CHAPTER 100 Digestion and Absorption of Nutrients and Vitamins
Most nutrients are absorbed with remarkable efficiency: Less than 5% of ingested carbohydrate, fat, and protein is excreted in the stool of adults consuming a normal diet.1 Even much of the indigestible dietary fiber is absorbed from the colon as short-chain fatty acids that are liberated by bacterial breakdown of fiber.2 The intestinal tract of neonates is less efficient: infants fail to absorb 10% to 15% of their dietary fat, and in premature infants as much as 25% to 35% of fat may be lost in the stool.3,4 In old age, nutrient absorption remains highly efficient unless the intestine becomes diseased.
Despite considerable variations in types of food ingested and nutritional intake across national and racial groups, absorption remains efficient. Absorptive mechanisms adapt to the nature and amount of various nutrients presented to the intestinal tract. Such changes occur not only during early development5 but also throughout life and at times of specific need, such as during pregnancy.6 In achieving the overall objective of nutrient absorption, the different parts of the gastrointestinal tract act in a closely integrated and coordinated manner under the control of neural and humoral regulatory mechanisms.
DIGESTION AND ABSORPTION OF NUTRIENTS
AN OVERVIEW OF THE DIGESTIVE PROCESS
The cerebral phase of digestion, whether triggered by the sight, smell, or thought of food, initiates the digestive process. Salivary and gastric secretory responses to this type of stimulus are mediated via the autonomic nervous system, and there is modest stimulation of pancreaticobiliary secretion via the vagus nerve.7 The further stimulus of nutrients in the mouth and upper gastrointestinal tract markedly potentiates secretion by humoral and local neural mechanisms (see Chapter 1).8
The rapidity with which food is normally chewed and swallowed affords little time for significant oral digestion of nutrients; however, good mastication and mixing with saliva initiates digestion of starch by salivary amylase. In infants, digestion of fat is begun in the stomach by gastric lipase. Gastric acid would soon switch off these enzymes were it not for the buffering capacity of food that allows some digestion to continue. The optimal pH for gastric lipases is 4.5 to 6.0, and it has been suggested that a considerable portion of dietary triglyceride may be digested by these lipases.9,10 Protein digestion begins in the stomach when gastric pepsinogens are converted to pepsins by gastric acid. Pepsins become increasingly active as intraluminal pH falls and therefore the digestive action of pepsins on proteins is restricted to the stomach.
During ingestion of food, the stomach may become distended, but intragastric pressure rises little because of neurally mediated receptive relaxation. The mechanisms by which subjects perceive satiety and, therefore, cease eating are complex and explained only partly by the sensation of fullness. Although dozens of enzymes and hormones are secreted by the gastrointestinal tract in response to intraluminal food, only a few are able to influence food intake directly. Satiety signals are relayed to the hindbrain, either indirectly via nerves such as the vagus nerve or else directly via the blood. Most factors that influence how much food is eaten during individual meals act by changing the sensitivity to satiety signals.11
Cholecystokinin (CCK), gastrin-releasing peptide, and apolipoprotein A-IV (apo A-IV) have all been implicated as messengers that transmit the satiety signal to the central nervous system (CNS).12,13,14 They potentiate each other’s actions, and a combination of these agents may participate in the satiety signal. The administration of exogenous CCK or other satiety signals causes smaller meals to be consumed, whereas blocking the action of endogenous CCK and other satiety signals allows larger meals to be consumed.11,15,16 Additional peptides, known as the anorectic peptides, including peptide YY (PYY), pancreatic polypeptide (PP), glucagon-like peptide 1 (GLP-1), and oxyntomodulin also have been shown to decrease appetite and promote satiety in animal and human models.17
Apo A-IV is a glycoprotein synthesized by the enterocytes of human intestine and the hypothalamus, especially the arcuate nucleus. Intestinal apo A-IV synthesis is markedly stimulated by fat absorption and does not appear to be mediated by the uptake or re-esterification of fatty acids to form triglycerides. The local formation of chylomicrons acts as a signal for the induction of intestinal apo A-IV synthesis. Intestinal apo A-IV synthesis is also enhanced by a factor from the ileum (probably peptide tyrosine-tyrosine [PYY]), as well as neuropeptide Y (NPY) and pancreatic polypeptide (PP).18 Inhibition of food intake by apo A-IV is mediated centrally. The stimulation of intestinal synthesis and secretion of apo A-IV by lipid absorption are rapid; thus apo A-IV plays a role in the short-term regulation of food intake. There also is evidence suggesting that apo A-IV may be involved in the long-term regulation of food intake and body weight, because it is influenced by leptin and insulin. Chronic ingestion of a high-fat diet blunts the intestinal and the hypothalamic apo A-IV response to lipid feeding.19 Hypothalamic apo A-IV level is reduced by food deprivation and restored by lipid feeding.20,21
Leptin, a hormone released from fat cells, is an important peripheral signal from fat stores that modulates food intake by acting on receptors in the arcuate nucleus and hypothalamus.22 Leptin deficiency and leptin receptor defects produce massive obesity. Only one gastrointestinal signal, ghrelin, has been shown to increase appetite.11
The major digestive processes are initiated in the duodenum. Delivery of chyme from the stomach is delicately adjusted so that it enters the duodenum at a controlled rate, thus allowing efficient mixing with pancreaticobiliary secretions. Control of gastric emptying is thus critical to ensuring optimal digestion. The characteristics of gastric contents that determine the rate at which the stomach empties include their consistency, pH, osmolality, and lipid and calorie content (Fig. 100-1).23 The pylorus is selective in that it allows rapid passage of liquids while retaining solid particles with diameters of 2 mm or larger.24 Thus, large particles are retained and progressively reduced in size by the gastric mill, a process referred to as trituration. Trituration ensures that particles will be small enough to allow them reasonably close apposition to digestive enzymes once the nutrient is allowed to enter the duodenum. Meals of high viscosity empty more slowly than do those of low viscosity.
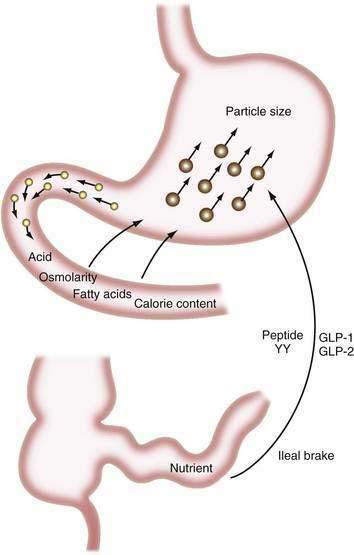
Figure 100-1. Some factors that delay gastric emptying. Receptors for acid, osmolarity, fatty acids, and other nutrients in the duodenum signal gastric delay via neurohumoral mechanisms. Food particles larger than 2 mm in diameter (large circles) are rejected by the antrum. Nutrients in the ileum and colon also influence gastric emptying by the ileal brake mechanism (see Chapter 48). GLP, glucagon-like peptide; peptide YY, peptide tyrosine-tyrosine.
Duodenal mucosal receptors for pH and osmolality trigger a delay in gastric emptying when the gastric effluent is acidic or hyper- or hypotonic.25,26 When duodenal luminal contents are neutralized by pancreaticobiliary bicarbonate and osmolality is adjusted by water fluxes, gastric emptying is encouraged once more. This careful titration in the duodenal lumen ensures that nutrients are presented optimally to the pancreatic enzymes, which function best at neutral pH.
The total calorie content of meals also controls gastric emptying rates; on average, the human stomach delivers about 150 kcal/hr to the duodenum.27 An increase in the size or energy density of a meal leads to a corresponding increase in the rate of delivery. Receptors for fatty acids, amino acids, and carbohydrates in the duodenal mucosa are involved in this response, which probably is mediated by both neural and humoral feedback mechanisms.28
Gastric emptying additionally is controlled by a mechanism involving the ileum and colon. If much nutrient escapes digestion and absorption in the jejunum, its presence in the ileum and colon delays gastrointestinal transit, and this again provides more time for digestion and absorption.29,30 This ileal brake probably is mediated by a neurohumoral mechanism, for which various neurotransmitters and hormones have been implicated including peptide YY and the glucagon-like peptides-1 and -2 (GLP-1 and GLP-2).31,32
The GLPs are synthesized in and cosecreted from enteroendocrine cells in the small and large intestine in response to luminal carbohydrate and fat. GLP-1 promotes efficient nutrient assimilation by decreasing appetite, slowing gastric empting and enhancing glucose-induced insulin secretion. GLP-2 is cosecreted with GLP-1and also regulates energy absorption by its effects on nutrient intake, gastric acid secretion, gastric emptying, and nutrient absorption. Circulating levels of GLP-1 and GLP-2 are low in the fasted state and increase rapidly following ingestion of nutrients.33–37
The gallbladder is stimulated to contract and the pancreas to secrete simultaneously in response to the presence of nutrients in the duodenal lumen. A range of nutrient receptors stimulates the release of CCK and secretin from mucosal endocrine cells into the portal circulation, and these are largely responsible for this response. Exocrine pancreatic secretion is primarily controlled by cephalic mechanisms (the vagus nerve), gastric mechanisms (acid and pepsin secretion, and nutrients delivered into the duodenum by gastric emptying), and intestinal mechanisms (secretin and CCK) (see Chapter 56).
CCK and other enterohormones stimulate the pancreas by excitation of sensory nerves and by triggering of long vagovagal or enteropancreatic reflexes. Numerous neurotransmitters, such as acetylcholine and nitric oxide, and certain neuropeptides, such as gastrin-releasing peptide (GRP), generated by neurons of the enteric nervous system, have been implicated in the regulation of the exocrine pancreas. CCK appears to act via vagal cholinergic pathways to mediate pancreatic enzyme secretion. Human pancreatic acini lack functional CCK-A receptors, explaining why a CCK infusion that produces plasma CCK levels similar to those seen postprandially stimulates pancreatic exocrine secretion by an atropine-sensitive pathway.38 Under physiologic conditions, cholinergic vagal afferent pathways rather than pancreatic acinar cells represent the primary targets on which CCK can act as a major mediator of postprandial pancreatic secretion.38
Serotonin (5-hydroxytryptamine, 5-HT) released from enterochromaffin cells in the intestinal mucosa and nerve terminals of the enteric nervous system and the intra-pancreatic nerves may be involved in both stimulation and inhibitory mechanisms through its various receptor subtypes; 5-HT also mediates the actions of secretin and CCK. A synergistic interaction between CCK and 5-HT at the level of the nodose ganglia might explain the robust postprandial pancreatic secretion despite a modest postprandial increase in plasma CCK. Peptides affecting appetite and originating from the intestine (e.g., leptin and ghrelin) or from the pancreas (e.g., PP and NPY) appear to modulate the exocrine pancreas via hypothalamic centers.39,40
Pancreatic juice provides both positive and negative feedback regulation of pancreatic secretion through mediation of both secretin- and CCK-releasing peptides. Three CCK-releasing peptides have been purified: monitor peptide from pancreatic juice, diazepam-binding inhibitor from porcine intestine, and luminal CCK-releasing factor from rat intestinal secretion. All have been shown to stimulate CCK release and pancreatic enzyme secretion. Pancreatic phospholipase A2 from pancreatic juice and intestinal secretions appears to function as a secretin-releasing peptide.41
Adequate lipid digestion is critically dependent on the presence of bile salts and pancreatic lipase and colipase at nearly neutral pH,42 whereas digestion of carbohydrate and protein depends on the combined actions of intraluminal secreted enzymes and then enzymes sited on the brush border membrane and within the intestinal mucosa. The close physical relationship, at the brush border, between the sites for terminal digestion of protein and carbohydrate and the active absorption of digestive products provides a very efficient mechanism for dealing with these nutrients.
Two other simultaneous phenomena encourage efficient digestion and absorption. Ingestion of a meal stimulates salt and water secretion by the jejunal mucosa, and this maintains luminal contents in a sufficiently fluid state for proper mixing and digestion (see Chapter 99).43 The other phenomenon is the motor response of the intestine. After feeding, the characteristic repetitive pattern of motility that occurs during fasting is disrupted. Instead, an apparently disordered pattern is seen, which, presumably, ensures that nutrients are well mixed and brought into close contact with intestinal mucosa (see Chapter 97). There is close integration of the neurohumoral control mechanisms involving the motor and secretory responses of the intestine.44 For rapidly absorbed molecules, intestinal blood flow may be the rate-limiting step.45
Efficient conservation and recycling mechanisms ensure that gastrointestinal secretions are not entirely lost. Gastric acid secretion is balanced to a large extent by pancreaticobiliary bicarbonate secretion, so that overall acid-base balance is not disturbed. Although intact digestive enzymes are reabsorbed only in trace amounts, the nitrogen they contain is reabsorbed after their digestion. Finally, efficient enterohepatic circulation recycles bile salts several times each day so that they may be used approximately twice for each meal.46 Although bile salts are passively reabsorbed throughout the small intestine, most reach the terminal ileum, where they are reabsorbed via specific active absorptive mechanisms. Thus, bile salts remain in the lumen where they are needed for lipid digestion, but they are largely reabsorbed at the last moment to avoid being lost by the colon (Fig. 100-2; see Chapter 64).
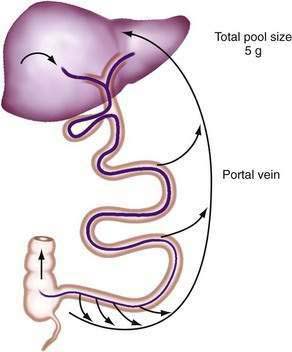
Figure 100-2. Enterohepatic circulation of bile salts. Active transport in the ileum retrieves most bile salts, and the small fraction lost into the colon and eliminated in the feces is compensated for by fresh hepatic synthesis (see Chapter 64).
FAT
DIETARY INTAKE
In the United States, fat intakes for all age groups and both sexes rose from approximately 34% of total energy consumption in the 1930s to 40% to 42% in the late 1950s to mid-1960s, and then fell steadily to approximately 36% (90 to 100 g/day) in 1984. Saturated and monounsaturated fatty acid intakes fell from 18% to 20% of total energy consumption in the early 1950s to 12% to 13% of energy in 1984; polyunsaturated fatty acid intakes rose from 2% to 4% of energy to 7.5%.47
The majority of fatty acids present in dietary triglyceride are oleate and palmitate (18 : 1 and 16 : 0, respectively).48 In animal triglyceride, most of the fatty acids are long-chained (i.e., longer than 14 carbon chains) and saturated (Fig. 100-3). Polyunsaturated fatty acids such as linoleic and linolenic acid are derived from phospholipids of vegetable origin and, because they cannot be synthesized de novo, they are considered essential fatty acids (Table 100-1).
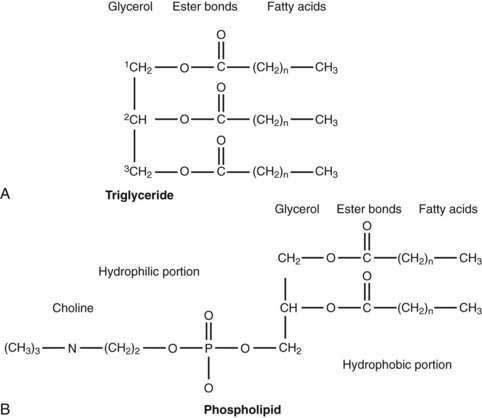
Figure 100-3. General molecular structure of triglyceride (A) and phospholipid (phosphatidyl choline or lecithin) (B).
Table 100-1 Common Dietary Fatty Acids
FATTY ACID | CONFIGURATION* |
---|---|
Saturated Fatty Acids | |
Butyric | 4 : 0 |
Caproic | 6 : 0 |
Lauric | 12 : 0 |
Myristic | 14 : 0 |
Palmitic | 16 : 0 |
Stearic | 18 : 0 |
Mono-unsaturated Fatty Acids | |
Oleic | 18 : 1 |
Palmitoleic | 16 : 1 |
Polyunsaturated Fatty Acids | |
Arachidonic | 20 : 4 |
Linoleic | 18 : 2 |
Linolenic | 18 : 3 |
* By convention, the number of carbon atoms in the chain is given by the first figure and the number of double bonds in the chain is given by the second.
The average range of phospholipid ingestion is between 2 and 8 g/day. The most commonly ingested phospholipid is phosphatidyl choline (lecithin), and the predominant fatty acids in phospholipid are linoleate and arachidonate (see Fig. 100-3). More phospholipid is found in the duodenal lumen (10 to 22 g/day) than is ingested, most of which is derived from endogenous sources, particularly bile. Cholesterol intake varies widely but averages about 200 to 250 mg/day.48 Some people consume as much as 500 mg/day.
Commercial hydrogenation of unsaturated bonds in the fatty acids of natural oils raises their melting points, thus allowing production of margarines and spreads of variable consistency. Hydrogenation, in addition to saturation, results in isomerization of cis to trans double bonds.49 Although many commercial products contain partially hydrogenated fats, the content of trans-fatty acids in some margarines exceeds 60%, thus raising concerns about their relationship to cancer induction.50
DIGESTION AND ABSORPTION
Most dietary lipid is absorbed by the upper two thirds of jejunum, although its rate and extent of absorption are influenced by the presence of other foods, particularly dietary fiber, which reduces the rate of absorption.51 The types of ingested fat also appear to influence the absorptive process, both by modifying the morphologic structure of the intestinal mucosa and by influencing its absorptive function for other nutrients such as carbohydrate.52
Triglyceride
Liberation of fatty acids from the glycerol backbone of triglycerides (lipolysis) is achieved by lipases acting at the surface of emulsified droplets (Table 100-2). This process occurs initially in the stomach, but most lipolysis is accomplished in the small intestine.53 Intragastric lipolysis might account for 20% to 30% of total intraluminal lipid digestion.54 Gastric lipase, which is of fundic origin, has been demonstrated in the gastric contents of premature neonates and in mucosal biopsy specimens from adults up to 80 years of age. Gastric lipase does not hydrolyze phospholipids or cholesterol esters. Human gastric lipase is a 379-amino acid protein that shares similar homology with rat gastric lipase but not human pancreatic lipase. For either gastric or small intestinal lipolysis to occur, two conditions are critical: First, a stable emulsion is required of fat droplets of such a size that they present a large surface area to the digestive enzyme; second, a mechanism is required for bringing enzyme and triglyceride into close apposition within the emulsion.
Emulsification
A number of factors assist in optimal production of an emulsion. Physical release of fat by mastication and the gastric milling of food produces a relatively unstable emulsion that is delivered into the duodenum. To permit its stabilization, the droplets in this emulsion have to be coated, and dietary phospholipid provides one such coat. The ratio of ingested phospholipid to triglyceride is about 1 : 30, and more phospholipid is added in the duodenum from bile.55 In breast milk, emulsion droplets are smaller and have proteins as well as phospholipid incorporated into their surface trilayer.56 Emulsification also is enhanced by the fatty acids liberated by intragastric lipolysis and, within the duodenum, by bile salts (Fig. 100-4). The final product in the duodenum is an emulsion consisting predominantly of triglyceride together with cholesterol esters and some diglyceride and coated by phospholipid, partially ionized fatty acids, monoglyceride, and bile salts.
Lipase
This stable emulsion is then presented to pancreatic triglyceride lipase. Unlike other soluble enzymes, which can act in a three-dimensional solution, lipase has to act at the two-dimensional surface of the emulsion droplet, and this requirement poses particular problems.57 Certain characteristics of the enzyme itself are important. Thus, the lipolytic zone of the molecule is hydrophobic and lies deep within it, shielded from the aqueous phase. It is revealed to the lipid only on close apposition to its surface. The presence of a coat on the lipid droplet thus poses a barrier to the action of lipase, and assistance is required to bring it into close contact with the triglyceride.
The presence of colipase, cosecreted by the pancreas along with lipase in a molar ratio of 1 : 1, is critical in approximating lipase to triglyceride (see Fig. 100-4). Colipase attaches to the ester bond region of the triglyceride, lipase then binding strongly to colipase by electrostatic interactions.55 Phospholipase A2 digestion of the phospholipid on the surface of the lipid emulsion allows exposure of the triglyceride core to the colipase-lipase complex, further enhancing colipase-dependent anchoring of lipase to the lipid emulsion. Phospholipase A2 digestion requires bile salts and Ca2+ for activation, which can further assist colipase-lipase–mediated triglyceride lipolysis by providing a mechanism for removing lipolytic products. In the absence of colipase, bile salts on the surface of the emulsion droplet inhibit lipase activity.
The colipase gene is located on chromosome 6, and the amino acid sequence of the lipid-binding domain, the lipase-binding domain, and the activation peptide appear to be highly conserved.58 Colipase is secreted by the pancreas as pro-colipase,59 which is activated when trypsin cleaves a pentapeptide from its N-terminus after entering the small intestinal lumen. A valine residue at position 407 and a leucine at position 412 are important for the interaction of lipase with colipase and the bile salt micelles.60 Interestingly, the pentapeptide cleaved from the pro-colipase by trypsin, called enterostatin, seems to be a specific satiety signal for the ingestion of fat.61
Because pancreatic lipase is most active at nearly neutral pH, secretion of bicarbonate by the pancreas and biliary tree is critically important and provides the necessary neutralization of gastric acid; however, luminal pH falls to about 6 in the jejunum, and here the fact that bile salts lower the pH optimal for lipase activity from 8 to 6 may be significant. In the presence of colipase and optimal pH, lipase activity releases fatty acids and monoglyceride extremely rapidly and efficiently (see Fig. 100-4). Pancreatic triglyceride lipase also binds strongly to the mucosal brush border membrane,62 where it may participate in lipolysis of cholesteryl esters or triglyceride, releasing fatty acids, monoglyceride, and free cholesterol in proximity to the brush border membrane, where they undergo rapid uptake.
In addition to pancreatic triglyceride lipase and its protein cofactor, colipase, pancreatic acini also synthesize two pancreatic lipase-related proteins (PLRP-1 and PLRP-2), which have strong nucleotide and amino acid sequence homology to pancreatic triglyceride lipase. Although PLRP-1 has no known activity, PLRP-2 does have lipase activity and, like pancreatic triglyceride lipase, PLRP-2 cleaves triglycerides but with broader substrate specificity. PLRP-2 also hydrolyzes phospholipids and galactolipids, two fats that are not substrates for pancreatic triglyceride lipase. It is also different from pancreatic triglyceride lipase with respect to sensitivity to bile salts and in response to colipase. A further critical difference is that PLRP-2 mRNA appears before birth and persists into adulthood, whereas pancreatic triglyceride lipase mRNA first appears at the suckling-to-weaning transition. This suggests that PLRP-2 plays a critical role in the digestion of breast-milk fats.63,64
Micelles and Other Lipid-Containing Particles
The products of lipolysis are distributed among the aqueous, oil, and intermediate phases in a number of forms prepared for transfer across the lumen to the mucosal brush border membrane. The shuttling of these products depends, in part, on the formation of micelles with bile salts. The concentration of bile salts secreted in bile is about 35 mmol and, in the duodenum, this is further decreased by dilution to 10 to 20 mmol; this concentration lies well above the critical concentration for micelle formation. Mixed micelle production depends on a number of other factors, including pH, presence or absence of lipids, and the types of bile salts that are secreted (see Chapter 64).42
Bile salts are capable of forming micelles because they have a particular three-dimensional structure and they are amphipathic; that is, their molecules have both water-soluble and lipid-soluble portions (see Fig. 100-4). They orient themselves at an oil-and-water interface and thus are ideal emulsifying agents. In addition, micelles are formed when bile salt levels are present above critical concentrations and thus are able to aggregate in disk-like particles with their hydrophobic sterolic backbones oriented toward each other and their hydrophilic polar groups facing outward into the aqueous phase. Bile salt micelles have the capacity to dissolve fatty acids, monoglycerides, and cholesterol, but not triglyceride.65 The mixed micelles thus formed are arranged so that the insoluble lipid is surrounded by bile salts that are oriented with their hydrophilic groups facing outward. Mixed micelles are about 50 to 80 nm in diameter and, unlike emulsion droplets, are too small to scatter light; thus, micellar solutions are clear. The presence of phospholipid secreted in bile enlarges mixed micelles and makes them more efficient in the dissolution of fat.
Other lipid-containing particles participate in the transfer of lipid to the mucosa. As the emulsion droplet shrinks during lipolysis, liquid crystalline structures are formed at its surface.66,67 These vesicular structures with multilamellar and unilamellar forms can be seen under the electron microscope, budding off the surface of emulsion droplets and occasionally close to the brush border membrane of the intestinal mucosa.68 This physical phase of lipid within the lumen might provide a significant mechanism for transfer of lipid to the mucosa, beyond that provided by bile salt micelles, and it could explain the observation that in the absence of bile salts, some 50% or more of dietary triglyceride may be absorbed. In the presence of adequate concentrations of bile salts, however, these vesicles undergo rapid spontaneous dissolution and release their lipid into micelles, which are likely to be the major route for lipid traffic (see Fig. 100-4); numerically, they are much more common than lipid vesicles.
Importance of Intraluminal pH
Lipid digestion and absorption are highly dependent on intraluminal pH at several steps in the process. Pancreatic lipase operates best in the presence of bile salts and at least pH 6. It therefore functions well at the pH of the luminal duodenum, where most lipid digestion occurs. Glycine-conjugated bile salts precipitate below pH 5; fatty acids are in their protonated form below about pH 6 and have limited solubility in bile salt micelles. Thus, in conditions in which intraluminal pH becomes more acid, as for example in the Zollinger-Ellison syndrome, pancreatic lipase is inactive, bile acids precipitate out of solution, and fatty acid partitioning is reduced. It is not surprising, therefore, that steatorrhea (without any other nutrient or hematologic disturbances) is a feature of this syndrome. Biological characteristics of lipases, including effect of pH on activities, are detailed in Table 100-2.
Unstirred Water Layer
An unstirred water layer is present on the surface of the intestinal epithelium, which in humans is approximately 40 µm deep.69 This layer may be rate limiting for uptake of long-chain fatty acids but not for short- or medium-chain fatty acids, the limiting step for which occurs at the brush border membrane.55 The provision of a high concentration of fatty acid in the microenvironment adjacent to the epithelium depends on the diffusion of micelles into this region. The microclimate here is slightly acidic, owing to activity of a sodium-hydrogen (Na+/H+) exchanger at the brush border membrane, and at pH between 5 and 6, the solubility of fatty acids in micelles decreases, thus encouraging liberation of fatty acids close to the mucosa. The high concentration of fatty acids necessary for diffusion across the mucosal membrane is thus achieved; evidence for this model is increasingly persuasive.70 The low-microclimate pH also encourages the fatty acids to be presented in an undissociated, protonated form. Thus, the pH partition hypothesis predicts that fatty acids could diffuse passively into the cell as protonated species and, at the near-neutral intracellular pH, become trapped in the ionized form.
A surfactant-like material has been discovered close to the brush border membrane, although its role in absorption, if any, is uncertain.71 It is secreted by enterocytes, contains phosphatidylcholine and alkaline phosphatase, and appears as flat lamellae or vesicles adjacent to the brush border membrane.
Other Lipids
Phosphatidylcholine, the major dietary phospholipid, is hydrolyzed by pancreatic phospholipase A2 (PLA2) to yield fatty acid from the 2-position and lysophosphatidylcholine. Pancreatic PLA2 is secreted as an anionic zymogen that is activated in the small intestine by tryptic cleavage of an N-terminal heptapeptide. It has a molecular weight of approximately 14 kd and requires calcium for activation and bile salts for its activity. It has multiple isoforms and apparently requires a 2 : 1 bile salt-to-phosphatidylcholine molar ratio for optimal activity. Although the bulk of intestinal PLA2 activity is derived from pancreatic juice, there is some contribution from the intestinal mucosa, where the enzyme is concentrated in the brush border.72
Cholesterol esters, in the presence of bile salts and calcium, are hydrolyzed by carboxyl ester lipase (CEL) (also known as pancreatic cholesterol esterase) to release the free sterol, in which form it is absorbed. Cell culture and other in vitro studies have thoroughly defined the potential functions of CEL in the digestion of cholesteryl ester, phospholipids, and triglycerides, but only its cholesteryl ester hydrolytic activity is unique to this enzyme in the digestive tract.73 CEL belongs to the α/β hydrolase family, is well conserved, and shares 78% homology in rats and humans.74 It is secreted primarily by the pancreatic acinar cells and lactating mammary glands. Using site-directed mutagenesis, the serine at position 194, the histidine at position 435, and the aspartic acid at position 320 have been shown to be important for CEL’s catalytic activity.73,75–77 The hydrolysis of water-insoluble substrates by CEL requires bile salt–containing 3α- or 7-α-hydroxy groups (e.g., cholate or chenodeoxycholate and their conjugates).78 The arginine-63 and arginine-423 sites play an important role in this bile salt–dependent process, but not in the bile salt–independent lysophospholipid hydrolytic activity of CEL.79
Transfer across the Brush Border Membrane
Much of the current understanding of the micellar solubilization and uptake of dietary lipids comes from the work of Hofmann and Borgstrom, who described the uptake of lipid digestion products by enterocytes.80 Further work by Carey discovered the coexistence of unilamellar liposomes with bile salt–lipid mixed micelles in the small intestine.81 Although the uptake of lipid digestion products by enterocytes has been accepted as a passive process, recent work has raised the possibility that some lipids may be taken up by enterocytes via carrier-mediated processes that are energy dependent.82
Studies with brush border membrane vesicles suggest that linoleic acid uptake occurs by facilitated diffusion.83 Absorption of oleic and arachidonic acid also appears to occur by a saturable process, suggesting the possibility of active transport. Several membrane proteins that increase the uptake of long chain fatty acids when overexpressed in cultured mammalian cells have been identified, the most prominent and best characterized of which are FAT/CD36, long-chain fatty acyl-CoA synthetases (LACS), and fatty acid transport proteins (FATPs/solute carrier family 27).84–87 The FATPs are transmembrane proteins that have been shown to enhance the cellular uptake of long-chain and very-long-chain fatty acids. In humans, FATPs comprise a family of six highly homologous proteins, hsFATP 1-6, which are found in all tissues of the body that use fatty acids.84,88,89 Although hsFATP1 is the best characterized of the FATPs, hsFATP4 is the only FATP expressed in the small intestine; it is localized to the apical brush border of the epithelial cells, where it is responsible for absorption of dietary lipids. Studies with cell lines and isolated enterocytes that overexpress FATP4 demonstrated that FATP4 is both necessary and sufficient for efficient uptake of long-chain and very-long-chain fatty acids.90 Detailed substrate studies based on 14C-labeled fatty acids have been presented for FATP1 and FATP4.87,90 Both studies showed that uptake of fatty acids shorter than 10 carbon atoms, such as butyric acid and octanoic acid, was unaffected by FATP expression, whereas uptake of common long-chain fatty acids, such as palmitate and oleate, was robustly enhanced.90 More recently, a wrinkle-free phenotype has been associated with the spontaneous autosomal recessive mutation of the gene for FATP4, resulting in a very tight and thick-skinned phenotype.91
Nutrients, hormones, and cytokines have been reported to regulate FATP expression. Rats fed a high-fat diet showed increased FATP expression in the heart, but not the liver. Several reports have shown a positive regulation of mouse FATP by ligands that activate either PPAR-γ, PPAR-α, or PPAR-γ/RXR heterodimers in hepatoma cell lines, the liver, and the intestine. Further, a PPAR binding site was identified in the murine FATP1 promoter. TNF-α is a negative regulator of FATP expression and down-regulates FATP mRNA in liver and FATP1 and FATP4 proteins in adipocytes.88
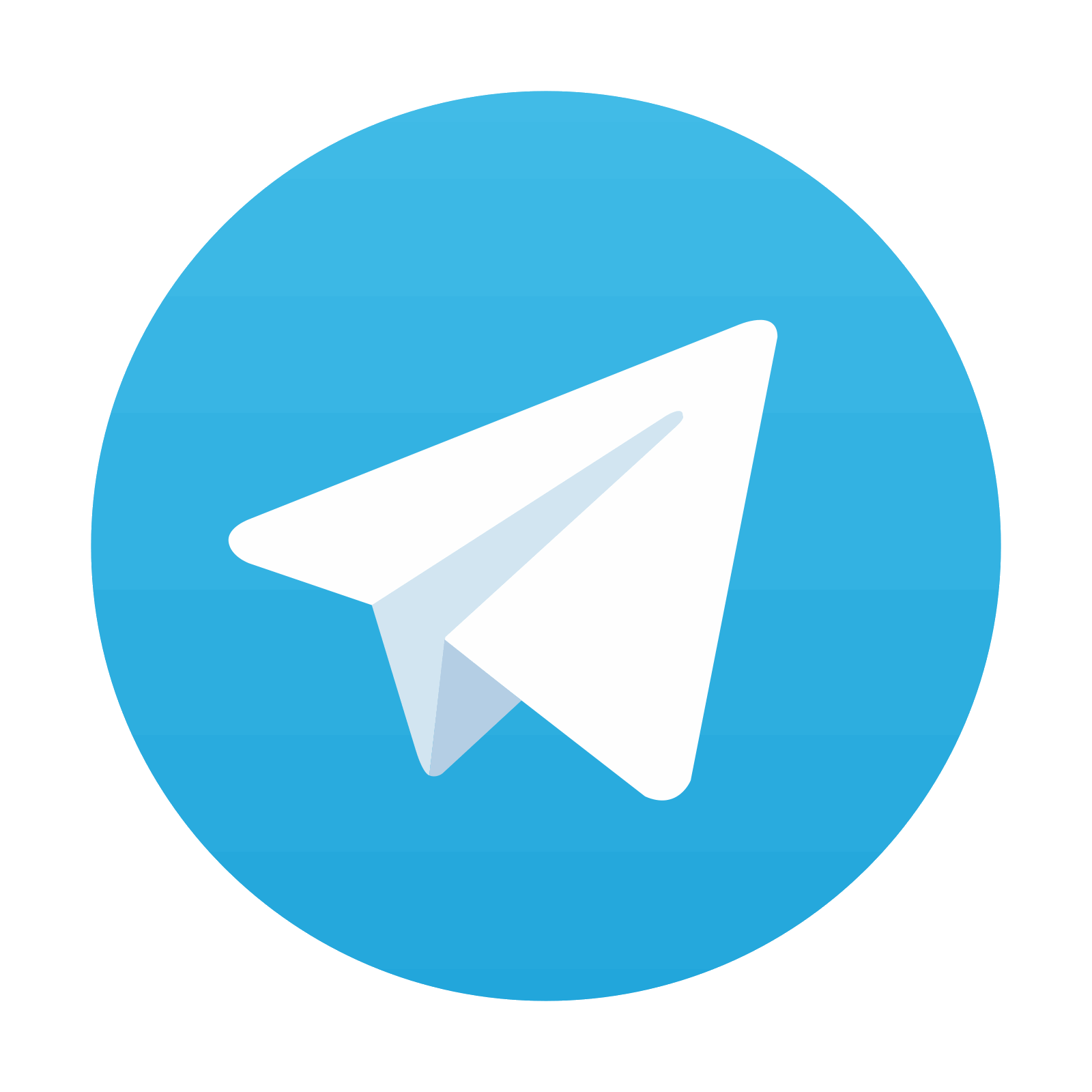
Stay updated, free articles. Join our Telegram channel
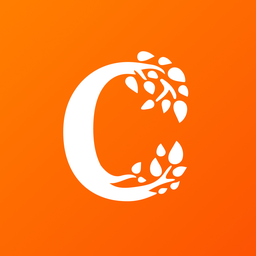
Full access? Get Clinical Tree
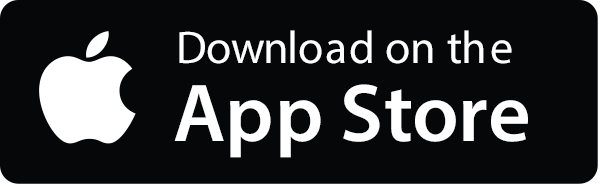
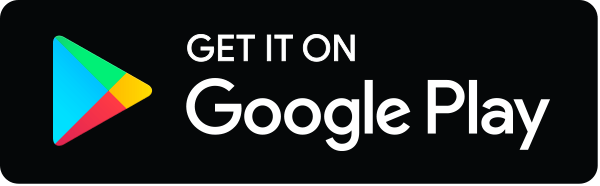