Chapter Outline
NORMAL POTASSIUM BALANCE, 559
RENAL POTASSIUM EXCRETION, 563
Potassium Transport in the Distal Nephron, 563
Control of Potassium Secretion, 565
Integrated Regulation of Distal Sodium Absorption and Potassium Secretion, 566
Regulation of Renal Renin and Adrenal Aldosterone, 568
Urinary Indices of Potassium Excretion, 569
CONSEQUENCES OF HYPOKALEMIA AND HYPERKALEMIA, 569
HYPOKALEMIA, 572
Epidemiology, 572
Spurious Hypokalemia, 572
Redistribution and Hypokalemia, 572
Hypokalemic Periodic Paralysis, 573
Potassium Loss, 574
Clinical Approach to Hypokalemia, 581
Treatment of Hypokalemia, 583
HYPERKALEMIA, 585
Epidemiology, 585
Pseudohyperkalemia, 585
Excess Intake of Potassium and Tissue Necrosis, 586
Redistribution and Hyperkalemia, 586
Reduced Renal Potassium Excretion, 588
Medication-Related Hyperkalemia, 590
Clinical Approach to Hyperkalemia, 592
Treatment of Hyperkalemia, 592
The diagnosis and management of potassium disorders are central skills in clinical nephrology, relevant not only to consultative nephrology but also to dialysis and renal transplantation. An understanding of the underlying physiology is critical to the diagnostic and management approach to hyperkalemic and hypokalemic patients. This chapter reviews those aspects of the physiology of potassium homeostasis judged to be relevant to the understanding of potassium disorders; a more detailed review of renal potassium transport is provided in Chapter 6 .
The pathophysiology of potassium disorders continues to evolve. The expanding list of drugs with a potential to affect plasma potassium concentration (K + ) has complicated clinical management and provided new insights. In addition, the evolving molecular understanding of rare disorders affecting plasma K + has uncovered novel pathways of regulation ; whereas none of these disorders constitutes a public health menace, they are experiments of nature that have provided new windows on critical aspects of potassium homeostasis. These advances can be incorporated into an increasingly mechanistic, molecular understanding of potassium disorders.
Normal Potassium Balance
The dietary intake of potassium ranges from less than 35 to more than 110 mmol/day in U.S. adults. Despite this widespread variation in intake, homeostatic mechanisms serve to maintain plasma K + precisely between 3.5 and 5.0 mmol/L. In a healthy individual at steady state, the entire daily intake of potassium is excreted, approximately 90% in the urine and 10% in the stool. More than 98% of total body potassium is intracellular, chiefly in muscle ( Figure 18.1 ). Buffering of extracellular K + by this large intracellular pool plays a crucial role in the regulation of plasma K + . Thus, within 60 minutes of an intravenous load of 0.5 mmol/kg of K + -Cl − , only 41% appears in the urine, yet serum K + rises by no more than 0.6 mmol/L ; adding the equivalent 35 mmol exclusively to the extracellular space of a 70-kg man would be expected to raise serum K + by ~2.5 mmol/L. Changes in cellular distribution also serve to defend plasma K + during K + depletion. For example, military recruits have been shown to maintain a normal serum K + after 11 days of basic training, despite a profound K + deficit generated by renal and extrarenal loss. The rapid exchange of intracellular K + with extracellular K + plays a crucial role in maintaining plasma K + within such a narrow range; this is accomplished by overlapping and synergistic regulation of a number of renal and extrarenal transport pathways.

Potassium Transport Mechanisms
The intracellular accumulation of K + against its electrochemical gradient is an energy-consuming process, mediated by the ubiquitous Na + -K + -ATPase enzyme. The Na + -K + -ATPase functions as an electrogenic pump, since the stoichiometry of transport is three intracellular Na + ions to two extracellular K + ions. The enzyme complex is made up of a tissue-specific combination of multiple α-, β-, and γ-subunits, which are further subject to tissue-specific patterns of regulation. The Na + -K + -ATPase proteins share significant homology with the corresponding subunits of the H + -K + -ATPase enzymes (see “ Potassium Transport in the Distal Nephron ”). Cardiac glycosides—digoxin and ouabain—bind to the α-subunits of Na + -K + -ATPase at an exposed extracellular hairpin loop that also contains the major binding sites for extracellular K + . The binding of digoxin and K + to the Na + -K + -ATPase complex is thus mutually antagonistic, explaining in part the potentiation of digoxin toxicity by hypokalemia. Although the four α-subunits have equivalent affinity for ouabain, they differ significantly in intrinsic K + -ouabain antagonism. Ouabain binding to isozymes containing the ubiquitous α 1 -subunit is relatively insensitive to K + concentrations within the physiologic range, such that this isozyme is protected from digoxin under conditions wherein cardiac α 2 – and α 3 -subunits, the probable therapeutic targets, are inhibited. Genetic reduction in cardiac α 1 -subunit content has a negative ionotropic effect, such that the relative resistance of this subunit to digoxin at physiologic plasma K + has an additional cardioprotective effect. Notably, the digoxin-ouabain binding site of α-subunits is highly conserved, suggesting a potential role in the physiologic response to endogenous ouabain and digoxin-like compounds. Novel knock-in mice have been generated that express α 2 -subunits with engineered resistance to ouabain. These mice are strikingly resistant to ouabain-induced hypertension and to adrenocorticotropic hormone (ACTH)–dependent hypertension, the latter known to involve an increase in circulating ouabain-like glycosides. This provocative data have given more credence to the controversial role of such ouabain-like molecules in hypertension and cardiovascular disease. Furthermore, modulation of the K + -dependent binding of circulating ouabain-like compounds to Na + -K + -ATPase may underlie at least some of cardiovascular complications of hypokalemia (see “ Consequences of Hypokalemia ”).
Skeletal muscle contains as much as 75% of body potassium (see Figure 18.1 ), and exerts considerable influence on extracellular K + . Exercise is thus a well-described cause of transient hyperkalemia; interstitial K + in human muscle can reach levels as high as 10 mmol/L after fatiguing exercise. Not surprisingly, therefore, changes in skeletal muscle Na + -K + -ATPase activity and abundance are major determinants of the capacity for extrarenal K + homeostasis. Hypokalemia induces a marked decrease in muscle K + content and Na + -K + -ATPase activity, an apparently altruistic mechanism to regulate plasma K + . This is primarily due to dramatic decreases in the protein abundance of the α 2 -subunit of Na + /K + -ATPase. In contrast, hyperkalemia due to potassium loading is associated with adaptive increases in muscle K + content and Na + -K + -ATPase activity. These interactions are reflected in the relationship between physical activity and the ability to regulate extracellular K + during exercise. For example, exercise training is associated with increases in muscle Na + -K + -ATPase concentration and activity, with reduced interstitial K + in trained muscles and an enhanced recovery of plasma K + after defined amounts of exercise.
Potassium can also accumulate in cells by coupling to the gradient for Na + entry, entering via the electroneutral Na + -K + -2Cl − cotransporters NKCC1 and NKCC2. The NKCC2 protein is found only at the apical membrane of the thick ascending limb (TAL) and macula densa cells ( Figure 18.2 ; see Figure 18.10 ), where it functions in transepithelial salt transport and tubular regulation of renin release. In contrast, NKCC1 is widely expressed in multiple tissues, including muscle. The cotransport of K + -Cl − by the four K + -Cl − cotransporters (KCC1-4) can also function in the transfer of K + across membranes; although the KCCs typically function as efflux pathways, they can mediate influx when extracellular K + increases.

The efflux of K + out of cells is largely accomplished by K + channels, which comprise the largest family of ion channels in the human genome. There are three major subclasses of mammalian K + channels: the six-transmembrane domain (TMD) family, which encompasses the voltage-sensitive and Ca 2+ -activated K + channels; the two-pore, four-TMD family; and the two-TMD family of inward rectifying K + (Kir) channels. There is tremendous genomic variety in human K + channels, with at least 26 separate genes encoding principal subunits of the voltage-gated Kv channels and 16 genes encoding the principal Kir subunits. Further complexity is generated by the presence of multiple accessory subunits and alternative patterns of messenger RNA (mRNA) splicing. Not surprisingly, an increasing number and variety of K + channels have been implicated in the control of K + homeostasis and the membrane potential of excitable cells, such as muscle and heart, with important and evolving roles in the pathophysiology of potassium disorders.
Factors Affecting Internal Distribution of Potassium
A number of hormones and physiologic conditions have acute effects on the distribution of K + between the intracellular and extracellular spaces ( Table 18.1 ). Some of these factors are of particular clinical relevance and are therefore reviewed here in detail.
Factor | Effect |
---|---|
Acute: Effect on Potassium | |
Insulin | Enhanced cell uptake |
β-Catecholamines | Enhanced cell uptake |
α-Catecholamines | Impaired cell uptake |
Acidosis | Impaired cell uptake |
Alkalosis | Enhanced cell uptake |
External potassium balance | Loose correlation |
Cell damage | Impaired cell uptake |
Hyperosmolality | Enhanced cell efflux |
Chronic: Effect on ATP Pump Density | |
Thyroid | Enhanced |
Adrenal steroids | Enhanced |
Exercise (training) | Enhanced |
Growth | Enhanced |
Diabetes | Impaired |
Potassium deficiency | Impaired |
Chronic renal failure | Impaired |
Insulin
The hypokalemic effect of insulin has been known since the early twentieth century. The impact of insulin on plasma K + and plasma glucose is separable at multiple levels, suggesting independent mechanisms. For example, despite impaired glucose uptake, peripheral K + uptake is not impaired in humans with type 2 diabetes. Notably, the hypokalemic effect of insulin is not renal-dependent. Insulin and K + appear to form a feedback loop of sorts, in that increases in plasma K + have a marked stimulatory effect on insulin levels. Insulin-stimulated K + uptake, measured in rats using a K + clamp technique, is rapidly reduced by 2 days of K + depletion, prior to a modest drop in plasma K + , and in the absence of a change in plasma K + in rats subject to a lesser K + restriction for 14 days. Insulin-mediated K + uptake is thus modulated by the factors that serve to preserve plasma K + in the setting of K + deprivation (see also “ Control of Potassium Secretion: Effect of Potassium Intake ”). Inhibition of basal insulin secretion in normal subjects by somatostatin infusion increases serum K + by up to 0.5 mmol/L in the absence of a change in urinary excretion, emphasizing the crucial role of circulating insulin in the regulation of plasma K + . Clinically, inhibition of insulin secretion by the somatostatin agonist octreotide can cause significant hyperkalemia in anephric patients and patients with normal renal function.
Insulin stimulates the uptake of K + by several tissues, most prominently liver, skeletal muscle, cardiac muscle, and fat. It does so by activating several K + transport pathways, with particularly well-documented effects on the Na + -K + -ATPase. Insulin activates Na + -H + exchange and/or Na + -K + -2Cl − cotransport in several tissues; although the ensuing increase in intracellular Na + was postulated to have a secondary activating effect on Na + -K + -ATPase, it is clear that this is not the primary mechanism in most cell types. Insulin induces translocation of the Na + -K + -ATPase α 2 -subunit to the plasma membrane of skeletal muscle cells, with a lesser effect on the α 1 -subunit. This translocation is dependent on the activity of phosphatidylinositol-3-kinase (PI3K), which itself also binds to a proline-rich motif in the N terminus of the α-subunit. The activation of PI3K by insulin thus induces phosphatase enzymes to dephosphorylate a specific serine residue adjacent to the PI3K binding domain. Trafficking of Na + -K + -ATPase to the cell surface also appears to require the phosphorylation of an adjacent tyrosine residue, perhaps catalyzed by the tyrosine kinase activity of the insulin receptor itself. Finally, the serum- and glucocorticoid-regulated kinase-1 (SGK1) plays a critical role in insulin-stimulated K + uptake, presumably via the known stimulatory effects of this kinase on Na + -K + -ATPase activity and/or Na + -K + -2Cl − cotransport. The hypokalemic effect of insulin plus glucose is blunted in SGK1 knockout mice, with a marked reduction in hepatic insulin-stimulated K + uptake.
Sympathetic Nervous System
The sympathetic nervous system plays a prominent role in regulating the balance between extracellular and intracellular K + . Again, as is the case for insulin, the effect of catecholamines on plasma K + has been known since the 1930s ; however, a complicating issue is the differential effect of stimulating α- and β-adrenergic receptors ( Table 18.2 ). Uptake of K + by liver and muscle, with resultant hypokalemia, is stimulated via β 2 -receptors. The hypokalemic effect of catecholamines appears to be largely independent of changes in circulating insulin and has been reported in nephrectomized animals. The cellular mechanisms whereby catecholamines induce K + uptake in muscle include an activation of the Na + -K + -ATPase, likely via increases in cyclic adenosine monophosphate (cAMP). However, β-adrenergic receptors in skeletal muscle also activate the inwardly directed Na + -K + -2Cl − cotransporter NKCC1, which may account for as much as one third of the uptake response to catecholamines.
Catecholamine Specificity | Sustained Effect on Serum K + |
---|---|
β 1 – + β 2 -Agonist (epinephrine, isoproterenol) | Decreased |
Pure β 1 -agonist (ITP) | None |
Pure β 2 -agonist (salbutamol, soterenol, terbutaline | Decreased |
β 1 – + β 2 -Antagonist (propranolol, sotalol) | Increased; blocks the effect of β-agonists |
β 1 -Antagonist (practolol, metoprolol, atenolol) | None; does not block effect of β-agonists |
β 2 -Antagonist (butoxamine, H 35/25) | Blocks hypokalemic effect of β-agonists |
α-Agonist (phenylephrine) | Increased |
α-Antagonist (phenoxybenzamine) | None; blocks effect of α-agonists |
In contrast to β-adrenergic stimulation, α-adrenergic agonists impair the ability to buffer increases in K + induced via intravenous loading or by exercise ; the cellular mechanisms whereby this occurs are not known. It is thought that β-adrenergic stimulation increases K + uptake during exercise to avoid hyperkalemia, whereas α-adrenergic mechanisms help blunt the ensuing postexercise nadir. The clinical consequences of the sympathetic control of extrarenal K + homeostasis are reviewed elsewhere in this chapter.
Acid-Base Status
The association between changes in pH and plasma K + was observed in 1934. It has long been thought that acute disturbances in acid-base equilibrium result in changes in serum K + , such that alkalemia shifts K + into cells, whereas acidemia is associated with K + release. It is thought that this effective K + -H + exchange serves to help maintain extracellular pH. Rather limited data exist for the durable concept that a change of 0.1 unit in serum pH will result in a 0.6-mmol/L change in serum K + in the opposite direction. However, despite the complexities of changes in K + homeostasis associated with various acid-base disorders, a few general observations can be made. The induction of metabolic acidosis by the infusion of mineral acids (NH 4 + -Cl − or H + -Cl − ) consistently increases serum K + , whereas organic acidosis generally fails to increase serum K + . Notably, another study failed to detect an increase in serum K + in normal human subjects with acute acidosis secondary to duodenal NH 4 + -Cl − infusion, in which a modest acidosis was accompanied by an increase in circulating insulin. However, as noted by Adrogué and Madias, the concomitant infusion of 350 mL of 5% dextrose in water (D 5 W) in these fasting subjects may have served to increase circulating insulin, thus blunting the potential hyperkalemic response to NH 4 + -Cl − . Clinically, use of the oral phosphate binder sevelamer hydrochloride in patients with end-stage kidney disease (ESKD) is associated with acidosis due to effective gastrointestinal absorption of H + -Cl − ; in hemodialysis patients, this acidosis has been associated with an increase in serum K + , which is ameliorated by an increase in dialysis bicarbonate concentration. Of note, hyperkalemia is not an expected complication of sevelamer carbonate, which has supplanted sevelamer hydrochloride as a phosphate binder. Metabolic alkalosis induced by sodium bicarbonate infusion usually results in a modest reduction in serum K + . Respiratory alkalosis reduces plasma K + by a magnitude comparable to that of metabolic alkalosis. Finally, acute respiratory acidosis increases plasma K + ; the absolute increase is smaller than that induced by metabolic acidosis secondary to inorganic acids. Again, however, some studies have failed to show a change in serum K + following acute respiratory acidosis.
Renal Potassium Excretion
Potassium Transport in the Distal Nephron
The proximal tubule and loop of Henle mediate the bulk of potassium reabsorption, such that a considerable fraction of filtered potassium is reabsorbed prior to entry into the superficial distal tubules. Renal potassium excretion is primarily determined by regulated secretion in the distal nephron, specifically within the connecting segment (CNT) and cortical collecting duct (CCD). The principal cells of the CNT and CCD play a dominant role in K + secretion; the relevant transport pathways are shown in Figures 18-2 and 18-3 . Apical Na + entry via the amiloride-sensitive epithelial Na + channel (ENaC) results in the generation of a lumen-negative potential difference in the CNT and CCD, which drives passive K + exit through apical K + channels. A critical, clinically relevant consequence of this relationship is that K + secretion is dependent on delivery of adequate luminal Na + to the CNT and CCD ; K + secretion by the CCD essentially ceases as luminal Na + drops below 8 mmol/L. Selective increases in thiazide-sensitive Na + -Cl − cotransport in the distal convoluted tubule (DCT), as seen in familial hyperkalemia with hypertension (FHHt; see “ Hyperkalemia: Hereditary Tubular Defects and Potassium Excretion ”), reduce Na + delivery to principal cells in the downstream CNT and CCD, leading to hyperkalemia. Dietary Na + intake also influences K + excretion, such that excretion is enhanced by excess Na + intake and reduced by Na + restriction ( Figure 18.4 ). Basolateral exchange of Na + and K + is mediated by the Na + -K + -ATPase, providing the driving force for Na + entry and K + exit at the apical membrane (see Figures 18-2 and 18-3 ).


Under basal conditions of high Na + -Cl − and low K + intake, the bulk of aldosterone-stimulated Na + and K + transport occurs in the CNT, prior to the entry of tubular fluid into the CCD. The density of Na + and K + channels is thus considerably greater in the CNT than in the CCD ; the capacity of the CNT for Na + reabsorption may be as much as 10 times greater than that of the CCD. The recruitment of ENaC subunits in response to dietary Na + restriction begins in the CNT, with progressive recruitment of subunits to the apical membrane of the CCD at lower levels of dietary Na + . The activity of secretory K + channels in the CNT is also influenced by changes in dietary K + ; again, this is consistent with progressive axial recruitment of transport capacity for the absorption of Na + and secretion of K + along the distal nephron.
Electrophysiologic characterization has documented the presence of several subpopulations of apical K + channels in the CCD and CNT, most prominently a small-conductance (SK), 30-picosiemens (pS) channel and a large-conductance, Ca 2+ -activated 150-pS (BK) channel (see Figure 18.3 ). The SK channel is thought to mediate K + secretion under baseline conditions, hence its designation as the secretory K + channel. SK channel activity is mediated by the ROMK ( r enal o uter m edullary K + ) channel protein, encoded by the Kcnj1 gene; targeted deletion of this gene in mice results in complete loss of SK activity within the CCD. Increased distal flow has a significant stimulatory effect on K + secretion, due in part to enhanced delivery and absorption of Na + and to increased removal of secreted K + . The apical Ca 2+ -activated BK channel plays a critical role in flow-dependent K + secretion by the CNT and CCD. BK channels have a heteromeric structure, with α-subunits that form the ion channel pore and modulatory β-subunits. The β 1 -subunits of BK channels are restricted to principal cells within the CNT, whereas β 4 -subunits are detectable at the apical membranes of TAL, DCT, and intercalated cells. Flow-dependent K + secretion is reduced in mice with targeted deletion of the α 1 – and β 1 -subunits, consistent with a dominant role for BK channels.
In addition to apical K + channels, considerable evidence implicates apical K + -Cl − cotransport in distal K + secretion. Pharmacologic studies of perfused tubules are consistent with K + -Cl − cotransport mediated by the KCC proteins. A provocative study has underlined the importance of ENaC-independent K + excretion, whether it is mediated by apical K + -Cl − cotransport and/or by other mechanisms. Rats were infused with amiloride via osmotic minipumps, generating urinary concentrations considered sufficient to inhibit more than 98% of ENaC activity. Whereas amiloride almost abolished K + excretion in rats with normal K + intake, acute and long-term high K + diets led to an increasing fraction of K + excretion that was independent of ENaC activity (~50% after 7 to 9 days on a high-K + diet).
In addition to secretion, the distal nephron is capable of considerable reabsorption of K + , particularly during restriction of dietary K + . This reabsorption is accomplished primarily by intercalated cells in the outer medullary collecting duct (OMCD) via the activity of apical H + -K + -ATPase pumps (see Figure 18.2 ). The molecular physiology of H + -K + -ATPase–mediated K + reabsorption is reviewed in Chapter 6 .
Control of Potassium Secretion
Aldosterone
Aldosterone is well established as an important regulatory factor in K + excretion, and increases in plasma K + are an important stimulus for aldosterone secretion (see also “ Regulation of Renal Renin and Adrenal Aldosterone ”). However, an increasingly dominant theme is that aldosterone plays a permissive and synergistic role in K + homeostasis. This is reflected clinically in the frequent absence of hyperkalemia or hypokalemia in disorders associated with a deficiency or an overabundance of circulating aldosterone, respectively (see “ Hyperaldosteronism ” and “ Hypoaldosteronism ”). Regardless, it is clear that aldosterone and downstream effectors of this hormone have clinically relevant effects on plasma K + levels and that the ability to excrete K + is modulated by systemic aldosterone levels (see Figure 18.4 ).
Aldosterone has no effect on the density of apical SK channels in the CCD or CNT; rather, the hormone induces a marked increase in the density of apical Na + channels, thus increasing the driving force for apical K + excretion. The apical, amiloride-sensitive ENaC is comprised of three subunits, α-, β-, and γ-, that assemble together to traffic synergistically to the cell membrane and mediate Na + transport. Aldosterone activates ENaC channel complexes by multiple mechanisms. First, it induces transcription of the α-ENaC subunit, increasing the availability for co-assembly with the more abundant β- and γ-subunits. Second, aldosterone and dietary Na + -Cl − restriction stimulate a significant redistribution of ENaC subunits in the CNT and early CCD, from a largely cytoplasmic location during dietary Na + -Cl − excess to a purely apical distribution after aldosterone or Na + -Cl − restriction. Third, aldosterone induces the expression of a serine-threonine kinase called SGK-1 (serum and glucocorticoid-induced kinase-1) ; coexpression of SGK-1 with ENaC subunits results in increased expression at the plasma membrane. SGK-1 modulates membrane expression of ENaC by interfering with regulated endocytosis of its channel subunits. Specifically, the kinase interferes with interactions between ENaC subunits and the ubiquitin ligase Nedd4-2. The so-called PPxY domains in the C termini of all three ENaC subunits bind to WW domains of Nedd4-2 ; these PPxY domains are deleted, truncated, or mutated in patients with Liddle’s syndrome (see later, “ Liddle’s Syndrome ”), leading to a gain of function in channel activity. Nedd4-2 ubiquitinates ENaC subunits, thus inducing removal of channel subunits from the cell membrane. followed by degradation in lysosomes and the proteasome. A PPxY domain in SGK-1 also binds to Nedd4-2, which is a phosphorylation substrate for the kinase; phosphorylation of Nedd4-2 by SGK-1 abrogates the inhibitory effect of this ubiquitin ligase on ENaC subunits ( Figure 18.5 ).

The importance of SGK-1 in K + and Na + homeostasis is illustrated by the phenotype of SGK-1 knockout mice. On a normal diet, homozygous SGK-1 −/− mice exhibit normal blood pressure and a normal plasma K + , with only a mild elevation of circulating aldosterone. However, dietary Na + -Cl − restriction of these mice results in relative Na + wasting and hypotension, marked weight loss, and a drop in the glomerular filtration rate (GFR), despite considerable increases in circulating aldosterone. In addition, dietary K + loading over 6 days leads to a 1.5-mmol/L increase in serum K + , also accompanied by a considerable increase in circulating aldosterone (~fivefold greater than that of wild-type littermate controls). This hyperkalemia occurs despite evident increases in apical ROMK expression, compared to the normokalemic littermate controls. The amiloride-sensitive, lumen-negative potential difference generated by ENaC is reduced in these SGK-1 knockout mice, resulting in a decreased driving force for distal K + secretion and the observed susceptibility to hyperkalemia.
Another mechanism whereby aldosterone activates ENaC involves proteolytic cleavage of the channel proteins by serine proteases. A channel-activating protease that increases channel activity of ENaC was initially identified in Xenopus laevis A6 cells. The mammalian ortholog, denoted CAP1 (channel-activating protease-1), or prostasin, is an aldosterone-induced protein in principal cells. Urinary excretion of CAP1 is increased in hyperaldosteronism, with a reduction after adrenalectomy. CAP1 is membrane-associated via a glycosylphosphatidylinositol (GPI) linkage ; mammalian principal cells also express two transmembrane proteases, denoted CAP2 and CAP3, with homology to CAP1. These and other proteases (e.g., furin, plasmin) activate ENaC by excising extracellular inhibitory domains from the α- and γ-subunits, increasing the open probability of channels at the plasma membrane. This proteolytic cleavage of ENaC appears to activate the channel by removing the so-called self-inhibitory effect of external Na + ; in the case of furin-mediated proteolysis of α-ENaC, this appears to involve the removal of an inhibitory domain from within the extracellular loop. Extracellular Na + appears to interact with a specific acidic cleft in the cleaved extracellular loop of α-ENaC, causing inhibition of the channel. Since SGK-1 increases channel expression at the cell surface, one would expect synergistic activation by coexpressed CAP1-3 and SGK, and this is indeed the case. Therefore, aldosterone activates ENaC by at least three separate synergistic mechanisms—induction of α-ENaC, induction of SGK-1 and repression of Nedd4-2, and induction of channel-activating proteases. Clinically, the inhibition of channel-activating proteases by the protease inhibitor nafamostat causes hyperkalemia due to inhibition of ENaC activity.
Effect of Potassium Intake
Changes in K + intake strongly modulate K + channel activity in the CNT and CCD (secretory capacity), in addition to H + -K + -ATPase activity in the OMCD (reabsorptive capacity). Increased dietary K + rapidly increases the activity of SK channels in the CCD and CNT, along with a modest increase in Na + channel (ENaC) activity ; this is associated with an increase in apical expression of the ROMK channel protein. The increase in ENaC and SK channel density in the CCD occurs within hours of intake of a high-K + diet, with a minimal associated increase in circulating aldosterone. BK channels in the CNT and CCD are also activated by dietary K + loading. Trafficking of BK subunits is thus affected by dietary K + , with largely intracellular distribution of α-subunits in K + -restricted rats and prominent apical expression in K + -loaded rats. Again, aldosterone does not contribute to the regulation of BK channel activity or expression in response to a high-K + diet.
A complex synergistic mix of signaling pathways regulates K + channel activity in response to changes in dietary K + (see also Chapter 6 ). In particular, the WNK ( w ith n o l ysine) kinases play a critical role in modulating distal K + secretion. WNK1 and WNK4 were initially identified as the causative genes for FHHt (see also “ Hyperkalemia: and Potassium Excretion ”). ROMK expression at the membrane of Xenopus oocytes is reduced by coexpression of WNK4; FHHt-associated mutations increase this effect, suggesting a direction inhibition of SK channels in FHHt. Transcription of the WNK1 gene generates several different isoforms; the predominant intrarenal WNK1 isoform is generated by a distal nephron transcriptional site that bypasses the N-terminal exons that encode the kinase domain, yielding a kinase-deficient short isoform (“WNK1-S”). Full-length WNK1 (WNK1-L) inhibits ROMK by inducing endocytosis of the channel protein; the shorter, kinase-deficient WNK1-S isoform inhibits this effect of WNK1-L. The ratio of WNK1-S to WNK1-L transcripts is reduced by K + restriction (greater endocytosis of ROMK) and increased by K + loading (reduced endocytosis of ROMK), suggesting that this ratio between WNK1-S and WNK1-L functions as a molecular switch to regulate distal K + secretion. The BK channel is also regulated by the WNK kinases.
The membrane trafficking of ROMK is also modulated by tyrosine phosphorylation of the channel protein, such that tyrosine phosphorylation stimulates endocytosis, and tyrosine dephosphorylation induces exocytosis. Intrarenal activity of the cytoplasmic tyrosine kinases c-src and c-yes is inversely related to dietary K + intake, with a decrease under high K + conditions and a marked increase after several days of K + restriction. Several studies have implicated the intrarenal generation of superoxide anions in the activation of cytoplasmic tyrosine kinases by K + depletion. Potential candidates for the upstream hormonal signals include angiotensin II (Ang II) and growth factors such as insulin-like growth factor-1 (IGF-1). In particular, Ang II inhibits ROMK activity in K + -restricted rats, but not rats on a normal K + diet. This inhibition by Ang II involves downstream activation of superoxide production and c-src activity, such that the induction of Ang II by a low-K + diet appears to play a major role in reducing distal tubular K + secretion.
Integrated Regulation of Distal Sodium Absorption and Potassium Secretion
Under certain physiologic conditions associated with marked induction of aldosterone, such as dietary sodium restriction, Na + balance can be maintained without significant effects on K + excretion. However, by activating ENaC and generating a more lumen-negative potential difference, increases in aldosterone should lead to an obligatory kaliuresis. How is this physiologic consequence avoided? The mechanisms that underlie this aldosterone paradox, the independent regulation of Na + and K + handling by the aldosterone-sensitive distal nephron, have been delineated. The major factors that allow for integrated but independent control of Na + and K + transport appear to include electroneutral thiazide-sensitive Na + -Cl − transport within the CCD, ENaC-independent K + excretion within the distal nephron, and differential regulation of various signaling pathways by aldosterone, Ang II, and dietary K +135,136 (see also Chapter 6 ).
Electroneutral Na + -Cl − transport in the CCD and ENaC-independent K + secretion may play important roles in disconnecting Na + and K + transport within the distal nephron. Electroneutral, thiazide-sensitive, and amiloride-resistant Na + -Cl − transport within the CCD is mediated by the combined activity of the Na + -dependent Cl − -HCO 3 − SLC4A8 Cl − -HCO 3 − exchanger and the SLC26A4 Cl − -HCO 3 − exchanger (see also Chapter 6 ). This transport mechanism is apparently responsible for as much as 50% of Na + -Cl − transport in mineralocorticoid-stimulated rat CCD, allowing for ENaC-independent, electroneutral Na + absorption that will not directly affect K + secretion. The converse effect emerges after dietary K + loading, which increases the fraction of ENaC-independent, amiloride-resistant K + excretion to approximately 50%.
Studies have indicated a key role in K + homeostasis for NCC, the thiazide-sensitive Na + -Cl − cotransporter in the DCT. Selective increases in DCT and NCC activity, as seen in FHHt, reduce Na + delivery to principal cells in the downstream CNT and CCD, leading to hyperkalemia. The DCT also clearly functions as a potassium sensor, directly responding to changes in circulating potassium. Reduction in potassium intake and/or hypokalemia thus lead to reduced basolateral [K + ] in the DCT; the subsequent hyperpolarization is dependent on basolateral KIR4.1-containing K + channels. Hyperpolarization leads to chloride exit via basolateral CLC-NKB chloride channels and a reduction in intracellular chloride; the reduction in intracellular chloride activates the WNK cascade, resulting in phosphorylation of NCC and activation of the transporter. Ang II also activates NCC via WNK-dependent activation of the SPAK kinase and phosphorylation of the transporter protein, reducing delivery of Na + to the CNT and limiting K + secretion. In contrast, Ang II inhibits ROMK activity via several mechanisms, including downstream activation of c-src tyrosine kinases (see earlier). Whereas K + restriction induces renin and circulating angiotensin-II (see “ Consequences of Hypokalemia ”), increases in dietary K + are suppressive. A high-K + diet also inactivates NCC due to the associated decrease in Ang II in addition to the increase in the ratio of WNK1-S to WNK1-L isoforms that occurs with increased K + intake. WNK1-S antagonizes the effect of WNK1-L on NCC, leading to inhibition of NCC in conditions with a relative excess of WNK1-S.
Finally, within principal cells, increases in aldosterone induce the SGK1 kinase, which phosphorylates WNK4 and attenuates the effect of WNK4 on ROMK while activating ENaC. However, when dietary K + intake is reduced, c-src tyrosine kinase activity increases under the influence of increased Ang II, causing direction inhibition of ROMK activity via tyrosine phosphorylation of the channel. The increase in c-src tyrosine kinase activity also abrogates the inhibitory effect of SGK1 on WNK4. Again, Ang II appears to mediate part of its inhibitory effect on ROMK through activation of c-src, such that c-src serves as an important component of the switch that regulates K + secretion in response to changes in dietary K + .
To summarize this important physiology, the differential effects of K + intake on Ang II versus aldosterone appear to be critical in resolving the aldosterone paradox; so too are the differential effects of K + intake on NCC-dependent Na + -Cl − transport in the DCT and on secretory K + channels within the downstream CNT and CCD ( Figure 18.6 ). Under conditions of low Na + intake but moderate K + intake, Ang II and aldosterone are both strongly induced, leading to enhanced Na + -Cl − transport via NCC, increased ENaC activity, and decreased secretory K + channel activity. Although ENaC is activated, the relative inhibition of ROMK by the increased Ang II prevents excessive kaliuresis. Ang II–dependent activation of c-src kinases has direct inhibitory effects on ROMK trafficking and also abrogates the inhibitory effect of SGK1 on WNK4, leading to unopposed inhibition of ROMK by WNK4. In addition, the aldosterone-dependent induction of electroneutral Na + -Cl − transport within the CCD increases Na + -Cl − reabsorption but blunts the effect on the lumen-negative potential, thus limiting kaliuresis. When dietary K + increases, circulating aldosterone is moderately induced, but Ang II is suppressed. This leads to inhibition of NCC and increased downstream delivery of Na + to principal cells in the CNT and CCD, where ENaC activity is increased and ROMK and BK channels are significantly activated. ENaC-independent K + secretion is also strongly induced by increased dietary K + intake, contributing significantly to the ability to excrete K + in the urine.

Regulation of Renal Renin and Adrenal Aldosterone
Modulation of the renin angiotensin aldosterone (RAAS) axis has profound clinical effects on K + homeostasis. Although multiple tissues are capable of renin secretion, renin of renal origin has a dominant physiologic impact. Renin secretion by juxtaglomerular cells within the afferent arteriole is initiated in response to a signal from the macula densa, specifically a decrease in luminal chloride transported through the Na + -K + -2Cl − cotransporter (NKCC2) at the apical membrane of macula densa cells. In addition to this macula densa signal, decreased renal perfusion pressure and renal sympathetic tone stimulate renal renin secretion. The various inhibitors of renin release include Ang II, endothelin, adenosine, atrial natriuretic peptide (ANP), tumor necrosis factor-α (TNF-α), and active vitamin D. The cyclic guanosine monophosphate (cGMP)–dependent protein kinase type II (cGKII) tonically inhibits renin secretion in that renin secretion in response to several stimuli is exaggerated in homozygous cGKII knockout mice. Activation of cGKII by ANP and/or nitric oxide has a marked inhibitory effect on the release of renin from juxtaglomerular cells. Local factors that stimulate renin release from juxtaglomerular cells include prostaglandins, adrenomedullin, catecholamines (β 1 -receptors), and succinate (GPR91 receptor).
The relationship between renal renin release, the RAAS, and cyclo-oxygenase-2 (COX-2) is particularly complex. COX-2 is heavily expressed in the macula densa, with a significant recruitment of COX-2 (+) cells seen with salt restriction or furosemide treatment. Reduced intracellular chloride in macula densa cells appears to stimulate COX-2 expression via p38 MAP kinase, whereas both aldosterone and Ang II reduce its expression. Prostaglandins derived from COX-2 in the macula densa play a dominant role in the stimulation of renal renin release by salt restriction, furosemide, renal artery occlusion, or angiotensin-converting enzyme (ACE) inhibition. Specifically, COX-2–derived prostaglandins appear to play a role in tonic expression of renin in juxtaglomerular (JG) cells via modulation of intracellular cAMP and calcium, rather than functioning in the acute regulation of renin release. Prostaglandins generated by the macula densa also participate in the recruitment of CD44 + mesenchymal stromal cells during salt restriction, which differentiate into renin-producing cells.
Renin released from the kidney ultimately stimulates aldosterone release from the adrenal via Ang II. Hyperkalemia per se is also an independent and synergistic stimulus ( Figure 18.7 ) for aldosterone release from the adrenal gland, although dietary K + loading is less potent than dietary Na + -Cl − restriction in increasing circulating aldosterone. The resting membrane potential of adrenal glomerulosa cells is hyperpolarized due to the activity of the leak K + channels TASK-1 and TASK-3; combined deletion of genes encoding these channels leads to baseline depolarization of adrenal glomerulosa cells and an increase in serum aldosterone that is resistant to dietary sodium loading. Ang II and K + both activate Ca 2+ entry in glomerulosa cells via voltage-sensitive, T-type Ca 2+ channels, primarily Cav3.2. Elevations in extracellular K + thus depolarize glomerulosa cells and activate these Ca 2+ channels, which are independently and synergistically activated by Ang II. Calcium-dependent activation of calcium-calmodulin (CaM)–dependent protein kinase in turn activates the synthesis and release of aldosterone via induction of aldosterone synthase. K + and Ang II also enhance transcription of the Cav3.2 Ca 2+ channel by abrogating repression of this gene by the neuron-restrictive silencing factor (NRS); this ultimately amplifies the induction of aldosterone synthase.

The role of adrenal K + sensing in aldosterone release has been dramatically underlined by the reports of germline and somatic mutations in aldosterone-producing adenomas of transport proteins that control membrane excitability of adrenal zona glomerulosa cells (see also “ Hyperaldosteronism ”). For example, somatic mutations in the adrenal K + channel KCNJ5 (GIRK4) can be detected in about 40% of aldosterone-producing adrenal adenomas ; these mutations endow the channel with a novel Na + conductance, leading to adrenal glomerulosa cell depolarization, Ca 2+ influx, and aldosterone release.
The adrenal release of aldosterone due to increased K + is dependent on an intact adrenal renin angiotensin system, particularly during Na + restriction. ACE inhibitors and angiotensin-receptor blockers (ARBs) thus completely abrogate the effect of high K + on salt-restricted adrenals. Direct, G protein–dependent activation of the TASK-1 and/or TASK-3 K + channels by Ang II receptor type 1A (AT 1A R) or Ang II receptor type 1B (AT 1B R) is thought to underlie the effect of Ang II on adrenal aldosterone release, with abrogation of this effect by ARBs or ACE inhibitors. Other clinically relevant activators of adrenal aldosterone release include prostaglandins and catecholamines via increases in cAMP. Finally, ANP exerts a potent negative effect on aldosterone release induced by K + and other stimuli, at least in part by inhibiting early events in aldosterone synthesis. ANP is therefore capable of inhibiting renal renin release and adrenal aldosterone release, functions that may be central to the pathophysiology of hyporeninemic hypoaldosteronism.
Urinary Indices of Potassium Excretion
A bedside test to measure distal tubular K + secretion directly in humans would be ideal; however, for obvious reasons, this not technically feasible. A widely used surrogate is the transtubular K + gradient (TTKG), which is defined as follows:
TTKG = ( [ K + ] urine × osmality blood ) / ( [ K + ] blood × osmality urine )
The expected values of the TTKG are largely based on historical data, and are less than 3 to 4 in the presence of hypokalemia and more than 6 to 7 in the presence of hyperkalemia; the shifting opinions regarding the physiologically appropriate TTKG in hyperkalemia have been reviewed multiple times. Clearly, water absorption in the CCD and medullary collecting duct is an important determinant of the absolute K + concentration in the final urine—hence, the use of a ratio of urine/plasma osmolality. Indeed, water absorption may in large part determine the TTKG, such that it far exceeds the limiting K + gradient. The TTKG may be less useful in patients ingesting diets of changing K + and mineralocorticoid intake. There is, however, a linear relationship between plasma aldosterone and the TTKG, suggesting that it provides a rough approximation of the ability to respond to aldosterone with a kaliuresis. The response of the TTKG to mineralocorticoid administration, typically fludrocortisone, can thus be used in the diagnostic approach to hyperkalemia (see also Figures 18-11 and 18-15 ). In hypokalemic patients, a TTKG of less than 2 to 3 separates patients with redistributive hypokalemia from those with hypokalemia due to renal potassium wasting, who will have TTKG values that are more than 4.
An alternative to the TTKG in hypokalemic patients is measurement of the urine K + /creatinine ratio. The urine K + /creatinine ratio is usually less than 13 mEq/g creatinine (1.5 mEq/mmol creatinine) when hypokalemia is caused by poor dietary intake, transcellular potassium shifts, gastrointestinal losses, or previous use of diuretics. Higher values are indicative of ongoing renal potassium wasting. The utility of the K + /creatinine ratio was evaluated in a study of 43 patients with severe hypokalemia (range, 1.5 to 2.6 mmol/L) associated with paralysis. The urine K + /creatinine ratio reliably distinguished between the 30 patients with hypokalemic periodic paralysis and the 13 patients with hypokalemia due mostly to renal potassium wasting. The K + /creatinine ratio was thus significantly lower in the patients with periodic paralysis (11 versus 36 mEq/g creatinine; 1.3 versus 4.1 mEq/mmol creatinine). The cutoff value was approximately 22 mEq/g creatinine (2.5 mEq/mmol).
The determination of urinary electrolytes for calculation of the TTKG or urine K + /creatinine ratio provides the opportunity for the measurement of urinary Na + , which will determine whether significant prerenal stimuli are limiting distal Na + delivery and thus K + excretion (see also Figure 18.4 ). Urinary electrolytes also afford the opportunity to calculate the urinary anion gap, an indirect index of urinary NH 4 + content. and thus the ability to respond to an acidemia.
Consequences of Hypokalemia and Hyperkalemia
Consequences of Hypokalemia
Excitable Tissues: Muscle and Heart
Hypokalemia is a well-described risk factor for ventricular and atrial arrhythmias. For example, in patients undergoing cardiac surgery, a serum K + of less than 3.5 mmol/L is a predictor of serious intraoperative arrhythmia, perioperative arrhythmia, and postoperative atrial fibrillation. Moderate hypokalemia does not, however, appear to increase the risk of serious arrhythmia during exercise stress testing. Electrocardiographic changes in hypokalemia include broad flat T waves, ST depression, and QT prolongation; these are most marked when serum K + is less than 2.7 mmol/L. Hypokalemia, often accompanied by hypomagnesemia, is an important cause of the long QT syndrome (LQTS) and torsades de pointes, either alone or in combination with drug toxicity, or with LQTS-associated mutations in cardiac K + and Na + channels. Hypokalemia accelerates the clathrin-dependent internalization and degradation of the cardiac hERG (human ether-à-go-go–related gene) K + channel protein. hERG encodes pore-forming subunits of the cardiac rapidly activating delayed rectifier K + channel (I Kr ); I Kr is largely responsible for potassium efflux during phases 2 and 3 of the cardiac action potential. Loss-of-function mutations in hERG reduce I Kr and cause type II LQTS ; downregulation of hERG and I Kr by hypokalemia provides an elegant explanation for the association with LQTS and torsades de pointes.
In accordance with the Nernst equation, the resting membrane potential is related to the ratio of the intracellular to extracellular potassium concentration. In skeletal muscle, a reduction in plasma K + will increase this ratio and therefore hyperpolarize the cell membrane (i.e., make the resting potential more electronegative); this impairs the ability of the muscle to depolarize and contract, leading to weakness. However, in some human cardiac cells, particularly Purkinje fibers in the conducting system, hypokalemia results in a paradoxic depolarization ; this paradoxic depolarization plays an important role in the genesis of hypokalemic cardiac arrhythmias. Resting membrane potential in excitable cells is largely determined by a large family of K2P1 K + channels, so-named due to the presence of two pore-forming (P) loop domains in each subunit. Hypokalemia causes K2P1 channels, which are normally selective for potassium, to transport sodium into cells suddenly, causing the paradoxic depolarization. Notably, rodent cardiac cells respond to hypokalemia with a Nernst equation–predicted hyperpolarization and, unlike human cardiomyocytes, do not express the K2P1 channel TWIK-1; genetic manipulation indicates that TWIK-1 expression confers this paradoxic depolarization behavior on human and mouse cardiomyocytes.
In skeletal muscle, hypokalemia causes hyperpolarization, thus impairing the capacity to depolarize and contract. Weakness and paralysis is therefore a not infrequent consequence of hypokalemia of diverse causes. On a historical note, the realization in 1946 that K + replacement reversed the hypokalemic diaphragmatic paralysis induced by treatment of diabetic ketoacidosis (DKA) was a milestone in diabetes care. Pathologically, muscle biopsies in hypokalemic myopathy demonstrate phagocytosis of degenerating muscle fibers, fiber regeneration, and atrophy of type 2 fibers. Most patients with significant myopathy will have elevations in creatine kinase, and hypokalemia of diverse causes predisposes to rhabdomyolysis, with acute renal failure.
Renal Consequences
Hypokalemia causes a host of structural and functional changes in the kidney, which are reviewed in detail elsewhere. In humans, the renal pathology includes a relatively specific proximal tubular vacuolization, interstitial nephritis, and renal cysts. Hypokalemic nephropathy can cause ESKD, mostly in patients with long-standing hypokalemia due to eating disorders and/or laxative abuse ; acute renal failure with proximal tubular vasculopathy has also been described. In animal models, hypokalemia increases susceptibility to acute renal failure induced by ischemia, gentamicin, and amphotericin. Potassium restriction in rats induces cortical AT-II and medullary endothelin-1 expression, with an ischemic pattern of renal injury.
The prominent functional changes in renal physiology that are induced by hypokalemia include Na + -Cl − retention, polyuria, phosphaturia, hypocitraturia, and increased ammoniagenesis. K + depletion in rats causes proximal tubular hyperabsorption of Na + -Cl − in association with an upregulation of Ang II, AT 1 receptor, and α 2 -adrenergic receptor in this nephron segment. NHE3, the dominant apical Na + entry site in the proximal tubule, is massively (>700%) upregulated in K + -deficient rats, which is consistent with the observed hyperabsorption of Na + -Cl − and bicarbonate. Polyuria in hypokalemia is due to polydipsia and to a vasopressin-resistant defect in urine-concentrating ability. This renal concentrating defect is multifactorial, with evidence for a reduced hydroosmotic response to vasopressin in the collecting duct and for decreased Na + -Cl − absorption by the TAL. K + restriction has been shown to result in a rapid, reversible decrease in the expression of aquaporin-2 in the collecting duct, beginning in the CCD and extending to the medullary collecting duct within the first 24 hours. In the TAL, the marked reductions seen during K + restriction in the apical K + channel ROMK and apical Na + -K + -2Cl − cotransporter NKCC2 reduce Na + -Cl − absorption, thus inhibiting countercurrent multiplication and the driving force for water absorption by the collecting duct.
Cardiovascular Consequences
A large body of experimental and epidemiologic evidence has implicated hypokalemia and/or reduced dietary K + in the genesis or worsening of hypertension, heart failure, and stroke. K + depletion in young rats induces hypertension, with a salt sensitivity that persists after K + levels are normalized; presumably this salt sensitivity is due to the significant tubulointerstitial injury induced by K + restriction. Short-term K + restriction in healthy humans and patients with essential hypertension also induces Na + -Cl − retention and hypertension, and abundant epidemiologic data have linked dietary K + deficiency and/or hypokalemia with hypertension. Correction of hypokalemia is particularly important in hypertensive patients treated with diuretics; blood pressure in this setting is improved with the establishment of normokalemia, and the cardiovascular benefits of diuretic agents are blunted by hypokalemia. Hypokalemia reduces insulin secretion; this mechanism may play an important role in thiazide-associated diabetes. Finally, K + depletion may play important roles in the pathophysiology and progression of heart failure.
Consequences of Hyperkalemia
Excitable Tissues: Muscle and Heart
Hyperkalemia constitutes a medical emergency, primarily due to its effect on the heart. Hyperkalemia depolarizes cardiac myocytes, reducing the membrane potential from −90 mV to approximately −80 mV. This brings the membrane potential closer to the threshold for generation of an action potential; mild and/or rapid-onset hyperkalemia will initially increase cardiac excitability, since a lesser depolarizing stimulus is required to generate an action potential. Mild increases in extracellular K + also affect the repolarization phase of the cardiac action potential, via increases in I Kr ; as discussed earlier (see “ Consequences of Hypokalemia ”), I Kr is highly sensitive to changes in extracellular K + . This effect on repolarization is thought to underlie the early signs of hyperkalemia, including ST-T segment depression, peaked T waves, and Q-T interval shortening. Persistent and increasing depolarization inactivates cardiac sodium channels, thus reducing the rate of phase 0 of the action potential (V max ); the decrease in V max results in a reduction in myocardial conduction, with progressive prolongation of the P wave, PR interval, and QRS complex. Severe hyperkalemia results in loss of the P wave and a progressive widening of the QRS complex; fusion with T waves causes a sine wave sinoventricular rhythm.
Cardiac arrhythmias associated with hyperkalemia include sinus bradycardia, sinus arrest, slow idioventricular rhythms, ventricular tachycardia, ventricular fibrillation, and asystole. The differential diagnosis and treatment of a wide-complex tachycardia in hyperkalemia can be particularly problematic; moreover, hyperkalemia potentiates the blocking effect of lidocaine on the cardiac Na + channel, such that use of this agent may precipitate asystole or ventricular fibrillation in this setting. Hyperkalemia can also cause a type I Brugada pattern in the electrocardiogram, with a pseudo–right bundle branch block (RBBB) and persistent coved ST segment elevation in at least two precordial leads. This hyperkalemic Brugada sign occurs in critically ill patients with significant hyperkalemia (serum K + > 7 mmol/L) and can be differentiated from genetic Brugada syndrome by an absence of P waves, marked QRS widening, and an abnormal QRS axis.
Classically, the electrocardiographic manifestations in hyperkalemia progress as shown in Table 18.3 . However, these changes are notoriously insensitive, such that only 55% of patients with serum K + more than 6.8 mmol/L in one case series manifested peaked T waves. There is large interpatient variability in the absolute potassium level, leading to electrocardiographic changes and cardiac toxicity of hyperkalemia. Relevant variables include the rapidity of the onset of hyperkalemia and the presence or absence of concomitant hypocalcemia, acidemia, and/or hyponatremia. Hemodialysis patients and patients with chronic renal failure in particular may not demonstrate electrocardiographic changes. Care should also be taken to adequately distinguish the symmetrically peaked, church steeple T waves induced by hyperkalemia from T wave changes of other causes. The ratio of precordial T wave to R wave amplitude (T/R ratio) may be a more specific sign of hyperkalemia than T wave tenting.
Serum K + Concentration (mmol/L) | Electrocardiographic Abnormality |
---|---|
5.5-6.5 | Tall peaked T waves with narrow base, best seen in precordial leads |
6.5-8.0 |
|
>8.0 |
|
Hyperkalemia can also rarely present with ascending paralysis, termed secondary hyperkalemic paralysis to differentiate it from familial hyperkalemic periodic paralysis (HYPP). This presentation of hyperkalemia can mimic Guillain-Barré syndrome and may include diaphragmatic paralysis and respiratory failure. Hyperkalemia from a diversity of causes can cause paralysis, as reviewed by Evers and colleagues. The mechanism is not entirely clear; however, nerve conduction studies in one case suggested a neurogenic mechanism, rather than a direct effect on muscle excitability.
In contrast to secondary hyperkalemic paralysis, HYPP is a primary myopathy. Patients with HYPP develop myopathic weakness during hyperkalemia induced by increased K + intake or rest after heavy exercise. The hyperkalemic trigger in HYPP serves to differentiate this syndrome from hypokalemic periodic paralysis (HOKP); a further distinguishing feature is the presence of myotonia in HYPP. Depolarization of skeletal muscle by hyperkalemia unmasks an inactivation defect in a tetrodotoxin-sensitive Na + channel in patients with HYPP, and autosomal dominant mutations in the SCN4A gene encoding this channel cause most forms of the disease. Mild muscle depolarization (5 to 10 mV) in HYPP results in a persistent inward Na + current through the mutant channel; the normal, allelic SCN4 channels quickly recover from inactivation and can then be reactivated, resulting in myotonia. When muscle depolarization is more marked (20 to 30 mV), all the Na + channels are inactivated, rendering the muscle inexcitable and causing weakness ( Figure 18.8 ). Related disorders due to mutations within the large SCN4A channel protein include HOKP type II, paramyotonia congenita, and K + -aggravated myopathy. American quarter horses have a high incidence (4.4%) of HYPP due to a mutation in equine SCN4A traced to the sire named Impressive (see Figure 18.8 ). Finally, loss-of-function mutations in the muscle-specific K + channel subunit MinK-related peptide 2 (MiRP2) have also been shown to cause HYPP; MiRP2 and the associated Kv3.4 K + channel play a role in setting the resting membrane potential of skeletal muscle.

Renal Consequences
Hyperkalemia has a significant effect on the ability to excrete an acid urine due to interference with the urinary excretion of ammonium (NH 4 + ). Potassium loading in humans results in modest reduction in urinary NH 4 + excretion and an impaired response to acid loading. In rats, chronic potassium loading leads to hyperkalemia and a metabolic acidosis due to a 40% reduction in urinary NH 4 + excretion. Proximal tubular ammonia generation falls, but without a significant effect on proximal tubular secretion of NH 4 + . The TAL absorbs NH 4 + from the tubular lumen, followed by countercurrent multiplication and ultimately excretion from the medullary interstitium ; hyperkalemia appears to inhibit renal acid excretion by competing with NH 4 + for reabsorption by the TAL.
The NH 4 + ion has the same ionic radius as K + and can be transported in lieu of K + by NKCC2, the apical Na + -K + -NH 4 + -2Cl − cotransporter of the TAL; NH 4 + exits the TAL via the basolateral Na + -H + exchanger NHE4. As is the case for other cations, countercurrent multiplication of NH 4 + by the TAL greatly increases the concentration of NH 4 + -NH 3 available for secretion in the collecting duct. The NH 4 + produced by the proximal tubule in response to acidosis is thus reabsorbed across the TAL, concentrated by countercurrent multiplication in the medullary interstitium, and secreted in the collecting duct. The capacity of the TAL to reabsorb NH 4 + is increased during acidosis due to an induction of NKCC2 and NHE4 expression. Hyperkalemia induces acidosis in rats by reducing the NH 4 + between the vasa recta (surrogate for interstitial fluid) and collecting duct due to interference with absorption of NH 4 + by the TAL.
Clinically, patients with hyperkalemic acidosis due to hyporeninemic hypoaldosteronism demonstrate an increase in urinary NH 4 + excretion in response to normalization of plasma K + with cation exchange resins, indicating a significant role for hyperkalemia in generation of the acidosis.
Hypokalemia
Epidemiology
Hypokalemia is a relatively common finding in outpatients and inpatients, perhaps the most common electrolyte abnormality encountered in clinical practice. When defined as a serum K + less than 3.6 mmol/L, it is found in up to 20% of hospitalized patients ; defined as a serum K + less than 3.4 mmol/L, it occurs in 16.8% of first-time hospital admissions. Hypokalemia is usually mild, with K + levels in the 3.0- to 3.5-mmol/L range, but in up to 25% of hypokalemic patients it can be moderate to severe (<3.0 mmol/L). The most common causative factors in hospitalized patients with hypokalemia are gastrointestinal losses of potassium, diuretic therapy, and hypomagnesemia. It is a particularly prominent problem in patients receiving thiazide diuretics for hypertension, with an incidence of up to 48% (average, 15% to 30%). The thiazide-type diuretic metolazone is frequently used in the management of heart failure refractory to loop diuretics alone, causing moderate (K + ≤ 3.0 mmol/L) or severe (K + ≤ 2.5 mmol/L) hypokalemia in approximately 40% and 10% of patients, respectively. Hypokalemia is also a common finding in patients receiving peritoneal dialysis, with 10% to 20% requiring potassium supplementation. Hypokalemia per se can increase in-hospital mortality rate up to 10-fold, likely due to the profound effects on arrhythmogenesis, blood pressure, and cardiovascular morbidity.
Spurious Hypokalemia
Delayed sample analysis is a well-recognized cause of spurious hypokalemia due to increased cellular uptake; this may become clinically relevant if the ambient temperature is increased. Very rarely, patients with profound leukocytosis due to acute leukemia present with artifactual hypokalemia caused by time-dependent uptake of K + by the large white cell mass. Such patients do not develop clinical or electrocardiographic complications of hypokalemia, and plasma K + is normal if measured immediately after venipuncture.
Redistribution and Hypokalemia
Manipulation of the factors affecting internal distribution of K + (see “ Factors Affecting Internal Distribution of Potassium ”) can cause hypokalemia due to redistribution of K + between the extracellular and intracellular compartments. Endogenous insulin is rarely a cause of hypokalemia, but administered insulin is a frequent cause of iatrogenic hypokalemia and may be a factor in what has been termed the dead in bed syndrome associated with aggressive glycemic control. Insulin also may play a significant role in the hypokalemia associated with refeeding syndrome. Alterations in the activity of the endogenous sympathetic nervous system can cause hypokalemia in several settings, including alcohol withdrawal, acute myocardial infarction, and head injury. Redistributive hypokalemia after severe head injury can be truly profound, with reported serum K + of 1.2 mmol/L and 1.9 mmol/L and marked rebound hyperkalemia after repletion.
Due to their ability to activate Na + -K + -ATPase and the Na + -K + -2Cl − cotransporter NKCC1, β 2 -agonists are powerful activators of cellular K + uptake. These agents are chiefly encountered in the therapy of asthma, but tocolytics such as ritodrine can induce hypokalemia and arrhythmias during maternal labor. The long-acting β 2 -agonist clenbuterol, not approved for medical use in the United States, has caused hypokalemia in poisonings, including an outbreak of toxicity from clenbuterol-adulterated heroin in the East Coast of the United States. Occult sources of sympathomimetics, such as pseudoephedrine and ephedrine in cough syrup or dieting agents, can be an overlooked cause of hypokalemia. Finally, downstream activation of cAMP by xanthines such as theophylline and dietary caffeine may induce hypokalemia and may synergize in this respect with β 2 -agonists.
Whereas β 2 -agonists activate K + uptake via Na + -K + -ATPase, one would expect that inhibition of passive K + efflux would also lead to hypokalemia; this is accomplished by barium, a potent inhibitor of inward-rectifying K + channels. This rare cause of hypokalemia is usually due to ingestion of the rodenticide barium carbonate, either unintentionally or during a suicide attempt. Suicidal ingestion of barium-containing shaving powder and hair remover has also been described. Barium salts are widely used in industry, and poisoning has been described by various mechanisms in industrial accidents. Patients have a particularly prominent U wave, likely due to direct inhibition of cardiac inward-rectifying K + channels. Muscle paralysis can also occur due to inhibition of muscle Kir channels. Treatment of barium poisoning with K + serves to increase plasma K + and displace barium from affected K + channels ; hemodialysis is also an effective treatment. Hypokalemia is also common with chloroquine toxicity or overdose, although the mechanism is not entirely clear.
Hypokalemic Periodic Paralysis
The periodic paralyses have genetic and acquired causes and are further subdivided into hyperkalemic and hypokalemic forms. The genetic and secondary forms of hyperkalemic paralysis are discussed earlier (see “ Consequences of Hyperkalemia ”). Autosomal dominant mutations in the CACNA1S gene encoding the α 1 -subunit of L-type calcium channels are the most common genetic cause of hypokalemic periodic paralysis (HOKP type I), whereas type II HOKP is due to mutations in the SCN4A gene encoding the skeletal Na + channel. In Andersen’s syndrome, autosomal dominant mutations in the KCNJ2 gene encoding the inwardly rectifying K + channel Kir2.1 cause periodic paralysis, cardiac arrhythmias, and dysmorphic features. Paralysis in Andersen’s syndrome can be normokalemic, hypokalemic, or hyperkalemic; however, the symptomatic trigger is consistent within individual kindreds.
The pathophysiology of HOKP is complex. Structurally, about 90% of the HOKP-associated mutations result in loss of positively charged arginine residues in the S4, voltage sensor domains of L-type calcium channels and the skeletal Na + channel. This generates a so-called gating current generated by a cation leak through an aberrant pore; this abnormal cation leak may directly lead to K + -dependent paradoxic depolarization and hypokalemic weakness. Muscles of a Na + channel knock-in mutant mouse (Na v 1.4-R669H) also exhibit an anomalous inward current at hyperpolarized potentials, attributed to this gating pore current.
Abnormalities in insulin-sensitive transport events may also contribute to the hypokalemic weakness in HOKP. Reversible attacks of paralysis with hypokalemia in HOKP are typically precipitated by rest after exercise and/or meals rich in carbohydrate. Although the induction of endogenous insulin by carbohydrate meals is thought to reduce plasma K + , thus triggering weakness, insulin can precipitate paralysis in HOKP in the absence of significant hypokalemia. The generation of action potentials and muscle contraction are reduced in types I and II HOKP muscle fibers exposed to insulin in vitro ; this effect is seen at an extracellular K + of 4.0 mmol/L and is potentiated as K + decreases. Type I HOKP muscles have a reduced activity of ATP-sensitive, inward-rectifying K + channels (K ATP ), which likely contributes to hypokalemia due to the resultant unopposed activity of muscle Na + -K + -ATPase. Insulin inhibits the remaining K ATP activity in muscle fibers of type I HOKP patients and hypokalemic rats, resulting in a depolarizing shift toward the equilibrium potential for the Cl − ion (≈50 mV); at this potential, voltage-dependent Na + channels are largely inactivated, resulting in paralysis.
Paralysis is associated with multiple other causes of hypokalemia, acquired and genetic. Renal causes of hypokalemia with paralysis include Fanconi’s syndrome, Gitelman’s syndrome, and the various causes of hypokalemic distal renal tubular acidosis. The activity and regulation of skeletal muscle K ATP channels are aberrant in animal models of hypokalemia, suggesting a parallel muscle physiology to that of genetic HOKP (see earlier). However, the pathophysiology of thyrotoxic periodic paralysis (TPP), a particularly important cause of hypokalemic paralysis, is distinctly different from that of HOKP; for example, despite the clinical similarities between the two syndromes, thyroxine has no effect on HOKP.
TPP is classically seen in patients of Asian origin, but also occurs at higher frequencies in Hispanic patients ; this shared predisposition has been linked to genetic variation in Kir2.6, a muscle-specific, thyroid hormone–responsive K + channel. Patients typically present with weakness of the extremities and limb girdles, with attacks occurring most frequently between 1 and 6 am . As in HOKP, paralytic attacks in TPP may be precipitated by rest and/or by carbohydrate-rich meals. Clinical signs and symptoms of hyperthyroidism are not invariably present. Hypokalemia is profound, with K + ranging between 1.1 and 3.4 mol/L, and is frequently accompanied by hypophosphatemia and hypomagnesemia ; all three abnormalities presumably contribute to the associated weakness. Diagnostically, a TTKG of less than 2 to 3 separates patients with TPP from those with hypokalemia due to renal potassium wasting, who will have TTKG values that are more than 4. This distinction is of considerable therapeutic relevance; patients with large potassium deficits require aggressive repletion with K + -Cl − , which has a significant risk of rebound hyperkalemia in TPP and related disorders.
The hypokalemia in TPP is most likely due to direct and indirect activation of Na + -K + -ATPase, given the evidence for increased activity in erythrocytes and platelets in TPP patients. Thyroid hormone clearly induces expression of multiple subunits of Na + -K + -ATPase in skeletal muscle. Increases in β-adrenergic responses due to hyperthyroidism also play an important role, since high-dose propranolol (3 mg/kg) rapidly reverses the hypokalemia, hypophosphatemia, and paralysis seen in acute attacks. Of particular importance, no rebound hyperkalemia is associated with this treatment, whereas aggressive K + replacement in TPP is associated with an incidence of about 25% ; repletion-associated rebound hyperkalemia in TPP can be fatal.
Outward-directed, inward-rectifying K + current, mediated by Kir channels (particularly Kir2.6 and Kir2.1), is also reduced in skeletal muscles of patients with TPP, providing an additional mechanism for hypokalemia. In patients with Kir2.6 mutations, this reduction is at least in part hereditary. Together with increased Na + -K + -ATPase activity and increased circulating insulin, this reduced Kir current may trigger a feed-forward cycle of hypokalemia, leading to inactivation of muscle Na + channels, paradoxic depolarization, and paralysis.
Potassium Loss
Nonrenal Potassium Loss
The loss of K + from skin is typically low, with the exception of extremes in physical exertion. Direct gastric loss of K + due to vomiting or nasogastric suctioning is also typically minimal; however, the ensuing hypochloremic alkalosis results in persistent kaliuresis due to secondary hyperaldosteronism and bicarbonaturia. Intestinal loss of K + due to diarrhea is a quantitatively important cause of hypokalemia, given the worldwide prevalence of diarrheal disease, and may be associated with acute complications, such as myopathy and flaccid paralysis. The presence of a non–anion gap metabolic acidosis with a negative urinary anion gap (consistent with an intact ability to increase NH 4 + excretion) should strongly suggest diarrhea as a cause of hypokalemia. Polyethylene glycol–based bowel preparation regimens for colonoscopy can also lead to hypokalemia in older patients. Noninfectious gastrointestinal processes such as celiac disease, ileostomy, and chronic laxative abuse can present with acute hypokalemic syndromes or with chronic complications, such as ESKD.
Three reports initially identified a novel association between colonic pseudo-obstruction (Ogilvie’s syndrome) and hypokalemia due to secretory diarrhea with an abnormally high K + content. In one patient with concomitant ESKD, immunohistochemistry revealed massive upregulation of the apical BK channel throughout the surface crypt axes ; colonic BK channels may play a significant role in intestinal K + secretion in a variety of pathologies, including ESKD. Several hypotheses for the association between Ogilvie’s syndrome and enhanced intestinal K + secretion have been postulated, including active stimulation by catecholamines induced by colonic pseudo-obstruction ; BK channels appear to mediate adrenaline-induced colonic K + secretion.
Increased fecal loss of K + may play a broader role in hypokalemia associated with diarrhea. Recruitment of colonic BK channels along intestinal crypts, similar to that seen in Ogilvie’s syndrome, has thus been demonstrated as a consistent feature of colonic biopsies in ulcerative colitis. Direct enhancement of intestinal K + excretion has also been demonstrated in a hypokalemic patient with Crohn’s disease following treatment with budesonide.
Renal Potassium Loss
Drugs
Diuretics are an especially important cause of hypokalemia due to their ability to increase distal flow rate and distal delivery of Na + . For a given degree of natriuresis, thiazides generally cause more profound degrees of hypokalemia than loop diuretics, despite their lower natriuretic efficacy. One potential explanation is the differential effect of loop diuretics and thiazides on calcium excretion. Whereas thiazides and loss-of-function mutations in the Na + -Cl − cotransporter decrease Ca 2+ excretion, loop diuretics cause a significant calciuresis. Increases in luminal Ca 2+ in the distal nephron serve to reduce the lumen-negative driving force for K + excretion, perhaps by direct inhibition of ENaC in principal cells. A mechanistic explanation is provided by the presence of an apical calcium-sensing receptor (CaSR) in the collecting duct ; analogous to the evident decrease in the apical trafficking of aquaporin-2 induced by luminal Ca 2+ , tubular Ca 2+ may stimulate endocytosis of ENaC via the CaSR and thus limit generation of the lumen-negative potential difference that is so critical for distal K + excretion. Regardless of the underlying mechanism, the increase in distal delivery of Ca 2+ induced by loop diuretics may serve to blunt kaliuresis; such a mechanism would not occur with thiazides, which reduce distal delivery of Ca 2+ , with unopposed activity of ENaC and increased kaliuresis.
Some studies have also indicated a key role in K + homeostasis for NCC, the thiazide-sensitive Na + -Cl − cotransporter in the DCT, so it is not surprising that thiazide treatment has such potent effects on serum K + . Selective increases in DCT and NCC activity, as seen in FHHt, reduce Na + delivery to principal cells in the downstream CNT and CCD, leading to hyperkalemia. The DCT also clearly functions as a potassium sensor, directly responding to changes in circulating potassium. A high-K + diet also inactivates NCC, whereas NCC is activated in hypokalemia.
Other drugs associated with hypokalemia due to kaliuresis include toxic levels of acetaminophen, which causes dose-dependent hypokalemia. High doses of penicillin-related antibiotics are another important cause of hypokalemia, increasing obligatory K + excretion by acting as nonreabsorbable anions in the distal nephron; in addition to penicillin, implicated antibiotics include nafcillin, dicloxacillin, ticarcillin, oxacillin, and carbenecillin. Increased distal delivery of other anions such as SO 4 2− and HCO 3 − also induces a kaliuresis. The usual explanation is that K + excretion increases so as to balance the negative charge of these nonreabsorbable anions. However, increased delivery of such anions will also increase the electrochemical gradient for K + -Cl − exit via apical K + -Cl − cotransport or parallel K + -H + and Cl − -HCO 3 − exchange (see also “ Potassium Transport in the Distal Nephron ”). Drugs are also an important cause of Fanconi’s syndrome, which is often associated with significant hypokalemia (see “ Renal Tubular Acidosis ”).
Several tubular toxins result in K + and magnesium wasting. These include gentamicin, which can cause tubular toxicity with hypokalemia that can masquerade as Bartter’s syndrome. Other drugs that can cause mixed magnesium and K + wasting include amphotericin, foscarnet, cisplatin, and ifosfamide. One intriguing cause of hypomagnesemia and hypokalemia is cetuximab, a humanized monoclonal antibody specific for the receptor for epidermal growth factor (EGF) ; paracrine EGF stimulates magnesium transport via the apical TRPM6 cation channel in the DCT, with magnesium wasting and hypomagnesemia in patients treated with cetuximab. Aggressive replacement of magnesium is obligatory in the treatment of combined hypokalemia and hypomagnesemia, since successful K + replacement depends on treatment of the hypomagnesemia.
Hyperaldosteronism
Increases in circulating aldosterone (hyperaldosteronism) may be primary or secondary. Increased levels of circulating renin in secondary forms of hyperaldosteronism lead to increased levels of Ang II, and thus aldosterone, and can be associated with hypokalemia; causes include renal artery stenosis, Page kidney (renal compression by a subcapsular mass or hematoma, with hyperreninemia), a paraneoplastic process, or renin-secreting renal tumors. The incidence of hypokalemia in renal artery stenosis is thought to be less than 20%. An unusual presentation of renal artery stenosis and renal ischemia is what has been termed the hyponatremic hypertensive syndrome, in which concurrent hypokalemia may be profound.
Primary hyperaldosteronism may be genetic or acquired. Hypertension and hypokalemia, generally attributed to increases in circulating 11-deoxycorticosterone, are seen in patients with congenital adrenal hyperplasia due to defects in steroid 11β-hydroxylase or steroid 17α-hydroxylase ; deficient 11β-hydroxylase results in virilization and other signs of androgen excess, whereas reduced sex steroids in 17α-hydroxylase deficiency result in hypogonadism. The two major forms of isolated PA are denoted familial hyperaldosteronism type I (FH-I, also known as glucocorticoid-remediable hyperaldosteronism [GRA]) and familial hyperaldosteronism type II (FH-II), in which aldosterone production is not repressible by exogenous glucocorticoids. Patients with FH-II are clinically indistinguishable from sporadic forms of PA due to bilateral adrenal hyperplasia; a gene has been localized to chromosome 7p22 by linkage analysis, but has yet to be characterized. A third form of familial hyperaldosteronism (FH-III) was initially described in 2008, with hyporeninemia, hyperaldosteronism resistant to dexamethasone, and very high levels of 18-oxocortisol and 18-hydroxycortisol. FH-III is due to somatic mutations in the adrenal K + channel KCNJ5, which endow the channel with a novel Na + conductance and activate adrenal glomerulosa proliferation and aldosterone release ; somatic mutations in KCNJ5 are also found in spontaneous adrenal adenomas (see later).
Patients with FH-I (GRA) are generally hypertensive, typically presenting at an early age; the severity of hypertension is variable, however, such that some affected individuals are normotensive. Aldosterone levels are modestly elevated and regulated solely by ACTH. The diagnosis can be biochemically confirmed by a dexamethasone suppression test, with a suppression of aldosterone to less than 4 ng/dL consistent with the diagnosis. Patients also have high levels of abnormal hybrid 18-hydroxylated steroids, generated by transformation of steroids typically formed in the zona fasciculata by aldosterone synthase, an enzyme that is normally expressed in the zona glomerulosa. FH-I has been shown to be caused by a chimeric gene duplication between the homologous 11β-hydroxylase gene ( CYP11B1 ) and aldosterone synthase gene ( CYP11B2 ), fusing the ACTH-responsive 11β-hydroxylase promoter to the coding region of aldosterone synthase. This chimeric gene is thus unde the control of ACTH and is expressed in a glucocorticoid-repressible fashion. Ectopic expression of the hybrid CYP11B1-CYP11B1 gene in the zona fasciculata has been reported in a single case in which adrenal tissue became available for molecular analysis. Direct genetic testing for the hybrid CYP11B1-CYP11B2 has largely supplanted biochemical screening for FH-I; genetic testing for FH-I should be pursued in patients with PA and a family history of PA and/or of strokes at a young age, or in younger patients with PA (age < 20 years).
Although the initial patients reported with FH-I were hypokalemic, most are normokalemic, albeit perhaps with a propensity to develop hypokalemia while on thiazide diuretics. Patients with FH-I are able to increase K + excretion appropriately in response to K + loading or fludrocortisone, but fail to increase plasma aldosterone in response to hyperkalemia. This may reflect the ectopic expression of the chimeric aldosterone synthase in the adrenal fasciculata, which likely lacks the appropriate constellation of ion channels to respond to increases in extracellular K + with an increase in aldosterone secretion.
Acquired causes of PA include aldosterone-producing adenomas (APAs; 35% of cases), primary (or unilateral) adrenal hyperplasia (PAH; 2% of cases), idiopathic hyperaldosteronism (IHA) due to bilateral adrenal hyperplasia (60% of cases), and adrenal carcinoma(<1% of cases). A rare case involving paraneoplastic overexpression of aldosterone synthase in lymphoma has also been described.
The molecular characterization of adrenal adenomas with whole-genome sequencing and related techniques has been remarkably fruitful. In particular, acquired mutations in the adrenal K + channel KCNJ5 can be detected in about 40% of aldosterone-producing adrenal adenomas. As in FH-III (see earlier), these somatic mutations endow the channel with a novel Na + conductance, leading to adrenal glomerulosa cell depolarization, Ca 2+ influx, and aldosterone release. Clinically, patients with adrenal KCNJ5 mutations have a higher preoperative aldosterone level and higher lateralization index in adrenal vein sampling, without affecting the surgical response to adrenalectomy. Less frequently, somatic mutations in adrenal adenomas can be detected in the calcium channel CACNAID or in a subunit (ATP2B3) of the Ca 2+ -ATPase pump, predicted also to lead to increased Ca 2+ influx and aldosterone release. Acquired mutations in the ATP1A α 1 -subunit of Na+-K+-ATPase are in turn thought to generate chronic depolarization, leading also to exaggerated aldosterone release.
Increasing use of the plasma aldosterone concentration (PAC)/plasma renin activity (PRA) ratio in hypertension clinics has led to reports of a much higher incidence of PA than previously appreciated, with incidence rates in hypertension ranging from 0% to 72% ; however, the prevalence was 3.2% in a large multicenter study of patients with mild to moderate hypertension without hypokalemia. Regardless, the PAC/PRA ratio is a screening tool, which typically must be confirmed by aldosterone suppression testing, which measures PAC or aldosterone secretion after loading with salt or intravenous saline. After controlling hypertension and hypokalemia, oral salt loading over 3 days is followed by measurement of 24-hour urine aldosterone, sodium, and creatinine excretion. The 24-hour sodium excretion should exceed 200 mmol/day for adequate suppression, and a urinary aldosterone of more than 33 nmol/day (12 µm/day) is consistent with PA. Alternatively, in the saline infusion test, recumbent patients are infused with 2 L of isotonic saline over 4 hours, followed by measurement of PAC. In patients without PA, the measured PAC after saline infusion should decrease to less than 139 pmol/L. The measured PAC in patients with PA usually does not decrease to less than 277 pmol/L; indeterminate values between 139 and 277 pmol/L can been seen in patients with IHA. It should be noted, however, that patients with high-probability factors (hypokalemia, hypertension, high PAC/PRA ratio, abnormalities) may not necessarily require confirmatory testing, proceeding instead directly to adrenal venous sampling.
Since surgery can be curative in APA, adequate differentiation of APA from IHA is critical; this requires adrenal imaging and adrenal venous sampling ( Figure 18.9 ). Contemporary reports and recommendations have thus emphasized the continued importance of adrenal vein sampling in subtype differentiation. Laparoscopic adrenalectomy has increasingly been the preferred surgical management for APA or PAH. Mineralocorticoid receptor antagonists are indicated for medical therapy of PA, with carefully monitored use of glucocorticoid to suppress ACTH in some patients with FH-I.

The true incidence of hypokalemia in patients with acquired forms of PA remains difficult to evaluate due to a variety of factors. First, historically, patients have only been screened for hyperaldosteronism when hypokalemia is present, so even case series from clinics with such a referral pattern may suffer from a selection bias; other series have concentrated on hypertensive patients, also with a selection bias. Second, the incidence of hypokalemia is higher in adrenal adenomas than in IHA, likely due to higher average levels of aldosterone. Third, since increased kaliuresis in hyperaldosteronism can be induced by dietary Na + -Cl − loading or diuretics, dietary factors and/or medications may play a role in the incidence of hypokalemia at presentation. Regardless, it is clear that hypokalemia is not a universal feature of PA; this is perhaps not unexpected, since aldosterone does not appear to affect the hypokalemic response of H + -K + -ATPase, the major reabsorptive pathway for K + in the distal nephron (see also Chapter 6 ). A related issue is whether PA is underdiagnosed when hypokalemia is used as a criterion for further investigation; the utility of the PAC/PRA ratio in screening for hyperaldosteronism is an active issue in hypertension research.
Finally, hypokalemia may also occur with systemic increases in glucocorticoids. In bona fide Cushing’s syndrome caused by increases in pituitary ACTH, the incidence of hypokalemia is only 10%, whereas it is 57% to 100% in patients with ectopic ACTH, despite a similar incidence of hypertension. Ectopic ACTH expression is associated primarily with neuroendocrine malignancies, most commonly bronchial carcinoid tumors, small lung cancer, and other neuroendocrine tumors. Indirect evidence suggests that the activity of renal 11β-hydroxysteroid dehydrogenase-2 (11βHSD-2) is reduced in patients with ectopic ACTH compared with Cushing’s syndrome, resulting in a syndrome of apparent mineralocorticoid excess (see later). Whether this reflects a greater degree of saturation of the enzyme by circulating cortisol or direct inhibition of 11βHSD-2 by ACTH is not entirely clear, and there is evidence for both mechanisms ; however, indirect indices of 11βHSD-2 activity in patients with ectopic ACTH expression correlate with hypokalemia and other measures of mineralocorticoid activity. Similar mechanisms likely underlie the severe hypokalemia reported in patients with familial glucocorticoid resistance, in which loss-of-function mutations in the glucocorticoid receptor result in marked hypercortisolism without cushingoid features, accompanied by very high ACTH levels.
Syndromes of Apparent Mineralocorticoid Excess
The syndromes of apparent mineralocorticoid excess (AME) have a self-explanatory label. In the classic form of AME, recessive loss-of-function mutations in the 11β-hydroxysteroid dehydrogenase-2 gene ( 11βHSD-2 ) cause a defect in the peripheral conversion of cortisol to the inactive glucocorticoid cortisone; the resulting increase in the half-life of cortisol is associated with a marked decrease in synthesis, such that plasma levels of cortisol are normal and patients are not cushingoid. The 11βHSD-2 protein is expressed in epithelial cells that are targets for aldosterone; in the kidney, these include cells of the DCT, CNT, and CCD. Since the mineralocorticoid receptor (MR) has equivalent affinity for aldosterone and cortisol, generation of cortisone by 11βHSD-2 serves to protect mineralocorticoid-responsive cells from illicit activation by cortisol. In patients with AME, the unregulated mineralocorticoid effect of glucocorticoids results in hypertension, hypokalemia, and metabolic alkalosis, with suppressed PRA and aldosterone levels. Biochemical diagnosis entails measuring the urinary free cortisol to urinary free cortisone ratio on a 24-hour urine collection. Biochemical studies of mutant enzymes usually indicate a complete loss of function; lesser enzymatic defects in patients with AME are associated with altered ratios of urinary cortisone-cortisol metabolites, lesser impairment in the peripheral conversion of cortisol to cortisone, and/or older age at presentation.
Mice with a homozygous targeted deletion of 11βHsd-2 exhibit hypertension, hypokalemia, and polyuria; the polyuria is likely secondary to the hypokalemia (see “ Consequences of Hypokalemia: Renal Consequences ”), which reaches 2.4 mmol/mL in 11βHsd-2 null mice. As expected, PRA and plasma aldosterone levels in the 11βHsd-2 -null mice are profoundly suppressed, with a decreased urinary Na + /K + ratio that is increased by dexamethasone (given to suppress endogenous cortisol). These knockout mice have significant nephromegaly, due to a massive hypertrophy and hyperplasia of distal convoluted tubules. The relative effect of genotype on the morphology of cells in the DCT, CNT, and CCD was not determined by appropriate phenotypic studies ; however, it is known that the DCT and CCD are target cells for aldosterone, and both cell types express 11βHSD-2. The induction of ENaC activity by unregulated glucocorticoid likely causes the Na + retention and marked increase in K + excretion in 11βHsd-2 null mice; distal tubular micropuncture studies in rats treated with a systemic inhibitor of 11βHSD-2 are consistent with such a mechanism. In addition, the cellular gain of function in the DCT would be expected to be associated with hypercalciuria, given the phenotype of pseudohypoaldosteronism type II and Gitelman’s syndrome (see “Hereditary Tubular Causes of Hyperkalemia” and later, “ Gitelman’s Syndrome ”); indeed, patients with AME are reported to exhibit nephrocalcinosis.
Pharmacologic inhibition of 11βHSD-2 is also associated with hypokalemia and AME. The most infamous offender is licorice, in its multiple guises (e.g., licorice root, tea, candies, herbal remedies). The early observations that licorice required small amounts of cortisol to exert its kaliuretic effect, in the addisonian absence of endogenous glucocorticoid, presaged the observations that its active ingredients (glycyrrhetinic acid or glycyrrhizinic acid and carbenoxolone) inhibit 11βHSD-2 and related enzymes. Licorice intake remains considerable in European countries, particularly Iceland, the Netherlands, and Scandinavia ; Pontefract cakes, eaten as sweets and as a laxative, are a continued source of licorice in the United Kingdom, whereas it is an ingredient in several popular sweeteners and preservatives in Malaysia. Glycyrrhizinic acid is used in Japan to treat hepatitis and has been under evaluation elsewhere for the management of hepatitis C; AME has been reported with its use for this indication. Glycyrrhizinic acid is also a component of Chinese herbal remedies, prescribed for disorders such as allergic rhinitis. Pharmacologic inhibition of 11βHSD-2 has also been tested in patients with ESKD as a novel mechanism to control hyperkalemia (see “ Treatment of Hyperkalemia ”). Carbenoxolone is, in turn, used in some countries in the management of peptic ulcer disease.
Finally, a mechanistically distinct form of AME has been reported due to a gain-of-function mutation in the MR. A single kindred has been described with autosomal dominant inheritance of severe hypertension and hypokalemia; the causative mutation involves a serine residue that is conserved in the MR from multiple species, yet differs in other nuclear steroid receptors. This mutation results in constitutive activation of the MR in the absence of ligand and induces significant affinity for progesterone. The MR is thus constitutively “on” in these patients, with a marked stimulation by progesterone; of interest, pregnancies in the affected female members of the family were all complicated by severe hypertension due to marked increases in plasma progesterone induced by the gravid state.
Liddle’s Syndrome
Liddle’s syndrome constitutes an autosomal dominant gain in function of ENaC, the amiloride-sensitive Na + channel of the CNT and CCD. Patients manifest severe hypertension with hypokalemia, unresponsive to spironolactone yet sensitive to triamterene and amiloride. Liddle’s syndrome could therefore also be classified as a syndrome of apparent mineralocorticoid excess. Both hypertension and hypokalemia are variable aspects of the Liddle’s phenotype; consistent features include a blunted aldosterone response to ACTH and reduced urinary aldosterone excretion. The differential diagnosis for Liddle’s syndrome, as a cause of hereditary hypertension with hypokalemia and suppressed aldosterone, includes AME due to deficient 11βHSD-2; however, whereas the Liddle’s syndrome phenotype is resistant to blockade of the MR with spironolactone and sensitive to amiloride, AME patients are sensitive to both drugs. Commercial genetic testing for both syndromes is available in the United States.
The vast majority of mutations target the C terminus of the β- or γ-ENaC subunit. ENaC channels containing Liddle’s syndrome mutations are constitutively overexpressed at the cell membrane ; unlike wild-type ENaC channels, they are not sensitive to inhibition by intracellular Na + , an important regulator of endogenous channel activity in the CCD. The mechanism whereby mutations in the C terminus of ENaC subunits lead to this channel phenotype have been discussed earlier in this chapter (see Figure 18.5 and “ Control of Potassium Secretion: Aldosterone ”). In addition to effects on interaction with Nedd4-2–dependent retrieval from the plasma membrane, Liddle’s syndrome–associated mutations increase proteolytic cleavage of ENaC at the cell membrane ; aldosterone-induced channel-activating proteases activate ENaC channels at the plasma membrane. This important result provides a mechanistic explanation for the long-standing observation that Liddle’s syndrome–associated mutations in ENaC appear to have a dual activating effect on both the open probability of the channel (i.e., on channel activity) and on expression at the cell membrane.
Given the overlapping and synergistic mechanisms that regulate ENaC activity, it stands to reason that mutations in ENaC that give rise to Liddle’s syndrome might do so by a variety of means. Indeed, mutation of a residue within the extracellular domain of ENaC increases open probability of the channel without changing surface expression; the patient with this mutation has a typical Liddle’s syndrome phenotype. Extensive searches for more common mutations and polymorphisms in ENaC subunits that correlate with blood pressure in the general population have essentially been negative. However, there are a handful of genetic studies that correlate specific variants in ENaC subunits with biochemical evidence of greater in vivo activity of the channel—that is, a suppressed PRA and aldosterone and/or increased ratios of the urinary K + level to aldosterone or to PRA.
Familial Hypokalemic Alkalosis
Bartter’s Syndrome.
Bartter’s and Gitelman’s syndromes are the two major variants of familial hypokalemic alkalosis; Gitelman’s syndrome is a much more common cause of hypokalemia than Bartter’s syndrome. Whereas a clinical subdivision of these syndromes has been used in the past, a genetic classification is increasingly in use, due in part to phenotypic overlap. Patients with classic Bartter’s syndrome (BS) typically suffer from polyuria and polydipsia and manifest a hypokalemic, hypochloremic alkalosis. They may have an increase in urinary calcium (Ca 2+ ) excretion, and 20% are hypomagnesemic. Other features include marked elevation of plasma Ang II, plasma aldosterone, and plasma renin levels. Patients with antenatal BS present earlier in life with a severe systemic disorder characterized by marked electrolyte wasting, polyhydramnios, and significant hypercalciuria with nephrocalcinosis. Prostaglandin synthesis and excretion is significantly increased and may account for many of the systemic symptoms. Decreasing prostaglandin synthesis by COX inhibition can improve polyuria in patients with BS by reducing the amplifying inhibition of urine-concentrating mechanisms by prostaglandins. Indomethacin also increases plasma K + and decreases plasma renin activity, but does not correct the basic tubular defect; it does, however, appear to help increase the growth of BS patients. Of interest, COX-2 immunoreactivity is increased in the TAL and macula densa of patients with BS, and studies have indicated a clinical benefit of COX-2 inhibitors.
Early studies in BS have suggested that these patients have a defect in the function of the TAL. Many of the clinical features are mimicked by the administration of loop diuretics, to which at least a subset of patients with antenatal BS do not respond. The apical Na + -K + -2Cl − cotransporter (NKCC2, SLC12A1) of the mammalian TAL ( Figure 18.10 ) was thus an early candidate gene. In 1996, disease-associated mutations were found in the human NKCC2 gene in four kindreds with antenatal BS ; in the genetic classification of BS, these patients are considered to have BS type I. Although the functional consequences of disease-associated NKCC2 mutations have not been comprehensively studied, the first and subsequent reports included patients with frameshift mutations and premature stop codons that predicted the absence of a functional NKCC2 protein.

BS is a genetically heterogeneous disease. Given the role of apical K + permeability in the TAL, encoded at least in part by ROMK, this K + channel was another early candidate gene. K + recycling via the Na + -K + -2Cl − cotransporter and apical K + channels generates a lumen-positive potential difference in the TAL, which drives the paracellular transport of Na + and other cations (see also Figure 18.10 ). Multiple disease-associated mutations in ROMK have been reported in patients with BS type II, most of whom exhibited the antenatal phenotype. Finally, mutations in BS type III have been reported in the chloride channel CLC-NKB, which is expressed at the basolateral membrane of at least the TAL and DCT. Patients with mutations in CLC-NKB typically have the classic Bartter’s phenotype, with a relative absence of nephrocalcinosis. In a significant fraction of patients with BS, the NKCC2, ROMK, and CLC-NKB genes are not involved. For example, a subset of patients with associated sensorineural deafness exhibit linkage to chromosome 1p31 ; the gene for this syndrome, denoted Barttin, is an obligatory subunit for the CLC-NKB chloride channel. The occurrence of deafness in these patients suggests that Barttin functions in the regulation or function of Cl − channels in the inner ear. Notably, the CLC-NKB gene is immediately adjacent to that for another epithelial Cl − channel, denoted CLC-NKA; digenic inactivation was described in two siblings with deafness and BS, suggesting that CLC-NKA plays an important role in Barttin-dependent Cl − transport in the inner ear.
Patients with activating mutations in the CaSR have been described with autosomal dominant hypocalcemia and hypokalemic alkalosis. The CaSR is heavily expressed at the basolateral membrane of the TAL, where it is thought to play an important inhibitory role in regulating the transcellular transport of both Na + -Cl − and Ca 2+ . For example, activation of the basolateral CaSR in the TAL is known to reduce apical K + channel activity, which would induce a Bartter-like syndrome (see Figure 18.10 ). Coexpression of NKCC2 with a Bartter’s syndrome gain-of-function mutant in the CaSR reveals reduced phosphorylation and reduced activity of NKCC2, dependent on the generation of inhibitory arachidonic acid–derived metabolites known to inhibit TAL function. Genetic activation of the CaSR by these mutations is also expected to increase urinary Ca 2+ excretion by inhibiting generation of the lumen-positive potential difference that drives paracellular Ca 2+ transport in the TAL. In addition, the set point of the CaSR response to Ca 2+ in the parathyroid is shifted to the left, inhibiting parathyroid hormone (PTH) secretion by this gland. It is very likely that the positional cloning of other BS genes will have a considerable impact on the mechanistic understanding of the TAL.
Despite the reasonable correlation between the disease gene involved and the associated subtype of familial alkalosis, there is significant phenotypic overlap and phenotypic variability in hereditary hypokalemic alkalosis. For example, patients with mutations in CLC-NKB usually exhibit classic BS, but can present with a more severe antenatal phenotype, or even with a phenotype similar to Gitelman’s syndrome. With respect to BS due to mutations in NKCC2, a number of patients have been described with variant presentations, including an absence of hypokalemia. Two brothers were described with a late onset of mild BS; these patients were found to be compound heterozygotes for a mutant form of NKCC2 that exhibits partial function, with a loss-of-function mutation on the other NKCC2 allele.
BS type II is particularly relevant to K + homeostasis, given that ROMK is the SK secretory channel of the CNT and CCD (see Chapter 6 , “ K + Secretion by the Distal Convoluted Tubule, Connecting Tubule, and Cortical Collecting Duct ”). Patients with BS type II typically have slightly higher serum K + than the other genetic forms of BS ; patients with severe (9.0 mmol/L), transient, neonatal hyperkalemia have also been described. It is likely that this reflects a transient developmental deficit in the other K + channels involved in distal K + secretion, including the apical maxi-K channel responsible for flow-dependent K + secretion in the distal nephron. Distal K + secretion in ROMK knockout mice is primarily mediated by maxi-K–BK channel activity, such that developmental deficits in this channel would lead to hyperkalemia in BS type II. The mammalian TAL has two major apical K + conductances, the 30-pS channel corresponding to ROMK and a 70-pS channel ; both are thought to play a role in transepithelial salt transport by the TAL. ROMK is evidently a subunit of the 70-pS channel, given the absence of this conductance in TAL segments of ROMK knockout mice. The identity of the other putative subunit of this 70-pS channel is not as yet known; one would assume that deficiencies in this gene would also be a cause of BS.
Finally, BS must be clinically differentiated from the various causes of pseudo–Bartter’s syndrome; these commonly include laxative abuse, furosemide abuse, and bulimia (see “ Clinical Approach to Hypokalemia ”). Other reported causes include gentamicin nephrotoxicity, Sjögren’s syndrome, and cystic fibrosis (CF). Fixed loss of Na + -Cl − in sweat is likely the dominant predisposing factor for hypokalemic alkalosis in patients with CF; patients with this presentation generally respond promptly to intravenous fluids and electrolyte replacement. However, the cystic fibrosis transmembrane conductance regularor (CFTR) protein coassociates with ROMK in the TAL and confers sensitivity to ATP and glibenclamide to apical K + channels in this nephron segment. Lu and associates have proposed that this interaction serves to modulate the response of ROMK to cAMP and vasopressin, such that K + excretion in CFTR deficiency would not be appropriately reduced during water diuresis, therefore predisposing these patients to the development of hypokalemic alkalosis.
Gitelman’s Syndrome.
A major advance in the understanding of hereditary alkaloses was the realization that a subset of patients exhibit marked hypocalciuria, rather than the hypercalciuria typically seen in BS; patients in this hypocalciuric subset are universally hypomagnesemic. Such patients are now clinically classified as suffering from Gitelman’s syndrome (GS). Although plasma renin activity may be increased, renal prostaglandin excretion is not elevated in these hypocalciuric patients, another distinguishing feature between BS and GS. GS is a milder disorder than BS; however, patients do report significant morbidity, mostly related to muscular symptoms and fatigue. The QT interval is frequently prolonged in GS, suggesting an increased risk of cardiac arrhythmia ; however, a more exhaustive cardiac evaluation of a large group of patients failed to detect significant abnormalities of cardiac structure or rhythm. However, presyncope and/or ventricular tachycardia has been observed in at least two patients with GS, one with concomitant long QT syndrome due to a mutation in the cardiac KCNQ1 K + channel.
The hypocalciuria detected in GS was an expected consequence of inactivating the thiazide-sensitive Na + -Cl − cotransporter NCC (SLC12A2), and loss-of-function mutations in the human gene have been reported. Many of these mutations lead to a defect in cellular trafficking when introduced into the human NCC protein. GS is genetically homogeneous, except for the occasional patient with mutations in CLC-NKB and an overlapping phenotype. However, genetic analysis is not generally available, given the significant number of exons in SLC12A2 and the absence of hot spot mutations in this disorder. One diagnostic alternative is to assess the physiologic response to thiazides; patients with GS have a blunted excretion of chloride after the administration of hydrochlorothiazide.
The NCC protein has been localized to the apical membrane of epithelial cells in the DCT and connecting segment. A mouse strain with targeted deletion of the Slc12a2 gene encoding NCC exhibits hypocalciuria and hypomagnesemia, with a mild alkalosis and marked increase in circulating aldosterone. These knockout mice exhibit marked morphologic defects in the early DCT, with a reduction in absolute number of DCT cells and changes in ultrastructural appearance. That GS is a disorder of cellular development and/or cellular apoptosis should perhaps not be a surprise, given the observation that thiazide treatment promotes marked apoptosis of this nephron segment. This cellular deficit leads to downregulation of the DCT magnesium channel TRPM6, resulting in the magnesium wasting and hypomagnesemia seen in GS. The downstream CNT tubules are hypertrophied in NCC-deficient mice, reminiscent of the hypertrophic DCT and CNT segments seen in furosemide-treated animals. These CNT cells also exhibit an increased expression of ENaC at their apical membranes versus littermate controls ; this is likely due to activation of SGK1-dependent trafficking of ENaC by the increase in circulating aldosterone (see “ Control of Potassium Secretion: Aldosterone ”).
Hypokalemia does not occur in NCC −/− mice on a standard rodent diet but emerges on a K + -restricted diet; plasma K + of these mice is about 1 mmol/L lower than K + -restricted littermate controls. Several mechanisms account for the hypokalemia seen in GS and NCC −/− mice. The distal delivery of Na + and fluid is decreased in NCC −/− mice, at least those on a normal diet; however, the increased circulating aldosterone and CNT hypertrophy likely compensate, leading to increased kaliuresis. As discussed earlier for thiazides, decreased luminal Ca 2+ in NCC deficiency may augment baseline ENaC activity, further exacerbating the kaliuresis. Of particular interest, NCC-deficient mice develop considerable polydipsia and polyuria on a K + -restricted diet ; this is reminiscent perhaps of the polydipsia that has been implicated in thiazide-associated hyponatremia.
Hypocalciuria in GS is not accompanied by changes in plasma calcium, phosphate, vitamin D, or PTH levels, suggesting a direct effect on renal calcium transport. The late DCT is morphologically intact in NCC-deficient mice, with preserved expression of the epithelial calcium channel (ECAC1, or TRPV5) and the basolateral Na + -Ca 2+ exchanger. Furthermore, the hypocalciuric effect of thiazides persists in mice deficient in TRPV5, arguing against the putative effects of this drug on distal Ca 2+ absorption. Rather, several lines of evidence have argued that the hypocalciuria of GS and thiazide treatment is due to increased absorption of Na + by the proximal tubule, with secondary increases in proximal Ca 2+ absorption. Regardless, reminiscent of the clinical effect of thiazides on bone, there are clear differences in bone density between affected and unaffected members of specific Gitelman kindreds. Thus, homozygous patients have much higher bone densities than unaffected wild-type family members, whereas heterozygotes have intermediate values for bone density and calcium excretion. An interesting association has repeatedly been described between chondrocalcinosis, the abnormal deposition of calcium pyrophosphate dihydrate (CPPD) in joint cartilage, and GS. Patients have also been reported with ocular choroidal calcification.
Finally, as in BS, there have been reports of acquired tubular defects that mimic those of GS. These include patients with hypokalemic alkalosis, hypomagnesemia, and hypocalciuria after chemotherapy with cisplatin. Patients have also been described with acquired GS due to Sjögren’s syndrome and tubulointerstitial nephritis, with a documented absence of coding sequence mutations in NCC.
Renal Tubular Acidosis
Renal tubular acidosis (RTA) and related tubular defects can be associated with hypokalemia. Proximal RTA is characterized by a reduction in proximal bicarbonate absorption, with a reduced plasma bicarbonate concentration. Isolated proximal RTA is rare; genetic causes include loss of function by mutations in the basolateral Na + -HCO 3 − transporter. More commonly, proximal RTA occurs in the context of multiple proximal tubular transport defects, encompassing Fanconi’s syndrome (FS). The cardinal features of FS include hyperaminoaciduria, glycosuria with a normal plasma glucose concentration, and phosphate wasting; associated defects include the proximal RTA, hypouricemia, hypercalciuria, hypokalemia, salt wasting, and increased excretion of low-molecular-weight proteins. FS is usually drug-associated; important causes include aristolochic acid, ifosfamide, and the acyclic nucleoside phosphonates (e.g., tenofovir, cidofovir, adefovir). Prior to treatment with bicarbonate, patients with a proximal RTA will typically demonstrate mild hypokalemia, due primarily to baseline hyperaldosteronism ; however, prior to treatment, patients have been described with profound hypokalemia on presentation. Regardless, treatment with oral sodium bicarbonate will markedly increase distal tubular Na + and HCO 3 − delivery, causing a marked increase in renal potassium wasting. Patients will often require mixed base replacement with oral citrate and bicarbonate in addition to aggressive K + -Cl − supplementation.
Hypokalemia is also associated with distal RTA, the so-called type 1 RTA. Hypokalemic distal RTA is most commonly due to a secretory defect, with reduced H + -ATPase activity and decreased ability to acidify the urine. For example, hereditary defects in subunits of H + -ATPase are associated with profound hypokalemia in addition to acidosis and hypercalciuria. Pathophysiology of the associated hypokalemia is multifactorial due to the loss of electrogenic H + secretion (with enhanced K + secretion to maintain electroneutrality in the distal nephron), loss of H + -K + -ATPase activity, and increases in aldosterone. Sjögren’s syndrome is perhaps the most common cause of hypokalemic distal RTA in adults; the associated hypokalemia can be truly profound, often resulting in marked weakness and respiratory arrest.
Magnesium Deficiency
Magnesium deficiency results in refractory hypokalemia, particularly if the plasma Mg 2+ level is less than 0.5 mmol/L ; hypomagnesemic patients are thus refractory to K + replacement in the absence of Mg 2+ repletion. Magnesium deficiency is also a common concomitant of hypokalemia, in part because associated tubular disorders (e.g., aminoglycoside nephrotoxicity) may cause kaliuresis and magnesium wasting. Plasma Mg 2+ levels must therefore be checked on a routine basis in hypokalemia.
Several mechanisms appear to contribute to the effect of magnesium depletion on plasma K + . Magnesium depletion has inhibitory effects on muscle Na + -K + -ATPase activity, resulting in significant efflux from muscle and a secondary kaliuresis. Distal K + secretion also appears to be enhanced due to a reduction in the normal physiologic inward rectification of ROMK secretory K + channels, with a subsequent increase in outward conductance. ROMK and other Kir channels are inward-rectifying—that is, K + flows inward more readily than outward; even though outward conductance is usually less than inward conductance, K + efflux predominates in the CNT and CCD since the membrane potential is more positive than the equilibrium potential for K + . Intracellular Mg 2+ plays a key role in inward rectification, binding and blocking the pore of the channel from the cytoplasmic side. The hypomagnesemia-associated reduction in cytoplasmic Mg 2+ in principal cells reduces inward rectification of ROMK, increasing outward conductance and increasing K + secretion; this has been confirmed in vivo. Finally, it has been suggested that the repletion of intracellular K + is impaired in hypomagnesemia, even in normokalemic patients. Decreased intracellular Mg 2+ enhances K + efflux from the cytoplasm of cardiac and perhaps skeletal myocytes, likely due to reduced intracellular blockade of inward rectifying K + channels (increased efflux) and inhibition of Na + -K + -ATPase (decreased influx); plasma K + levels thus remain normal at the expense of intracellular K + . This phenomenon is particularly important in patients with cardiac disease who are taking both diuretics and digoxin. In such patients, hypokalemia and arrhythmias will respond to a correction of the magnesium deficiency and potassium supplementation.
Clinical Approach to Hypokalemia
The initial priority in the evaluation of hypokalemia is an assessment for signs and/or symptoms (e.g., muscle weakness, changes in the electrocardiogram [ECG]) suggestive of an impending emergency that requires immediate treatment. The cause of hypokalemia is usually obvious from the history, physical examination, and/or basic laboratory tests. However, persistent hypokalemia, despite appropriate initial intervention, requires a more rigorous workup; in most cases, a systematic approach reveals the underlying cause ( Figure 18.11 ).

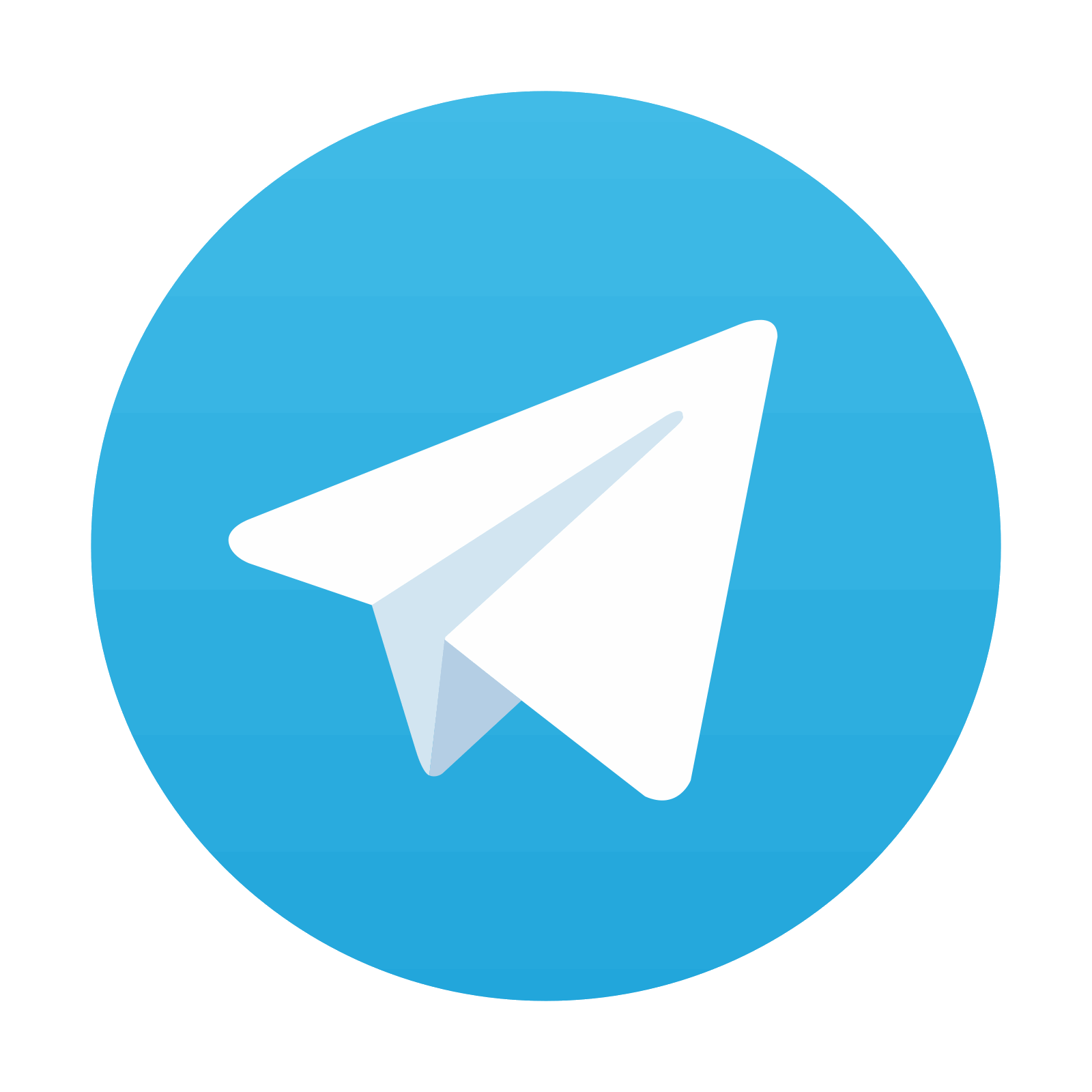
Stay updated, free articles. Join our Telegram channel
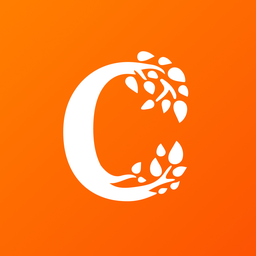
Full access? Get Clinical Tree
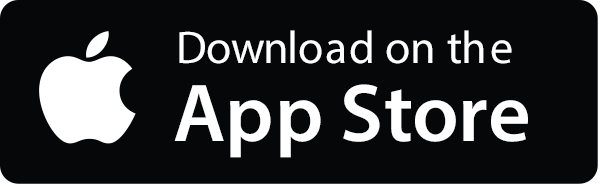
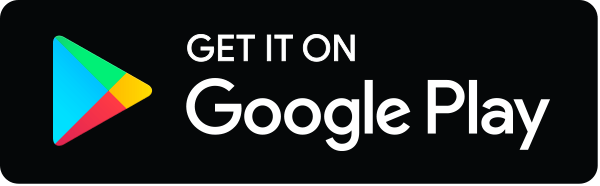
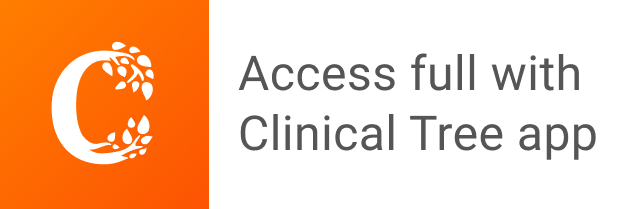