INTRODUCTION
Potassium is found in nearly all food sources. It is the predominant intracellular cation in the body. A high cellular concentration is required to maintain normal function of a number of cellular processes. These include nucleic acid and protein synthesis, regulation of cell volume and pH, cell growth, and enzyme activation. In particular, a high intracellular K+ concentration is necessary for the maintenance of the resting membrane potential. The resting membrane potential, in concert with the threshold membrane potential, sets the stage for generation of the action potential. This process is ultimately required for proper functioning of excitable tissues. Hence, these actions allow normal functioning of cardiac and skeletal muscles. Regulation of K+ homeostasis is achieved mainly through cellular shifts of potassium, as well as renal K+ excretion. These 2 regulatory mechanisms are under the control of a variety of factors that are reviewed in subsequent sections. Disturbances in these homeostatic mechanisms result in either hypokalemia or hyperkalemia. Both of these disturbances in K+ balance promote a variety of clinical symptoms and physical findings that are predominantly caused by disruption of action potential formation, leading to neuromuscular dysfunction and inhibition of normal cell enzymatics. Rapid recognition and treatment of these disorders are required to avoid serious morbidity and mortality.
POTASSIUM HOMEOSTASIS
Total-body K+ stores in an adult are between 3000 and 4000 mEq (50–60 mEq/kg body weight). Total-body K+ content is also influenced by age and sex. As compared with a young male, an elderly man has 20% less totalbody K+ content. Also, age-matched females have 25% less total-body K+ than males. Potassium is readily absorbed from the gastrointestinal (GI) tract and subsequently distributed in cells of muscle, liver, bone, and red blood cells. Maintenance of total-body K+ stores within narrow limits is achieved by zero net balance between input and output, as well as by regulation of K+ between the extracellular fluid (ECF) and intracellular fluid (ICF). The bulk (90%) of dietary potassium is excreted in urine and the rest in feces (10%) in an adult. In contrast to sodium (Na+), K+ is predominantly an intracellular cation, with 98% of body K+ located inside the cell. Hence, only 2% of K+ is present in the ECF. As a result, there is a dramatic difference in K+ concentration intracellularly (145 mEq/L) versus extracellularly (4 to 5 mEq/L). Despite this fact, however, the serum K+ concentration is employed as an index of potassium balance because it is the most readily available clinical test. In general, it is a reasonably accurate reflection of total-body potassium content. In disease states, however, the serum potassium concentration may not always represent total-body K+ stores. The clinician must keep this is mind when assessing patients with abnormal laboratory values.
KEY POINTS
ROLE OF POTASSIUM IN THE RESTING MEMBRANE POTENTIAL
Movement of cations, such as K+ and Na+, into their respective compartments requires active and passive cellular transport mechanisms. The location of K+ and Na+ in their respective fluid compartments is maintained predominantly by the action of the Na+-K+-adenosine triphosphatase (ATPase) pump in the cell membrane. This enzyme hydrolyzes adenosine triphosphate (ATP) to create the energy required to pump Na+ out of the cell and K+ into the cell in a 3:2 ratio. Potassium moves out of the cell at a rate dependent on the electrochemical gradient, this creates the resting membrane potential (E). As seen below, the Goldman-Hodgkin-Katz equation calculates the membrane potential on the inside of the membrane using Na+ and K+. Three factors determine the Em: (a) the electrical charge of each ion; (b) the membrane permeability to each ion; and (c) the concentration of the ion on each side of the membrane. Inserting the intracellular K+ (145) and Na+ (12) concentrations and extracellular K+ (4.0) and Na+ (140) concentrations into the formula results in a resting membrane potential of –90 mV. The cell interior is –90 mV, largely as a result of the movement of K+ out of the cell via the Na+-K+-ATPase pump.
The resting potential sets the stage for membrane depolarization and generation of the action potential. Any change in serum K+ concentration alters the action potential and excitability of the cell. Thus, regulation of K+ distribution must be efficient, because a small movement of K+ from the ICF or ECF results in a potentially fatal change in serum K+ concentration. Physiologic and pathologic factors influence K+ distribution between ICF and ECF.
CELLULAR DISTRIBUTION OF POTASSIUM
Many foods have a high K+ content that can raise serum K+ concentrations, sometimes to levels that significantly disturb cell function and, as a result, are potentially lethal. To maintain the serum K+ concentration within a safe range, cellular movement of K+ is the first response of the body following ingestion of a potassium-rich meal. This is a key feature of K+ homeostatic mechanisms because renal excretion of K+ requires several hours. The critical importance of this process is illustrated in the following case.
Not surprisingly, insulin, which is secreted following a meal to maintain proper glucose balance, is also integral to cellular K+ homeostasis. As such, serum K+ concentration is maintained in the normal range by the physiologic effects of insulin. This role of insulin to move K+ into cells is well suited as renal K+ excretion does not occur immediately following ingestion of a meal containing large amounts of potassium. Movement of K+ into cells allows rapid lowering of the serum K+ concentration until the K+ load is fully excreted by the kidneys. Insulin stimulates K+ uptake into cells by increasing the activity and number of Na+-K+-ATPase pumps in the cell membrane. Two K+ ions are transported into the cell, while 3 Na+ ions are moved out of the cell by this energy-requiring transporter. The intracellular shift of K+ is independent of glucose transport. A deficiency of insulin, as occurs in many patients with type 1 diabetes mellitus, is associated with hyperkalemia from impaired cellular uptake of K+. The following clinical experiment illustrates the effect of insulin on cellular K+ homeostasis.
Infusion of somatostatin, an inhibitor of pancreatic insulin release, in normal subjects reduced basal insulin concentrations to very low levels. Serum K+ concentrations were measured with KCl infusion during baseline, infusion with somatostatin, and infusion with somatostatin plus insulin. An exaggerated rise in serum K+ concentration developed with somatostatin; this effect was completely reversed by insulin infusion.
As noted with insulin, endogenous catecholamines and β2-adrenergic agonists promote K+ movement into cells through stimulation of the Na+-K+-ATPase. Activation of the β2 receptor underlies the effect on this active enzyme pump to move K+ into cells. Receptor activation is signaled through adenylate cyclase to generate cyclic adenosine monophosphate (AMP). This second messenger system ultimately stimulates the Na+-K+-ATPase pump to shift K+ into cells. Medications such as albuterol, a β2-adrenergic agonist used for asthma, can lower serum K+concentration through stimulation of cell uptake, whereas propranolol, an antihypertensive medication that blocks β2-adrenergic receptors, may cause hyperkalemia through inhibition of K+ movement into cells. Intoxication with a medication such as digoxin may raise serum K+ concentration by disrupting the Na+-K+-ATPase, thereby blocking cellular K+ uptake. The clinical observation described below demonstrates the effect of digoxin on Na+-K+-ATPase function and serum K+ concentration.
Other physiologic factors that modulate cellular K+ movement include exercise, changes in extracellular pH, in particular metabolic acidosis and alkalosis, as well as changes in plasma osmolality. Exercise has a dual effect on cellular K+ movement. A transient rise in serum K+ concentration occurs primarily to increase blood flow to muscle. This homeostatic effect occurs because local release of K+ vasodilates vessels and improves perfusion of ischemic muscles (provides more oxygen). A counterbalancing effect of endogenous catecholamine secretion also develops with exercise; this moves K+ back into the ICF (activation of β2-adrenergic receptors) and restores the serum K+ concentration to normal. The level of exercise influences the cellular release of K+. For example, a 0.3- to 0.4-mEq/L rise with slow walking, a 0.7- to 1.2-mEq/L rise with moderate exercise, and as much as a 2-mEq/L rise with exercise to the point of exhaustion. Rest is associated with rapid correction of the rise in serum K+ concentration, mainly through the actions of the Na+-K+-ATPase. Physical conditioning reduces the rise in K+ concentration, presumably through an improvement in pump activity.
Changes in pH also influence serum K+ concentration. Metabolic acidosis is associated with an exit of K+ from cells in exchange for protons (H+) as the cells attempt to buffer the ECF pH. The exchange of K+ for H+ maintains electroneutrality across membranes. In this setting, up to 60% of excess protons are buffered within cells. An opposite effect is observed with metabolic alkalosis as K+ enters the ICF to allow H+ to enter the ECF and reduce alkalemia. In general, the serum K+ concentration increases or decreases by 0.4 mEq/L for every 0.1 decrease or increase in pH. There is a wide variability, however, in the change in serum K+ concentration with pH change in metabolic acidosis (0.2 to 1.7 mEq/L for every 0.1 fall in pH). Furthermore, this effect is more prominent with mineral (nonanion gap) metabolic acidoses than organic anion acidoses. The explanation for the differential effects of these types of acute acidoses on cellular K+ movement is based on the presence of different types of ion transporters in cell membranes. In mineral metabolic acidosis, multiple ion transporters work in a coordinated fashion to buffer extracellular acidosis. The fall in extracellular pH inhibits both the Na+-H+-antiporter activity and Na+–, which causes a slowing of Na+-K+-ATPase and a reduction in cellular K+ uptake. In addition,
-Cl– exchanger activity, which is increased to buffer extracellular H+, is coupled to Cl+-K+-cotransport. This results in increased movement of K from the cell to extracellular space. These buffering pathways for acute mineral metabolic acidosis lead to increased extracellular K+ and hyperkalemia. In the setting of metabolic alkalosis, K+ moves in the opposite direction and hypokalemia can develop from intracellular shift. In contrast, in the setting of organic acidosis (lactic acidosis), K movement into and out of the cell is balanced. For example, the decline in extracellular pH increases extracellular K through the mechanisms discussed for mineral acidosis except that a strong influx of H+ and lactate occurs through the monocarboxylate (H+-A–) transporter, resulting in a larger fall in intracellular pH and
. This tends to increase intracellular Na+ via Na+-H+-antiporter and Na+–
cotransporter, stimulating Na+-K+-ATPase and movement of K+ into cells. These effects tend to have no net change in extracellular K and no clinically significant hyperkalemia.
An increase in plasma osmolality, as occurs with hyperglycemia in diabetes mellitus, raises serum K+ concentration as a result of a shift of K+ out of cells. Potassium movement from cells is induced by the increase in intracellular K+ concentration that occurs as water leaves the cell. As the intracellular K+ concentration rises, an increased driving force for passive diffusion of K+ out of the cell develops and extracellular K+ increases. In general, the serum K+ concentration rises by 0.4 to 0.8 mEq/L for every 10 mOsm/kg increase in the effective osmolality. As will be discussed later, other hyperosmolar substances can cause a shift of K+ out of cells. There exists a small amount of data suggesting that aldosterone may increase cellular uptake of K+ through stimulation of the Na+-K+-ATPase pump. The role of aldosterone on cellular K+ movement, however, is controversial, and probably of only minor importance. As is noted later, the major effect of aldosterone is to enhance renal K+ excretion.
KEY POINTS
POTASSIUM HANDLING BY THE KIDNEY
Proximal Tubule
K+ handling in kidney occurs through the processes of glomerular filtration and both tubular reabsorption and secretion. In proximal nephron, 100% of K+ reaches the tubule as K+ is freely filtered by the glomerulus. Approximately 60% to 80% of filtered K+ is reabsorbed by proximal tubule. Uptake of K+ occurs via passive rather than active transport mechanisms. Potassium is reabsorbed by a K+ transporter and through paracellular pathways coupled with Na+ and water. Any process that affects Na+ and water movement in proximal tubule will also influence K+ reabsorption. For example, volume depletion will increase Na+ and water reabsorption, also increasing K+ uptake, whereas volume expansion will inhibit passive diffusion of Na+-coupled K+ transport.
Loop of Henle
In the loop of Henle, K+ is both secreted and reabsorbed. Ultimately, 25% of the filtered K+ is reabsorbed in this nephron segment. Potassium is secreted into the lumen and the K+ concentration at the tip of the loop of Henle may exceed the amount filtered. In contrast, K+ is actively and passively reabsorbed in the medullary thick ascending limb. Active K+ transport occurs by the 1Na+-1K+-2Cl– cotransporter (Figure 6.1), which is powered by the enzymatic activity of Na+-K+-ATPase on the basolateral membrane. Secondary active cotransport is driven by the steep Na+ gradient across the apical membrane created by this enzyme pump. To allow continued cotransport, K+ must recycle across the apical membrane from the cell into the tubular lumen. This provides a continuous supply of K+ ions for cotransport with Na+ and Cl–, and negates the limiting effect of low luminal K+. Medications such as loop diuretics and certain genetic disorders impair the transport function of this cotransporter resulting in Na+ and K+ wasting.
FIGURE 6-1. Na+-K+-ATPase. The Na+-K+-ATPase on the basolateral membrane provides the energy required to drive secondary active K+ transport by the 1Na+-1K+-2Cl– cotransporter in the thick ascending limb of Henle.
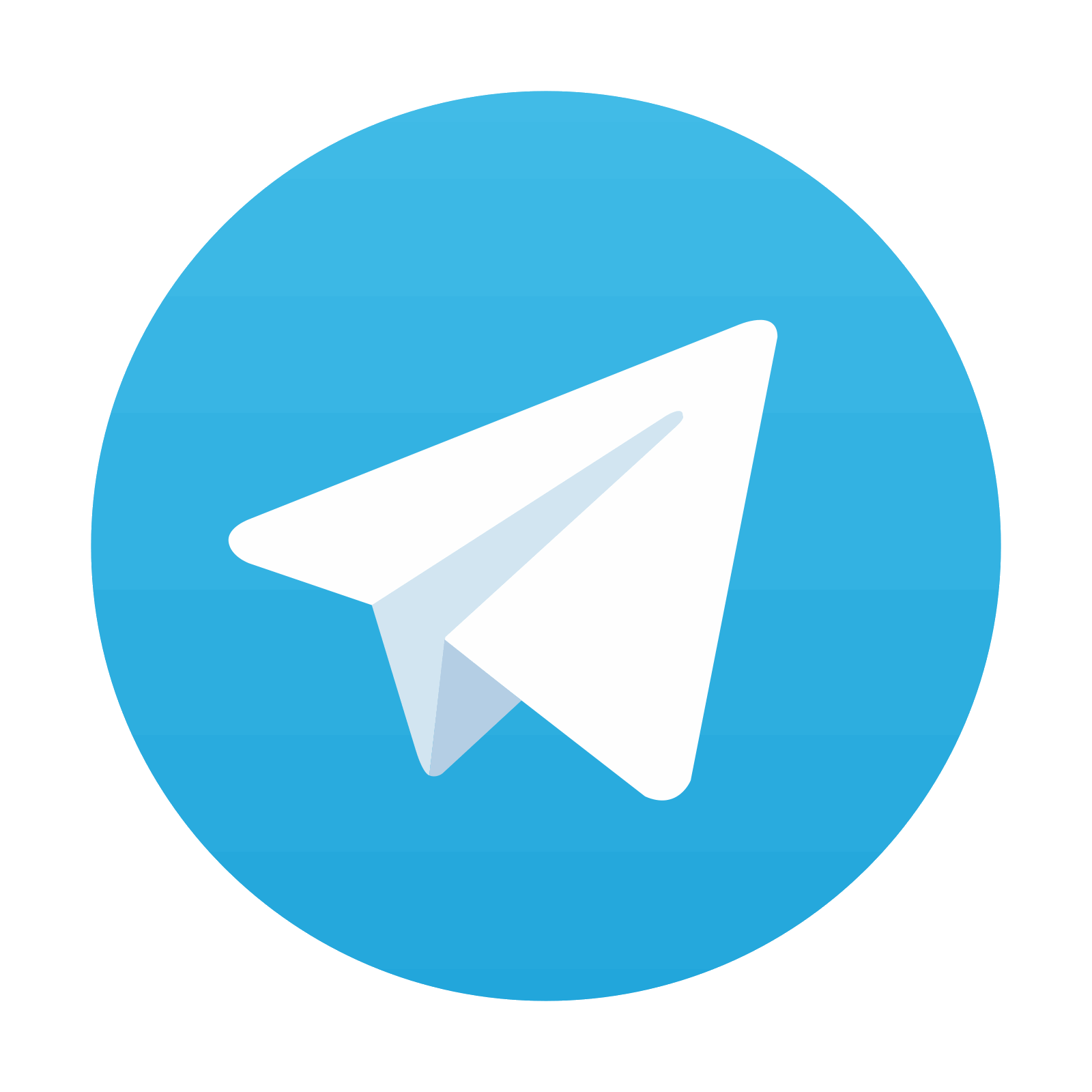
Stay updated, free articles. Join our Telegram channel
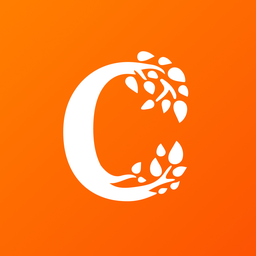
Full access? Get Clinical Tree
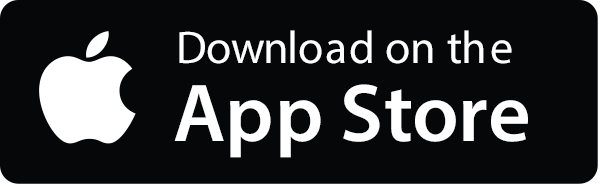
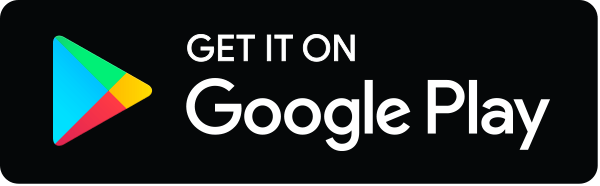