discussed later.
A second general principle is that the capacity of the neurohypophysis to synthesize AVP is greatly in excess of the body’s daily needs for maintenance of water homeostasis. Carefully controlled studies of surgical section of the pituitary stalk in dogs have clearly demonstrated that destruction of 80% to 90% of the magnocellular neurons in the hypothalamus is required to produce polyuria and polydipsia in these species. Thus, even lesions that cause destruction of the AVP magnocellular neuron cell bodies must result in a large degree of destruction to produce DI. The most illustrative example of this is surgical section of the pituitary stalk in humans. Necropsy studies of these patients have revealed atrophy of the posterior pituitary and loss of the magnocellular neurons in the hypothalamus. This loss of magnocellular cells presumably results from retrograde degeneration of neurons whose axons were cut during surgery. As is generally true for all neurons, the likelihood of retrograde neuronal degeneration depends on the proximity of the axotomy, in this case section of the pituitary stalk, to the cell body of the neuron. This was shown clearly in studies of human subjects in whom section of the pituitary stalk at the level of the diaphragm sellae (a low stalk section) produced transient but not permanent DI, whereas section at the level of the infundibulum (a high stalk section) was required to cause permanent DI in most cases.
Several genetic causes of AVP deficiency have also been characterized. Prior to the application of techniques for amplification of genomic DNA, the only experimental model to study the mechanism of hereditary hypothalamic DI was the Brattleboro rat, a strain that was found serendipitously to have CDI. In this animal, the disease demonstrates a classic pattern of autosomal recessive inheritance in which DI is expressed only in the homozygotes. The hereditary basis of the disease has been found to be a single base deletion producing a translational frameshift beginning in the third portion of the neurophysin coding sequence. Because the gene lacks a stop codon, there is a modified neurophysin, no glycopeptides, and a long polylysine tail. Although the mutant prohormone accumulates in the endoplasmic reticulum, sufficient AVP is produced by the normal allele that the heterozygotes are asymptomatic. In contrast, almost all families with genetic CDI in humans that have been described to date demonstrate an autosomal dominant mode of inheritance. In these cases, DI is expressed, despite the expression of one normal allele, which is sufficient to prevent the disease in the heterozygous Brattleboro rats. Numerous studies have been directed at understanding this apparent anomaly. Two potentially important clues about the cause of the DI in familial genetic CDI are the following:
- 1.
Severe-to-partial deficiencies of AVP and overt signs of DI do not develop in these patients until several months to several years after birth and then gradually progress over the ensuing decades, suggesting adequate initial function of the normal allele, with later decompensation.
- 2.
A limited number of autopsy studies have suggested that some of these cases are associated with gliosis and a marked loss of magnocellular AVP neurons in the hypothalamus, although other studies have shown normal neurons with decreased expression of AVP or no hypothalamic abnormality. In most of these cases, the hyperintense signal normally emitted by the neurohypophysis in T1-weighted MRI scans (see later discussion) is also absent, although some exceptions have been reported.
Another interesting, but as yet unexplained, observation is that some adults in these families have been described in whom DI was clinically apparent during childhood but who went into remission as adults, without evidence that their remissions could be attributed to renal or adrenal insufficiency or to increased AVP synthesis.
The autosomal dominant form of familial CDI is caused by diverse mutations in the gene that codes for the AVP-neurophysin precursor ( Figure 16.9 ). All the mutations identified to date have been in the coding region of the gene and affect only one allele. They are located in all three exons and are predicted to alter or delete amino acid residues in the signal peptide, AVP, and neurophysin moieties of the precursor. Only the C-terminus glycopeptide, or copeptin moiety, has not been found to be affected. Most are missense mutations, but nonsense mutations (premature stop codons) and deletions also occur. One characteristic shared by all the mutations is that they are predicted to alter or delete one or more amino acids known, or reasonably presumed, to be crucial for processing, folding, and oligomerization of the precursor protein in the endoplasmic reticulum. Because of the related functional effects of the mutations, the common clinical characteristics of the disease, the dominant-negative mode of transmission, and the autopsy and hormonal evidence of postnatal neurohypophyseal degeneration, it has been postulated that all the mutations act by causing production of an abnormal precursor protein that accumulates and eventually kills the neurons because it cannot be correctly processed, folded, and transported out of the endoplasmic reticulum. Expression studies of mutant DNA from several human mutations in cultured neuroblastoma cells support this misfolding-neurotoxicity hypothesis by demonstrating abnormal trafficking and accumulation of mutant prohormone in the endoplasmic reticulum with low or absent expression in the Golgi apparatus, suggesting difficulty with packaging into neurosecretory granules. However, cell death may not be necessary to decrease available AVP. Normally, proteins retained in the endoplasmic reticulum are selectively degraded, but if excess mutant is produced and the selective normal degradative process is overwhelmed, an alternate, nonselective, degradative system (autophagy) is activated. As more and more mutant precursor builds up in the endoplasmic reticulum, the normal wild-type protein becomes trapped with the mutant protein and degraded by the activated nonspecific degradative system. In this case, the amount of AVP that matures and is packaged would be markedly reduced. This explanation is consistent with those cases in which little pathology is found in the magnocellular neurons and also with DI in which a small amount of AVP can still be detected.

Wolfram’s syndrome is a rare autosomal recessive disease with DI, diabetes mellitus, optic atrophy, and deafness (DIDMOAD). The genetic defect is the protein wolframin, which is found in the endoplasmic reticulum and is important for folding proteins. Wolframin is involved in β-cell proliferation, intracellular protein processing, and calcium homeostasis, producing a wide spectrum of endocrine and central nervous system (CNS) disorders; DI is usually a late manifestation associated with decreased magnocellular neurons in the paraventricular and supraoptic nuclei.
Idiopathic forms of AVP deficiency represent a large pathogenic category in adults and children. A study in children has revealed that over half (54%) of all cases of CDI were classified as idiopathic. These patients do not have historic or clinical evidence of any injury or disease that can be linked to their DI, and MRI of the pituitary-hypothalamic area generally reveals no abnormality other than the absence of the posterior pituitary bright spot and sometimes varying degrees of thickening of the pituitary stalk. Several lines of evidence have suggested that many of these patients may have had an autoimmune destruction of the neurohypophysis to account for their DI. First, the entity of lymphocytic infundibuloneurohypophysitis has been documented to be present in a subset of patients with idiopathic DI. Lymphocytic infiltration of the anterior pituitary, lymphocytic hypophysitis, has been recognized as a cause of anterior pituitary deficiency for many years, but it was not until an autopsy called attention to a similar finding in the posterior pituitary of a patient with DI that this pathology was recognized to occur in the neurohypophysis as well. Since that initial report, a number of similar cases have been described, including cases in the postpartum period, which is characteristic of lymphocytic hypophysitis. With the advent of MRI, lymphocytic infundibuloneurohypophysitis has been diagnosed based on the appearance of a thickened stalk and/or enlargement of the posterior pituitary, mimicking a pituitary tumor. In these cases, the characteristic bright spot on MRI T1-weighted images is lost. The enlargement of the stalk can mimic a neoplastic process, resulting in some of these patients undergoing surgery based on the suspicion of a pituitary tumor. Since then, a number of patients with a suspicion of infundibuloneurohypophysitis and no other obvious cause of DI have been followed and have shown regression of the thickened pituitary stalk over time. Several cases have been reported with the coexistence of CDI and adenohypophysitis; these presumably represent cases of combined lymphocytic infundibuloneurohypophysitis and hypophysisis. A second line of evidence supporting an autoimmune cause in many cases of idiopathic DI is based on the finding of AVP antibodies in the serum of as many as one third of patients with idiopathic DI and two thirds of those with Langerhans cell histiocytosis X, but not in patients with DI caused by tumors. A recently recognized form of infundibuloneurohypophysitis occurs in middle-aged to older men and is associated with immunoglobulin G4 (IgG4)–related systemic disease. Various organs, especially the pancreas, are infiltrated with IgG4 plasma cells, and neurohypophysitis is only one manifestation of a multiorgan disease that may include other endocrine glands. This cause should be considered as a cause of DI based on age and gender at presentation and evidence of other systemic disease. The diagnosis can be established by elevated serum IgG4 levels and characteristic histology of biopsies. Response to steroids or other immunosuppressive drugs is characteristic.
Pathophysiology
The normal inverse relationship between urine volume and urine osmolality (see Figure 16.5 ) means that initial decreases in maximal AVP secretion will not cause an increase in urine volume sufficient to be detected clinically by polyuria. In general, basal AVP secretion must fall to less than 10% to 20% of normal before basal urine osmolality decreases to less than 300 mOsm/kg H 2 O and urine flow increases to symptomatic levels (i.e., >50 mL/kg BW/day). This resulting loss of body water produces a slight rise in plasma osmolality that stimulates thirst and induces a compensatory polydipsia. The resultant increase in water intake restores balance with urine output and stabilizes the osmolality of body fluids at a new, slightly higher but still normal level. As the AVP deficit increases, this new steady-state level of plasma osmolality approximates the osmotic threshold for thirst (see Figure 16.5 ). It is important to recognize that the deficiency of AVP need not be complete for polyuria and polydipsia to occur; it is only necessary that the maximal plasma AVP concentration achievable at or below the osmotic threshold for thirst is inadequate to concentrate the urine. The degree of neurohypophyseal destruction at which such failure occurs varies considerably from person to person, largely because of individual differences in the set point and sensitivity of the osmoregulatory system. In general, functional tests of AVP levels in patients with DI of variable severity, duration, and cause have indicated that AVP secretory capacity must be reduced by at least 75% to 80% for significant polyuria to occur, which also agrees with neuroanatomic studies of cell loss in the supraoptic nuclei of dogs with experimental pituitary stalk section and of patients who had undergone pituitary surgery.
Because renal mechanisms for sodium conservation are unimpaired with impaired or absent AVP secretion, there is no accompanying sodium deficiency. Although untreated DI can lead to hyperosmolality and volume depletion, until the water losses become severe, volume depletion is minimized by osmotic shifts of water from the ICF compartment to the more osmotically concentrated ECF compartment. This phenomenon is not as evident following increases in ECF [Na + ] because such osmotic shifts result in a slower increase in the serum [Na + ] than would otherwise occur. However, when non–sodium solutes such as mannitol are infused, this effect is more obvious due to the progressive dilutional decrease in serum [Na + ] caused by translocation of intracellular water to the ECF compartment. Because patients with DI do not have impaired urine Na + conservation, the ECF volume is generally not markedly decreased, and regulatory mechanisms for the maintenance of osmotic homeostasis are primarily activated—stimulation of thirst and AVP secretion (to whatever degree the neurohypophysis is still able to secrete AVP). In cases in which AVP secretion is totally absent (complete DI), patients are dependent entirely on water intake for the maintenance of water balance. However, in cases in which some residual capacity to secrete AVP remains (partial DI), plasma osmolality can eventually reach levels that allow moderate degrees of urinary concentration ( Figure 16.10 ).

The development of DI following surgical or traumatic injury to the neurohypophysis represents a unique situation and can follow any of several different, well-defined patterns. In some patients, polyuria develops 1 to 4 days after injury and resolves spontaneously. Less often, the DI is permanent and continues indefinitely (see previous discussion on the relationship between the level of pituitary stalk section and development of permanent DI). Most interestingly, a triphasic response can occur as a result of pituitary stalk transection ( Figure 16.11 ). The initial DI (first phase) is due to axon shock and lack of function of the damaged neurons. This phase lasts from several hours to several days and is followed by an antidiuretic phase (second phase) that is due to the uncontrolled release of AVP from the disconnected and degenerating posterior pituitary or from the remaining severed neurons. Overly aggressive administration of fluids during this second phase does not suppress the AVP secretion and can lead to hyponatremia. The antidiuresis can last from 2 to 14 days, after which DI recurs following depletion of the AVP from the degenerating posterior pituitary gland (third phase).

Transient hyponatremia without preceding or subsequent DI has been reported following transsphenoidal surgery for pituitary microadenomas, which generally occurs 5 to 10 days postoperatively. The incidence may be as high as 30% when these patients are carefully followed, although most cases are mild and self-limited. This is due to inappropriate AVP secretion via the same mechanism as in the triphasic response, except that in these cases only the second phase occurs (isolated second phase) because the initial neural lobe or pituitary stalk damage is not sufficient to impair AVP secretion enough to produce clinical manifestations of DI (see Figure 16.11 ).
Once a deficiency of AVP secretion has been present for more than a few days or weeks, it rarely improves, even if the underlying cause of the neurohypophyseal destruction is eliminated. The major exception to this is in patients with postoperative DI, for whom spontaneous resolution is the rule. Although recovery from DI that persists more than several weeks postoperatively is less common, well-documented cases of long-term recovery have nonetheless been reported. The reason for amelioration and resolution is apparent from pathologic and histologic examination of neurohypophyseal tissue following pituitary stalk section. Neurohypophyseal neurons that have intact perikarya are able to regenerate axons and form new nerve terminal endings capable of releasing AVP into nearby capillaries. In animals, this may be accompanied by a bulbous growth at the end of the severed stalk, which represents a new, albeit small, neural lobe. In humans, the regeneration process appears to proceed more slowly, and formation of a new neural lobe has not been noted. Nonetheless, histologic examination of a severed human stalk from a patient 18 months after hypophysectomy has demonstrated reorganization of neurohypophyseal fibers, with neurosecretory granules in close proximity to nearby blood vessels, closely resembling the histology of a normal posterior pituitary.
Recognition of the fact that almost all patients with CDI retain a limited capacity to secrete some AVP allows an understanding of some otherwise perplexing features of the disorder. For example, in many patients, restricting water intake long enough to raise plasma osmolality by only 1% to 2% induces sufficient AVP secretion to concentrate the urine ( Figure 16.12 ). As the plasma osmolality increases further, some patients with partial DI can even secrete enough AVP to achieve near-maximal urine osmolality (see Figure 16.10 ). However, this should not cause confusion about the diagnosis of DI because, in these patients, the urine osmolality will still be inappropriately low at plasma osmolality within a normal range, and they will respond to exogenous AVP administration with further increases in urine osmolality. These responses to dehydration illustrate the relative nature of the AVP deficiency in most cases and underscore the importance of the thirst mechanism to restrict the use of residual secretory capacity under basal conditions of ad lib water intake.





CDI is also associated with changes in the renal response to AVP. The most obvious change is a reduction in maximal concentrating capacity, which has been attributed to washout of the medullary concentration gradient caused by the chronic polyuria. The severity of this defect is proportional to the magnitude of the polyuria and is independent of its cause. Because of this, the level of urinary concentration achieved at maximally effective levels of plasma AVP is reduced in all types of DI. In patients with CDI, this concentrating abnormality is offset to some extent by an apparent increase in renal sensitivity to low levels of plasma AVP ( Figure 16.13 ). The cause of this supersensitivity is unknown, but it may reflect upward regulation of AVP V 2 receptor expression or function secondary to a chronic deficiency of the hormone.





Osmoreceptor Dysfunction
Causes
There is an extensive literature in animals indicating that the primary osmoreceptors controlling AVP secretion and thirst are located in the anterior hypothalamus; lesions of this region in animals, called the AV3V area, cause hyperosmolality through a combination of impaired thirst and impaired osmotically stimulated AVP secretion. Initial reports in humans described this syndrome as essential hypernatremia, and subsequent studies used the term adipsic hypernatremia in recognition of the profound thirst deficits found in most of the patients. Based on the known pathophysiology, all these syndromes can be grouped together as disorders of osmoreceptor dysfunction. Although the pathologies responsible for this condition can be quite varied, all the cases reported to date have been due to various degrees of osmoreceptor destruction associated with a variety of different brain lesions, as summarized in Table 16.2 . Many of these are the same types of lesions that can cause CDI but, in contrast to CDI, these lesions usually occur more rostrally in the hypothalamus, consistent with the anterior hypothalamic location of the primary osmoreceptor cells (see Figure 16.2 ). One lesion that is unique to this disorder is an anterior communicating cerebral artery aneurysm. Because the small arterioles that feed the anterior wall of the third ventricle originate from the anterior communicating cerebral artery, an aneurysm in this region (but more often following surgical repair of such an aneurysm that typically involves ligation of the anterior communicating artery ) produces infarction of the part of the hypothalamus containing the osmoreceptor cells.
Pathophysiology
The cardinal defect of patients with this disorder is lack of the osmoreceptors that regulate thirst. With rare exceptions, osmoregulation of AVP is also impaired, although the hormonal response to nonosmotic stimuli remains intact ( Figure 16.14 ). Four major patterns of osmoreceptor dysfunction have been described as characterized by defects in thirst and/or AVP secretory responses: (1) upward resetting of the osmostat for both thirst and AVP secretion (normal AVP and thirst responses but at an abnormally high plasma osmolality); (2) partial osmoreceptor destruction (blunted AVP and thirst responses at all plasma osmolalities); (3) total osmoreceptor destruction (absent AVP secretion and thirst, regardless of plasma osmolality); and (4) selective dysfunction of thirst osmoregulation with intact AVP secretion. Regardless of the actual pattern, the hallmark of this disorder is an abnormal thirst response in addition to variable defects in AVP secretion. Thus, such patients fail to drink sufficiently as their plasma osmolality rises and, as a result, the new set point for plasma osmolality rises far above the normal thirst threshold. Unlike patients with CDI, whose polydipsia maintains their plasma osmolality within a normal range, patients with osmoreceptor dysfunction typically have osmolality in the range of 300 to 340 mOsm/kg H 2 O. This again underscores the critical role played by normal thirst mechanisms in maintaining body fluid homeostasis; intact renal function alone is insufficient to maintain plasma osmolality within normal limits in such cases.

The rate of development and severity of hyperosmolality and hypertonic dehydration in patients with osmoreceptor dysfunction are influenced by a number of factors. First is the ability to maintain some degree of osmotically stimulated thirst and AVP secretion, which will determine the new set point for plasma osmolality. Second are environmental influences that affect the rate of water output. When physical activity is minimal, and the ambient temperature is not elevated, the overall rates of renal and insensible water loss are low, and the patient’s diet may be sufficient to maintain a relatively normal balance for long periods of time. Anything that increases perspiration, respiration, or urine output greatly accelerates the rate of water loss and thereby uncovers the patient’s inability to mount an appropriate compensatory increase in water intake. Under these conditions, severe and even fatal hypernatremia can develop relatively quickly. When the dehydration is only moderate (plasma osmolality = 300 to 330 mOsm/kg H 2 O), the patient is usually asymptomatic and signs of volume depletion are minimal, but if the dehydration becomes severe, the patient can exhibit symptoms and signs of hypovolemia, including weakness, postural dizziness, paralysis, confusion, coma, azotemia, hypokalemia, hyperglycemia, and secondary hyperaldosteronism (see later, “ Clinical Manifestations of Diabetes Insipidus ”). In severe cases, there may also be rhabdomyolysis, with marked serum elevations in muscle enzyme levels and occasionally acute renal failure.
However, a third factor also influences the degree of hyperosmolality and dehydration present in these patients. For all cases of osmoreceptor dysfunction, it is important to remember that afferent pathways from the brainstem to the hypothalamus remain intact; therefore, these patients will usually have normal AVP and renal concentrating responses to baroreceptor-mediated stimuli, such as hypovolemia and hypotension (see Figure 16.14 ), or to other nonosmotic stimuli, such as nausea (see Figure 16.8 ). This has the effect of preventing severe dehydration because, as hypovolemia develops, this will stimulate AVP secretion via baroreceptive pathways through the brainstem (see Figure 16.2 ). Although protective, this effect often causes confusion, because sometimes these patients appear to have DI yet at other times they can concentrate their urine quite normally. Nonetheless, the presence of refractory hyperosmolality with absent or inappropriate thirst should alert clinicians to the presence of osmoreceptor dysfunction, regardless of apparent normal urine concentration occasionally.
In a few patients with osmoreceptor dysfunction, forced hydration has been found to lead to hyponatremia in association with inappropriate urine concentration. This paradoxic defect resembles that seen in SIADH and has been postulated to be caused by two different pathogenic mechanisms. One is continuous or fixed secretion of AVP because of loss of the capacity for osmotic inhibition and stimulation of hormone secretion. These observations, as well as electrophysiologic data, have strongly suggested that the osmoregulatory system is bimodal (i.e., it is composed of inhibitory and stimulatory input to the neurohypophysis; see Figure 16.6 ). The other cause of the diluting defect appears to be hypersensitivity to the antidiuretic effects of AVP because, in some patients, urine osmolality may remain elevated, even when the hormone is undetectable.
Hypodipsia is also a common occurrence in older adults in the absence of any overt hypothalamic lesion. In such cases, it is not clear whether the defect is in the hypothalamic osmoreceptors, in their projections to the cortex, or in some other regulatory mechanism. However, in most cases, the osmoreceptor is likely not involved because basal and stimulated plasma AVP levels have been found to be normal, or even hyperresponsive, in relation to plasma osmolality in older adults, with the exception of only a few studies that showed decreased plasma levels of AVP relative to plasma osmolality.
Gestational Diabetes Insipidus
Causes
A relative deficiency of plasma AVP can also result from an increase in the rate of AVP metabolism. This condition has been observed only in pregnancy and therefore it is generally termed gestational diabetes insipidus. It is due to the action of a circulating enzyme called cysteine aminopeptidase (oxytocinase or vasopressinase) that is normally produced by the placenta to degrade circulating oxytocin and prevent premature uterine contractions. Because of the close structural similarity between AVP and OT, this enzyme degrades both peptides. There are two types of gestational diabetes insipidus. In the first type, the activity of cysteine aminopeptidase is extremely and abnormally elevated. This syndrome has been referred to as vasopressin-resistant diabetes insipidus of pregnancy. It can occur in association with preeclampsia, acute fatty liver, and coagulopathies (e.g., HELLP syndrome [ h emolysis, e levated l iver enzymes, and l ow p latelet count]). These patients have decreased metabolism of vasopressinase by the liver. Usually, in subsequent pregnancies, these women have neither diabetes insipidus nor acute fatty liver. In the second type, the accelerated metabolic clearance of vasopressin produces DI in a patient with borderline vasopressin function from a specific disease (e.g., mild nephrogenic DI or partial CDI). AVP is rapidly destroyed, and the neurohypophysis is unable to keep up with the increased demand. Labor and parturition usually proceed normally, and patients have no trouble with lactation. There is the threat of chronic and severe dehydration when DI is unrecognized, and this may pose a threat to a pregnant woman. The relationship of this disorder to the transient nephrogenic DI (NDI) of pregnancy is not clear.
Pathophysiology
The pathophysiology of gestational DI is similar to that of CDI. The only exception is that the polyuria is usually not corrected by administration of AVP because this is rapidly degraded, just as is endogenous AVP, but it can be controlled by treatment with desmopressin, the AVP V 2 receptor agonist that is more resistant to degradation by oxytocinase or vasopressinase. It should be remembered that patients with partial CDI in whom only low levels of AVP can be maintained, or patients with compensated NDI in whom the lack of response of the kidney to AVP may not be absolute, can be relatively asymptomatic with regard to polyuria. However, with accelerated destruction of AVP during pregnancy, the underlying DI may become manifest. Consequently, patients presenting with gestational DI should not be assumed simply to have excess oxytocinase or vasopressinase; rather, these patients should be evaluated for other possible underlying pathologic diagnoses (see Table 16.2 ).
Nephrogenic Diabetes Insipidus
Causes
Resistance to the antidiuretic action of AVP is usually due to some defect within the kidney, and is commonly referred to as nephrogenic diabetes insipidus (NDI). It was first recognized in 1945 in several patients with the familial, sex-linked form of the disorder. Subsequently, additional kindreds with the X-linked form of familial NDI were identified. Clinical studies of NDI have indicated that symptomatic polyuria is present from birth, plasma AVP levels are normal or elevated, resistance to the antidiuretic effect of AVP can be partial or almost complete, and the disease affects mostly males and is usually, although not always, mild or absent in carrier females. More than 90% of cases of congenital NDI are caused by mutations of the AVP V 2 receptor (see Chapter 45 ). Most mutations occur in the part of the receptor that is highly conserved among species and/or is conserved among similar receptors, such as homologies with AVP V 1A or OT receptors. The effect of some of these mutations on receptor synthesis, processing, trafficking, and function has been studied by in vitro expression. These types of studies have shown that the various mutations cause several different defects in cellular processing and function of the receptor but can be classified into four general categories based on differences in transport to the cell surface and AVP binding and/or stimulation of adenylyl cyclase: (1) the mutant receptor is not inserted in the membrane; (2) the mutant receptor is inserted in the membrane but does not bind or respond to AVP; (3) the mutant receptor is inserted in the membrane and binds AVP but does not activate adenylyl cyclase; or (4) the mutant protein is inserted into the membrane and binds AVP but responds subnormally in terms of adenylyl cyclase activation. Two studies have shown a relationship between the clinical phenotype and genotype and/or cellular phenotype. Approximately 10% of the V 2 receptor defects causing congenital NDI are thought to be de novo. This high incidence of de novo cases, coupled with the large number of mutations that have been identified, hinders the clinical use of genetic identification because it is necessary to sequence the entire open reading frame of the receptor gene rather than short sequences of DNA. Nonetheless, the use of automated gene sequencing techniques in selected families has been shown to identify mutations in patients with clinical disease and in asymptomatic carriers. Although most female carriers of the X-linked V 2 receptors defect have no clinical disease, some have been reported with symptomatic NDI. Carriers can have a decreased maximum urine osmolality in response to plasma AVP levels, but are generally asymptomatic because of the absence of overt polyuria. In one study, a girl manifested severe NDI due to a V 2 receptor mutation, which was likely due to skewed inactivation of the normal X chromosome.
Congenital NDI can also result from mutations of the autosomal gene that codes for AQP2, the protein that forms the water channels in renal medullary collecting tubules. When the proband is a girl, it is likely the defect is a mutation of the AQP2 gene on chromosome 12, region q12-q13. More than 20 different mutations of the AQP2 gene have been described (see Chapter 45 ). The patients may be heterozygous for two different recessive mutations or homozygous for the same abnormality from both parents. Because most of these mutations are recessive, the patients usually do not present with a family history of DI unless consanguinity is present. Functional expression studies of these mutations have shown that all of them result in varying degrees of reduced water transport because the mutant aquaporins are not expressed in normal amounts, are retained in various cellular organelles, or simply do not function as effectively as water channels. Regardless of the type of mutation, the phenotype of NDI from AQP2 mutations is identical to that produced by V 2 receptor mutations. Some of the defects in cellular routing and water transport can be reversed by treatment with chemicals that act like chaperones, suggesting that misfolding of the mutant AQP2 may be responsible for misrouting. Similar salutary effects of chaperones have been found to reverse defects in cell surface expression and function of selected mutations of the AVP V 2 receptor.
NDI can also be caused by a variety of drugs, diseases, and metabolic disturbances, including lithium, hypokalemia, and hypercalcemia (see Table 16.2 ). Some of these disorders (e.g., polycystic kidney disease) act to distort the normal architecture of the kidney and interfere with the normal urine concentration process. However, experimental studies in animal models have suggested that many have in common a downregulation of AQP2 expression in the renal collecting tubules ( Figure 16.15 ; see also Chapters 10 and 11 ). The polyuria associated with potassium deficiency develops in parallel with decreased expression of kidney AQP2, and repletion of potassium reestablishes the normal urinary concentrating mechanism and normalizes renal expression of AQP2. Similarly, hypercalcemia has also been found to be associated with downregulation of AQP2. A low-protein diet diminishes the ability to concentrate the urine, primarily by a decreased delivery of urea to the inner medulla, thus decreasing the medullary concentration gradient, but rats on a low-protein diet also appear to downregulate AQP2, which could be an additional component of the decreased ability to concentrate the urine. Bilateral urinary tract obstruction causes an inability to produce a maximum concentration of the urine, and rat models have demonstrated a downregulation of AQP2, which persists for several days after release of the obstruction. However, it is not yet clear which of these effects on AQP2 expression are primary or secondary, and which cellular mechanism(s) are responsible for the downregulation of AQP2 expression.

The administration of lithium to treat psychiatric disorders is the most common cause of drug-induced NDI and illustrates the multiple mechanisms likely involved in producing this disorder. As many as 10% to 20% of patients on chronic lithium therapy develop some degree of NDI. Lithium is known to interfere with the production of cAMP and produces a dramatic (95%) reduction in kidney AQP2 levels in animals. The defect of aquaporins is slow to correct in experimental animals and humans and, in some cases, it can be permanent in association with glomerular or tubulointerstitial nephropathy. Several other drugs that are known to induce renal concentrating defects have also been associated with abnormalities of AQP2 synthesis.
Pathophysiology
Similar to CDI, renal insensitivity to the antidiuretic effect of AVP also results in the excretion of an increased volume of dilute urine, decrease in body water, and rise in plasma osmolality, which by stimulating thirst induces a compensatory increase in water intake. As a consequence, the osmolality of body fluid stabilizes at a slightly higher level, which approximates the osmotic threshold for thirst. As in patients with CDI, the magnitude of polyuria and polydipsia varies greatly depending on a number of factors, including the degree of renal insensitivity to AVP, individual differences in the set points and sensitivity of thirst and AVP secretion, and total solute load. It is important to note that the renal insensitivity to AVP need not be complete for polyuria to occur; it is only necessary that the defect is great enough to prevent concentration of the urine at plasma AVP levels achievable under ordinary conditions of ad lib water intake (i.e., at plasma osmolalities near the osmotic threshold for thirst). Calculations similar to those used for states of AVP deficiency indicate that this requirement is not met until the renal sensitivity to AVP is reduced by more than 10-fold. Because renal insensitivity to the hormone is often incomplete, especially in cases of acquired rather than congenital NDI, many patients with NDI are able to concentrate their urine to varying degrees when they are deprived of water or given large doses of desmopressin.
Information about the renal concentration mechanism from studies of AQP2 expression in experimental animals (see Chapters 10 and 11 ) has suggested that a form of NDI is likely associated with all types of DI, as well as with primary polydipsia. Brattleboro rats have been found to have low levels of kidney AQP2 expression compared to Long-Evans control rats; AQP2 levels are corrected by treatment with AVP or desmopressin, but this process takes 3 to 5 days, during which time urine concentration remains subnormal, despite pharmacologic concentrations of AVP. Similarly, physiologic suppression of AVP by chronic overadministration of water produces a downregulation of AQP2 in the renal collecting duct. Clinically, it is well known that patients with both CDI and primary polydipsia often fail to achieve maximally concentrated urine when they are given desmopressin during a water deprivation test to differentiate among the various causes of DI. This effect has long been attributed to a washout of the medullary concentration gradient as a result of the high urine flow rates in polyuric patients; however, based on the results of animal studies, it seems certain that at least part of the decreased response to AVP is due to a downregulation of kidney AQP2 expression. This also explains why it takes time, typically several days, to restore normal urinary concentration after patients with primary polydipsia and CDI are treated with water restriction or antidiuretic therapy.
Primary Polydipsia
Causes
Excessive fluid intake also causes hypotonic polyuria and, by definition, polydipsia. Consequently, this disorder must be differentiated from the various causes of DI. Furthermore, it is apparent that despite normal pituitary and kidney function, patients with this disorder share many characteristics of both CDI (AVP secretion is suppressed as a result of the decreased plasma osmolality) and NDI (kidney AQP2 expression is decreased as a result of the suppressed plasma AVP levels). Many different names have been used to describe patients with excessive fluid intake, but the term primary polydipsia remains the best descriptor because it does not presume any particular cause for the increased fluid intake. Primary polydipsia is often due to a severe mental illness, such as schizophrenia, mania, or an obsessive-compulsive disorder, in which case it is termed psychogenic polydipsia . These patients usually deny true thirst and attribute their polydipsia to bizarre motives, such as a need to cleanse their body of poisons. Studies of a series of polydipsic patients in a psychiatric hospital have shown an incidence as high as 42% of patients with some form of polydipsia and, in most reported cases, there was no obvious explanation for the polydipsia.
However, primary polydipsia can also be caused by an abnormality in the osmoregulatory control of thirst, in which case it has been termed dipsogenic diabetes insipidus. These patients have no overt psychiatric illness and invariably attribute their polydipsia to a nearly constant thirst. Dipsogenic DI is usually idiopathic but can also be secondary to organic structural lesions in the hypothalamus identical to any of the disorders described as causes of CDI, such as neurosarcoidosis of the hypothalamus, tuberculous meningitis, multiple sclerosis, or trauma. Consequently, all polydipsic patients should be evaluated with an MRI scan of the brain before concluding that excessive water intake has an idiopathic or psychiatric cause. Primary polydipsia can also be produced by drugs that cause a dry mouth or by any peripheral disorder causing pathologic elevations of renin and/or angiotensin levels.
Finally, primary polydipsia is sometimes caused by physicians, nurses, or the lay press who advise a high fluid intake for valid (e.g., recurrent nephrolithiasis) or unsubstantiated reasons of health. These patients lack overt signs of mental illness but also deny thirst and usually attribute their polydipsia to habits acquired from years of adherence to their drinking regimen.
Pathophysiology
The pathophysiology of primary polydipsia is essentially the reverse of that in CDI—the excessive intake of water expands and slightly dilutes body fluids, suppresses AVP secretion, and dilutes the urine. The resultant increase in the rate of water excretion balances the increase in intake, and the osmolality of body water stabilizes at a new, slightly lower level that approximates the osmotic threshold for AVP secretion. The magnitude of the polyuria and polydipsia vary considerably, depending on the nature or intensity of the stimulus to drink. In patients with abnormal thirst, the polydipsia and polyuria are relatively constant from day to day. However, in patients with psychogenic polydipsia, water intake and urine output tend to fluctuate widely and at times can be quite large.
Occasionally, fluid intake rises to such extraordinary levels that the excretory capacity of the kidneys is exceeded, and dilutional hyponatremia develops. There is little question that excessive water intake alone can sometimes be sufficient to override renal excretory capacity and produce severe hyponatremia. Although the water excretion rate of normal adult kidneys can generally exceed 20 L/day, maximum hourly rates rarely exceed 1000 mL/hr. Because many psychiatric patients drink predominantly during the day or during intense drinking binges, they can transiently achieve symptomatic levels of hyponatremia, with the total daily volume of water intake less than 20 L if ingested rapidly enough. This likely accounts for many of the patients who present with maximally dilute urine, accounting for as many as 50% of patients in some studies, and are corrected quickly via free water diuresis. The prevalence of this disorder, based on hospital admissions for acute symptomatic hyponatremia, may have been underestimated because studies of polydipsic psychiatric patients have shown a marked diurnal variation in serum [Na + ], from 141 mEq/L at 7 am to 130 mEq/L at 4 pm , suggesting that many such patients drink excessively during the daytime but then correct themselves via water diuresis at night. This and other considerations have led to defining this disorder as the psychosis, intermittent hyponatremia, and polydipsia (PIP) syndrome.
However, many other cases of hyponatremia with psychogenic polydipsia have been found to meet the criteria for a diagnosis of SIADH, suggesting the presence of nonosmotically stimulated AVP secretion. As might be expected, in the presence of much higher than normal water intake, almost any impairment of urinary dilution and water excretion can exacerbate the development of a positive water balance and thereby produce hypo-osmolality. Acute psychosis itself can also cause AVP secretion, which often appears to take the form of a reset osmostat. It is therefore apparent that no single mechanism can completely explain the occurrence of hyponatremia in polydipsic psychiatric patients, but the combination of higher than normal water intake plus modest elevations of plasma AVP levels from a variety of potential sources appears to account for a significant portion of these cases.
Clinical Manifestations of Diabetes Insipidus
The characteristic clinical symptoms of DI are the polyuria and polydipsia that result from the underlying impairment of urine-concentrating mechanisms, which have been described earlier in the sections on the pathophysiology of specific types of DI. Interestingly, patients with DI typically describe a craving for cold water, which appears to quench their thirst better. Patients with CDI also typically describe a precipitous onset of their polyuria and polydipsia, which simply reflects the fact that urinary concentration can be maintained fairly well until the number of AVP-producing neurons in the hypothalamus decreases to 10% to 15% of normal, after which plasma AVP levels decrease to the range at which urine output increases dramatically.
However, patients with DI, particularly those with osmoreceptor dysfunction syndromes, can also present with varying degrees of hyperosmolality and dehydration, depending on their overall hydration status. It is therefore also important to be aware of the clinical manifestations of hyperosmolality. These can be divided into the signs and symptoms produced by dehydration, which are largely cardiovascular, and those caused by the hyperosmolality itself, which are predominantly neurologic and reflect brain dehydration as a result of osmotic water shifts out of the CNS. Cardiovascular manifestations of hypertonic dehydration include hypotension, azotemia, acute tubular necrosis secondary to renal hypoperfusion or rhabdomyolysis, and shock. Neurologic manifestations range from nonspecific symptoms, such as irritability and cognitive dysfunction, to more severe manifestations of hypertonic encephalopathy, such as disorientation, decreased level of consciousness, obtundation, chorea, seizures, coma, focal neurologic deficits, subarachnoid hemorrhage, and cerebral infarction. One study also suggested an increased incidence of deep venous thrombosis in hyperosmolar patients.
The severity of symptoms can be roughly correlated with the degree of hyperosmolality, but individual variability is marked and, for any single patient, the level of serum [Na + ] at which symptoms will appear cannot be accurately predicted. Similar to hypo-osmolar syndromes, the length of time over which hyperosmolality develops can markedly affect the clinical symptomatology. The rapid development of severe hyperosmolality is frequently associated with marked neurologic symptoms, whereas gradual development over several days or weeks generally causes milder symptoms. In this case, the brain counteracts osmotic shrinkage by increasing the intracellular content of solutes. These include electrolytes such as potassium and a variety of organic osmolytes, which previously had been termed idiogenic osmoles; for the most part, these are the same organic osmolytes that are lost from the brain during adaptation to hypo-osmolality. The net effect of this process is to protect the brain against excessive shrinkage during sustained hyperosmolality. However, once the brain has adapted by increasing its solute content, rapid correction of the hyperosmolality can produce brain edema because it takes a finite length of time (24 to 48 hours in animal studies) to dissipate the accumulated solutes and, until this process has been completed, the brain will accumulate excess water as plasma osmolality is normalized. This effect is usually seen in dehydrated pediatric patients who can develop seizures with rapid rehydration, but has been described only rarely in adults, including the most severely hyperosmolar patients with nonketotic hyperglycemic hyperosmolar coma.
Differential Diagnosis of Polyuria
Before beginning involved diagnostic testing to differentiate among the various forms of DI and primary polydipsia, the presence of true hypotonic polyuria should be established by measurement of a 24-hour urine for volume and osmolality. Generally accepted standards are that the 24-hour urine volume should exceed 50 mL/kg BW, with an osmolality lower than 300 mOsm/kg H 2 O. Simultaneously, there should be a determination of whether the polyuria is due to an osmotic agent such as glucose or intrinsic renal disease. Routine laboratory studies and the clinical setting will usually distinguish these disorders; diabetes mellitus and other forms of solute diuresis usually can be excluded by the history, routine urinalysis for glucose, and/or measurement of the solute excretion rate (urine osmolality × 24-hour urine volume [in liters] > 15 mOsm/kg BW/day). There is universal agreement that the diagnosis of DI requires stimulating AVP secretion osmotically and then measuring the adequacy of the secretion by direct measurement of plasma AVP levels or indirect assessment by urine osmolality.
In a patient who is already hyperosmolar, with submaximally concentrated urine (i.e., urine osmolality < 800 mOsm/kg H 2 O), the diagnosis is straightforward and simple; primary polydipsia is ruled out by the presence of hyperosmolality, confirming a diagnosis of DI. CDI can then be distinguished from NDI by evaluating the response to the administration of AVP (5 units SC) or, preferably, of the AVP V 2 receptor agonist desmopressin (1-deamino-8- d -arginine vasopressin [DDAVP], 1 to 2 µg subcutaneously or intravenously). A significant increase in urine osmolality within 1 to 2 hours after injection indicates insufficient endogenous AVP secretion, and therefore CDI, whereas an absent response indicates renal resistance to AVP effects, and therefore NDI. Although conceptually simple, interpretational difficulties can arise because the water diuresis produced by AVP deficiency in CDI produces a washout of the renal medullary concentrating gradient and downregulation of kidney AQP2 water channels (see earlier), so that initial increases in urine osmolality in response to administered AVP or desmopressin are not as great as would be expected. Generally, increases of urine osmolality of more than 50% reliably indicate CDI, and responses of less than 10% indicate NDI, but responses between 10% and 50% are indeterminate. Therefore, plasma AVP levels should be measured to aid in this distinction; hyperosmolar patients with NDI will have clearly elevated plasma AVP levels, whereas those with CDI will have absent (complete) or blunted (partial) AVP responses relative to their plasma osmolality (see Figure 16.11 ). Because it will not be known beforehand which patients will have diagnostic versus indeterminate responses to AVP or desmopressin, a plasma AVP level should be determined prior to AVP or desmopressin administration in patients with hyperosmolality and inadequately concentrated urine without a solute diuresis.
Patients with DI have intact thirst mechanisms, so they usually do not present with hyperosmolality, but rather with a normal plasma osmolality and serum [Na + ] and symptoms of polyuria and polydipsia. In these cases, it is most appropriate to perform a fluid deprivation test. The relative merits of the indirect fluid deprivation test (Miller-Moses test ) versus direct measurement of plasma AVP levels after a period of fluid deprivation has been debated in literature, with substantial pros and cons in support of each of these tests. On the one hand, the standard indirect test has a long track record of making an appropriate diagnosis in the large majority of cases, generally yields interpretable results by the end of the test, and does not require sensitive assays for the notoriously difficult measurement of plasma AVP levels. However, maximum urine concentrating capacity is well known to be variably reduced in all forms of DI, as well as primary polydipsia, and as a result the absolute levels of urine osmolality achieved during fluid deprivation and after AVP administration are reduced to overlapping degrees in patients with partial CDI, partial NDI, and primary polydipsia.
Measurements of basal plasma osmolality or serum [Na + ] are of little use because they also overlap considerably among these disorders. Although association with certain diseases, surgical procedures, or family history often helps differentiate among these disorders, sometimes the clinical setting may not be helpful because certain disorders (e.g., sarcoidosis, tuberculous meningitis, other hypothalamic pathologies) can cause more than one type of DI (see Table 16.2 ). Consequently, a simpler approach that has been proposed is to measure plasma or urine AVP before and during a suitable osmotic stimulus, such as fluid restriction or hypertonic NaCl infusion, and plot the results as a function of the concurrent plasma osmolality or plasma [Na + ] (see Figures 16-12 and 16-13 ). Using a highly sensitive and validated research assay for plasma AVP determinations, this approach has been shown to provide a definitive diagnosis in most cases, provided the final level of plasma osmolality or sodium achieved is above the normal range (>295 mOsm/kg H 2 O or 145 mmol/L, respectively). The diagnostic effectiveness of this approach derives from the fact that the magnitude of the AVP response to osmotic stimulation is not appreciably diminished by chronic overhydration or dehydration. Hence, the relationship of plasma AVP to plasma osmolality is usually within or above normal limits in NDI and primary polydipsia. In most cases, these two disorders can then be distinguished by measuring urine osmolality before and after the dehydration test and relating these values to the concurrent plasma AVP concentration (see Figure 16.12 ). However, because maximal concentrating capacity can be severely blunted in patients with primary polydipsia, it is often better to analyze the relationship under basal nondehydrated conditions when the plasma AVP level is not elevated. Because of the solute diuresis that often ensues following infusion of hypertonic NaCl, measurements of urine osmolality or AVP excretion are unreliable indicators of changes in hormone secretion and are of little or no diagnostic value when this procedure is used to increase osmolality to more than 295 mOsm/kg H 2 O. Given the proven usefulness of the indirect and direct approaches, a combined fluid deprivation test that synthesizes the crucial aspects of both tests can easily be performed ( Table 16.3 ). In many cases, this will allow interpretation of the plasma AVP levels and response to an AVP challenge.
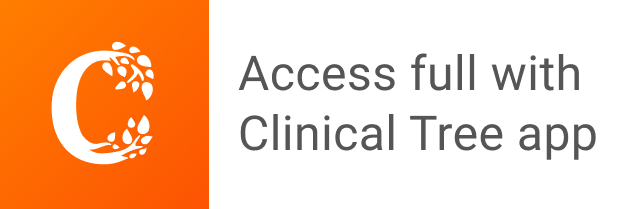