Abstract
Pathologies of mitochondrial structure and function are now recognized as the etiology of a growing list of monogenic mitochondrial disorders as well as contributing to many common diseases. Studying patients with respiratory chain disorders has contributed much to our current knowledge about mitochondrial biology [1]. Mutations impacting mitochondrial proteins as well as ribosomal and transfer RNAs (tRNAs) are the underlying cause of diseases affecting the nervous system, skeletal and cardiac muscle, the liver, bone marrow, the endocrine and exocrine pancreas, kidney, inner ear and small and large intestines (Table 35.1) [1]. Perturbations in mitochondrial function result in defective oxidative phosphorylation (OXPHOS) and ATP generation, increased generation of reactive oxygen species (ROS), accumulation of hepatocytic lipid, impairment of other mitochondria-based metabolic processes and activation of apoptotic, autophagic and necrotic cell death pathways [2]. The spectrum of genetic etiologies of inherited mitochondrial hepatic and gastrointestinal disorders continues to expand. In addition, mitochondrial dysfunction may be one of the key determinants of hepatocyte survival in other disorders that are not monogenic mitochondrial diseases. Thus, the concept of primary (or genetic) and secondary (or acquired) mitochondrial hepatopathies has developed.
Introduction
Pathologies of mitochondrial structure and function are now recognized as the etiology of a growing list of monogenic mitochondrial disorders as well as contributing to many common diseases. Studying patients with respiratory chain disorders has contributed much to our current knowledge about mitochondrial biology [1]. Mutations impacting mitochondrial proteins as well as ribosomal and transfer RNAs (tRNAs) are the underlying cause of diseases affecting the nervous system, skeletal and cardiac muscle, the liver, bone marrow, the endocrine and exocrine pancreas, kidney, inner ear and small and large intestines (Table 35.1) [1]. Perturbations in mitochondrial function result in defective oxidative phosphorylation (OXPHOS) and ATP generation, increased generation of reactive oxygen species (ROS), accumulation of hepatocytic lipid, impairment of other mitochondria-based metabolic processes and activation of apoptotic, autophagic and necrotic cell death pathways [2]. The spectrum of genetic etiologies of inherited mitochondrial hepatic and gastrointestinal disorders continues to expand. In addition, mitochondrial dysfunction may be one of the key determinants of hepatocyte survival in other disorders that are not monogenic mitochondrial diseases. Thus, the concept of primary (or genetic) and secondary (or acquired) mitochondrial hepatopathies has developed. The accumulation over time of new somatic (not inherited) mutations of mtDNA in various tissues is well-documented and while it is not certain that this is an etiology of age-related disease, it is clear that many aspects of mitochondrial dysfunction contribute to the common age-related diseases in the population [3]. In this chapter, we will review continued advances in our understanding of the genetics, the structure and function of mitochondria, classification of mitochondrial hepatopathies, the evolving diagnostic approach for these disorders, and treatment modalities including liver transplantation.
Cardiac: | Hypertrophic cardiomyopathy, arrhythmias, heart block, sudden death, Barth syndrome (cardiomyopathy, cyclic neutropenia) |
Eye: | Cataracts, optic atrophy, pigmentary retinopathy, ptosis, ophthalmoplegia |
Ear: | Sensorineural deafness, aminoglycoside deafness |
Renal: | Renal tubular dysfunction (Fanconi syndrome), nephritis, nephrotic syndrome |
Endocrine: | Diabetes mellitus, short stature, growth hormone deficiency, hypoparathyroidism, hypothyroidism, adrenal insufficiency, ovarian or testicular insufficiency |
Hematologic: | Pancytopenia, sideroblastic anemia, vacuolization |
Gastrointestine: | Pancreatic insufficiency, pancreatitis, intestinal villus atrophy, intestinal pseudo-obstruction, chronic diarrhea |
Neurologic: | Seizures, stroke-like episodes, ataxia, migraines, dementia, parkinsonism, developmental regression, peripheral neuropathy, dystonia, spasticity |
Muscular: | Weakness, exercise intolerance |
Dermatologic: | Mottled pigmentation, hypertrichosis, dry brittle hair, alopecia |
Metabolic: | Lactic acidosis, hyperammonemia |
Mitochondrial Structure and Genetics
The mitochondrion is a double membrane-enclosed organelle. The outer membrane serves to hold the highly folded inner membrane in place, to regulate transport of proteins and other molecules into the mitochondria and as the basis of the dynamic reshaping of the mitochondrial network in different cellular environments. The inner mitochondrial membrane also contains specific transporters and is the site of the respiratory chain complexes and ATP synthase. The mitochondrial matrix (contained by the inner membrane) is a concentrated mixture of enzymes that are active in the tricarboxylic acid (TCA) cycle, fatty acid oxidation, the urea cycle and other metabolic pathways with corresponding inherited metabolic diseases caused by specific enzyme deficiencies.
Mitochondria are unique among cellular organelles in that they contain a separate extranuclear genome (mtDNA) as well as the molecular machinery for DNA maintenance, replication, repair, transcription and translation [4]. The mitochondrial genome is a double-stranded, circular DNA molecule containing 16,569 base pairs that encode 37 genes, including two ribosomal RNAs, 22 tRNAs, and 13 of the subunits of OXPHOS complexes I, III, IV, and V (Table 35.2). In addition to the small number of mtDNA-encoded proteins and RNAs, there are 1,158 nuclear DNA-encoded genes whose products are translated outside of the mitochondria and then are trafficked in to contribute to mitochondrial function [5].
Table 35.2 Mitochondrial Respiratory Chain Protein Complexes: Polypeptide Subunits
Complex | Total Number of Subunits | Subunits Encoded by Nuclear DNA | Subunits Encoded by mtDNA |
---|---|---|---|
I | 41 | 34 | 7: ND1, ND2, ND3, ND4, ND4L, ND5, ND6 |
II | 4 | 4 | None |
III | 11 | 10 | 1: cyt. b |
IV | 13 | 10 | 3: cyt. Oxidase I, cyt. Oxidase II, cyt. Oxidase III |
V | 14 | 12 | 2: ATPase 6, ATPase 8 |
Unlike nuclear DNA (nDNA), mtDNA is transcribed as a polycistronic and intronless RNA molecule. Virtually all mtDNA in a developing embryo is derived from the unfertilized oocyte (sperm contribute virtually no mitochondria), and hence disease-causing mtDNA mutations are maternally inherited. About 25% of mtDNA mutations arise de novo in the affected individual and are not inherited. In particular, the vast majority (about 95%) of mtDNA deletions are de novo [6]. During development of the female germline cell populations, there is a reduction of number of mtDNA molecules within each oocyte followed by mtDNA replication to reach over 100,000 mtDNA genomes per mature oocyte. This “bottleneck” can result in wide variability in the prevalence (heteroplasmy) of mtDNA mutations between different oocytes and make counseling on recurrence risk for mtDNA-mediated disorders difficult [6].
A typical cell contains thousands of copies of mtDNA unlike the universal two copies of the nDNA genome per cell. If the sequence at a particular position is uniform among the copies of mtDNA, it is termed homoplasmic. If a percentage of mtDNA molecules have a base that differs from the others, this is referred to as heteroplasmy and is documented as the percentage of copies of mtDNA that have a mutation [4]. For pathogenic mutations, the phenotype of the cell (and hence the tissue) is determined by the relative proportion of normal to mutated mtDNA. mtDNA mutates 10–20 times as frequently as nDNA due to absence of protective histones and exposure to reactive oxygen species generated in the mitochondria, resulting in point mutations, deletions and duplications. During cell division, mitochondria are randomly partitioned into daughter cells, resulting in non-uniform distribution of mutated mtDNA in progeny cells.
The threshold for heteroplasmy of mutated mtDNA needed to produce a deleterious phenotype varies among individuals, organs, and within affected tissues as well as being variable by specific mtDNA mutation. The clinical phenotype associated with a mitochondrial mutation will depend on all of these factors and can be widely variable even within a single family. Mutations in the nuclear genes encoding proteins responsible for mtDNA maintenance and replication result in generalized depletion of mtDNA and ongoing accumulation of mtDNA point mutations and deletions over time. Assessment of mtDNA in individuals with these mtDNA maintenance disorders shows multiple different deletions at different levels of heteroplasmy.
Functions of Mitochondria
The essential functions of mitochondria are related to the multitude of enzyme systems located in the various compartments of the organelle [7]. Mitochondria from different organs exhibit distinct patterns of substrate use, biosynthetic capacities and different physical structure [8, 9]. A major function of mitochondria is to synthesize the ATP that drives energy-dependent reactions and transport processes in all cells. The transduction of energy by the transfer of electrons from the reactions of glycolysis, the TCA cycle (via NADH) and from the fatty acid oxidation cycle (via NADH and FADH2) to oxygen is facilitated by the respiratory chain, a group of four large protein complexes embedded in the inner mitochondrial membrane, plus ubiquinone (coenzyme Q; CoQ) and cytochrome c (Figure 35.1). These include Complex I (NADH-CoQ reductase), Complex II (succinate-CoQ reductase), Complex III (reduced CoQ-cytochrome c reductase) and Complex IV (cytochrome c oxidase) [1]. The free energy generated from these stepwise redox reactions is converted into a transmembrane proton gradient by the extrusion of protons through the inner membrane at complexes I, III, and IV. At complex V (ATP synthase), protons flow back into the mitochondrial matrix and the released energy drives ATP synthesis. When ADP and Pi are bound to the active site, protons are allowed to move down the concentration gradient and the free energy is enzymatically coupled to the formation of a bond between ADP and Pi. Three ATP molecules are generated for each molecule of NADH oxidized. Free NAD+ is regenerated for use in the TCA cycle and other integral mitochondrial matrix enzyme pathways. In addition to its role as the final electron acceptor in the respiratory chain, 2–3% of the oxygen utilized by mitochondria results in the generation of superoxide by complexes I and III. This superoxide is normally converted to hydrogen peroxide by the manganese-superoxide dismutase present in the mitochondrial matrix or combines with nitric oxide to form peroxynitrite. The hydrogen peroxide may diffuse into cytosol or remain in the mitochondrial matrix, only to be reduced to water by glutathione peroxidases present in both the mitochondria and cytoplasm. If the balance of generation and scavenging of these reactive oxygen species is upset, increased oxidative stress may develop within the mitochondria, damaging mitochondrial proteins, lipids and mtDNA [10].
Figure 35.1 The respiratory chain protein complexes and oxidative phosphorylation of the mitochondria. During glycolysis, fatty acid oxidation and the tricarboxylic acid cycle, reducing equivalents are derived from the sequential metabolism of each metabolic fuel. NADH acts as a carrier of reducing equivalents from glycolysis into the mitochondria matrix, and NADH and FADH2 shuttle reducing equivalents produced by fatty acid oxidation and the TCA cycle. Succinate carries reducing equivalents derived from the TCA cycle. In order to transduce this reducing power into energy, a system of electron carriers (protein complexes I through IV, coenzyme [Q] and cytochrome c) in the inner mitochondrial membrane convert the reducing equivalents into ATP through the efficient transport of electrons down this chain, resulting in the generation of a transmembrane proton gradient that drives the synthesis of ATP by complex V. FP: flavoprotein; Fe∙S: iron sulfur cluster; cyt: cytochrome.
Figure 35.2 Liver histology from an 11-week-old male with mtDNA depletion syndrome caused by POLG mutations. Biopsy was obtained at clinical presentation with diarrhea, weakness, failure to thrive and elevated AST, ALT, INR and plasma lactate. Histology shows mild hepatocyte swelling with scattered microvesicular steatosis and cholestasis. Portal tracts are normal and there is no fibrosis. (Hematoxylin and eosin stain, magnification 200×.)
Figure 35.3 Higher power views of liver histology from a patient with mtDNA depletion syndrome in Figure 35.2, showing microvesicular steatosis (arrows) and canalicular cholestasis (circles). (Periodic acid-Schiff-diastase stain, magnification 800×.)
Figure 35.4 Frozen section of liver biopsy in Figure 35.2, stained with Oil-red-O and showing marked microvesicular steatosis in most hepatocytes, despite benign appearance of the biopsy. (Magnification 100×)
Figure 35.5 Electron microscopy (original magnification 13,700×) of liver in mtDNA depletion syndrome patient from Figure 35.2. Small droplets of neutral lipid are present in hepatocyte. Virtually all mitochondria are abnormal with enlargement and pleomorphic size and shape, unusual cristae, and flocculent matrix.
Both primary and secondary defects in function of the respiratory chain can have severe consequences for the metabolic homeostasis of the cell [7]. These include a generalized deficiency of high energy molecules; a substantially increased dependence on glycolysis with increased lactate production in the cytosol; an increase in the intramitochondrial and cytoplasmic concentration of reducing equivalents; the functional impairment of the TCA cycle due to this altered redox state (an excess of NADH and lack of NAD); and an increase in the generation of oxygen free radicals with resultant oxidation of lipids in membranes, thiol-containing proteins and mitochondrial nucleic acids and opening of the mitochondrial permeability pore. The mitochondrial permeability transition releases cytochrome c and apoptosis inducing factors into cytosol, triggering the activation of caspases and the irreversible process of cellular apoptosis. In addition, the permeability transition results in loss of mitochondrial membrane potential and interruption of ATP synthesis. Thus, both metabolic failure and the induction of hepatocyte apoptosis or necrosis could result from impairment of normal electron flow in the respiratory chain.
Another important intermediary metabolic pathway within the mitochondria involves the fate of pyruvate. Glucose oxidation via glycolysis in the cytoplasmic compartment results in the formation of two moles of pyruvate from each mole of glucose. Without mitochondrial oxidation, pyruvate is anaerobically reduced to lactate; yielding only two of the total 38 moles of potentially available ATP if pyruvate were metabolized via the TCA cycle. Pyruvate can be translocated across the mitochondrial membrane and oxidized to acetyl-coenzyme A (CoA) by the pyruvate dehydrogenase complex (PDHC), which then enters the TCA cycle by combining with oxaloacetate to form citrate. The TCA cycle enzymes are located in the mitochondrial matrix and depend on transporters embedded in the otherwise impermeable inner mitochondrial membrane for the influx of substrates and efflux of generated products.
Fatty acid oxidation (FAO) is the predominant source of energy for cardiac and skeletal muscle at all times and becomes the major pathway for energy production during fasting in liver, cardiac, and skeletal muscle. With prolonged fasting, fatty acids are converted into ketone bodies in the liver and exported to extrahepatic tissues as an alternate fuel when the supply of glucose is limited, thus sparing glucose for use by the brain. FAO leads to the generation of reducing equivalents for the respiratory chain: FADH2 and NADH. Fatty acids must be modified and transported to the mitochondrial matrix by the carnitine shuttle. The carnitine shuttle involves the synthesis of acylcarnitines by carnitine palmitoyl transferase 1 (CPT1) in the outer mitochondrial membrane followed by transport across the inner mitochondrial membrane by carnitine-acylcarnitine translocase (CACT) and finally conversion from an acylcarnitine back to an acyl-CoA by carnitine palmitoyl transferase 2 (CPT2).
Now located in the mitochondrial matrix, the long chain fats can enter the β-oxidation cycle that catalyzes the repetitive cleavage of two-carbon fragments from the fatty-acid chain and the generation of acetyl-CoA. Acetyl-CoA either condenses with oxaloacetate to form citrate and enters the TCA cycle, or becomes available for ketogenesis during fasting. Additional mitochondrial matrix enzymes catalyze the synthesis of acetoacetate and its reduction to β-hydroxybutyrate.
Other metabolic pathways partially housed in the mitochondria include the urea cycle, bile acid synthesis, methylmalonic acid and ethanol metabolism, and fatty acid synthesis. In addition, the mitochondrion plays a key role in intracellular calcium homeostasis. For the purposes of this chapter, mitochondrial diseases that affect oxidative phosphorylation will be emphasized. These disorders are caused by primary defects or are secondary to other interrelated metabolic defects or drug or toxin interactions. With advances in molecular genetic testing, it has become apparent that many unexplained diseases of infancy and early childhood are caused by genetic disorders of mitochondrial function. In this chapter, disorders of liver, pancreatic, and intestinal dysfunction in their primary presentation either alone or as part of a more global picture of CNS, muscle, bone marrow or renal disease will be described, with emphasis on the clinical manifestations, diagnosis, and treatment. Other common manifestations of these disorders are diabetes, cardiomyopathy, deafness, and retinitis pigmentosa. Details of the pathophysiology and molecular biology of these disorders are contained in several excellent reviews [1].
Epidemiology of Mitochondrial Disorders
There have been multiple studies documenting the prevalence of mitochondrial diseases in different populations (Table 35.3) and several considerations impact the interpretation of these data. Children are more likely to be symptomatic due to mutations in nDNA-encoded genes whereas adults are more likely to have a genetic diagnosis resulting from a mutation in mtDNA [11]. Most nDNA-mediated mitochondrial diseases have a recessive mechanism of inheritance and therefore the prevalence of specific diseases can be dramatically higher within communities that have a founder mutation in a specific gene. One pertinent example of that phenomenon is that of MPV17-related disease in the Navajo population [12].
Table 35.3 Epidemiologic Studies of Mitochondrial Diseases
Country | Age Range | Years | Method of Ascertainment of Cases | Prevalence per 100,000 | Reference |
---|---|---|---|---|---|
Australia | 0–16 years | 1987–1996 | Referred for mitochondrial evaluation | 5 | 11 |
Finland | 0–18 years | 1990–1996 | Referred for encephalopathy or myopathy | 12 | 97 |
Sweden | 0–16 years | 1984–1998 | Comprehensive review of medical records | 4.8 | 100 |
Ireland | 0–18 years | 1995–2002 | Referred for mitochondrial evaluation | 11 | 96 |
Spain | 0–16 years | 1990–2004 | Referred for neurological symptoms | 8.7 | 98 |
Portugal | 0–10 years | 1997–2006 | Referred for mitochondrial evaluation | 5.4 | 99 |
England | Over 16 years | 1990–2014 | Referred for mitochondrial evaluation | 20 | 13 |
Mitochondrial disease epidemiology is complicated by the fact that many carriers of mtDNA mutations are asymptomatic or oligosymptomatic due to having relatively lower heteroplasmy for the mutation. In aggregate, known pathogenic mutations in mtDNA and nDNA mitochondrial genes are present in one in 4,300 adults and about half of those with a known mutation are symptomatic [13]. It is very important to note that some mtDNA mutations, in particular deletions, may not be detectable in blood because leukocytes without the mtDNA mutation outcompete those cells with the mutation and over time the entire lineage is populated by cells without the mtDNA mutation. This is an important consideration when genetic testing is performed on leukocytes only, and not the affected tissues.
Canonical Mitochondrial Syndromes
Mitochondrial diseases typically manifest with signs and symptoms affecting multiple organ systems. Manifestations that are often a part of mitochondrial clinical syndromes include: skeletal myopathy, cardiomyopathy, cardiac conduction defects, optic neuropathy, ophthalmoplegia, retinopathy, hearing loss, peripheral neuropathy as well as a wide variety of different signs and symptoms related to the central nervous system such as seizures, ataxia, dystonia and developmental delay. The canonical mitochondrial syndromes are listed in Table 35.4. In the current era, there is an increased emphasis on the genetic etiology of mitochondrial disease and a decreased emphasis on classification by clinical syndrome as there are many known phenocopies of mitochondrial disease due to non-mitochondrial genetic etiologies [14]. Additionally, there is typically a broad spectrum of phenotypes associated with each genetic etiology that often have only partial overlap with the associated canonical syndrome.
1. | Leigh syndrome |
2. | Pearson Marrow–Pancreas syndrome |
3. | Kearns–Sayre syndrome |
4. | Barth syndrome |
5. | MERRF: myoclonus, epilepsy, ragged red fibers |
6. | MELAS: myopathy, encephalopathy, lactic acidosis and stroke-like episodes |
7. | LHON: Leber hereditary optic neuropathy |
8. | NARP: Neuropathy, ataxia, and retinitis pigmentosa |
9. | MNGIE: Mitochondrial neuro-gastrointestinal encephalomyopathy |
10. | CPEO: Chronic progressive external ophthalmoplegia |
11. | Alpers–Huttenlocher Disease |
Classification of Mitochondrial Hepatopathies
In a variety of hepatic disorders, genetic or acquired defects in either specific biochemical pathways or more general dysfunction of mitochondria have been described. These conditions are frequently, but not universally, associated with morphologic changes of hepatic mitochondrial structure or number or with neuromuscular or other systemic involvement. These disorders (mitochondrial hepatopathies) can be classified by phenotype or genotype. In the Phenotypic Classification scheme (Table 35.5 and 35.10), the disorders are divided into primary hepatopathies, in which the mitochondrial defect is the primary cause of the liver disorder, and secondary hepatopathies, in which a secondary insult to mitochondria is caused by either a gene defect that affects non-mitochondrial proteins or by an acquired injury to mitochondria. The genotypic classification of mitochondrial hepatopathies is shown in Table 35.6. Many of the nuclear gene mutations affecting oxidative phosphorylation result in primarily neurological or neuromuscular disease. Of note, mutations in nuclear genes encoding proteins responsible for mtDNA maintenance and replication are more often associated with hepatopathy than other categories of genetic mitochondrial disease. This group of genes includes TYMP, DGUOK, POLG, and MPV17 among others. These phenotypically and genotypically heterogeneous disorders share a common mechanism of disturbed mitochondrial nucleoside pools or mtDNA stability [15]. The identification of mutations in additional nuclear genes impacting mitochondrial function is the focus of ongoing research efforts in the field of mitochondrial medicine that will certainly lead to many additional new genetic etiologies of mitochondrial hepatopathy in coming years.
Gene (Associated Syndrome) | Protein Function | Respiratory Chain Complex Deficiencies | Biochemical Findings | Hepatic Features | Notable Extrahepatic Clinical Features |
---|---|---|---|---|---|
Multigene mtDNA deletion (Pearson) | − | I, III, IV | Lactic acidosis | Steatosis, fibrosis, mitochondrial proliferation | Anemia, exocrine pancreatic insufficiency, arrhythmia, renal Fanconi, progressive neurological disease |
POLG (Alpers-Huttenlocher) | Mitochondrial DNA polymerase | I, III, IV | Often normal | Acute liver failure with valproate exposure | Intractable and progressive epilepsy, characteristic brain MRI findings |
MPV17 (Navajo Neurohepatopathy) | Mitochondrial inner membrane nucleotide transporter | I, III, IV | Often normal | Steatosis, fibrosis, liver failure | Peripheral neuropathy, developmental delay, hypotonia |
DGUOK | Deoxynucleotide metabolism | I, III, IV | Lactic acidosis, hypoglycemia | Steatosis, fibrosis, liver failure, noncirrhotic portal hypertension | Hypotonia, nystagmus, developmental delay in more severely affected patients |
TYMP (MNGIE) | Thymidine and deoxyuridine phosphorylase | I, III, IV | Elevated thymidine | Hepatopathy typically a late manifestation of disease | Gastrointestinal pseudoobstruction, peripheral neuropathy, ptosis, ophthalmoplegia, myopathy, characteristic brain MRI findings |
TWNK | Mitochondrial DNA helicase | I, III, IV | Often normal | Alpers-like syndrome, hepatopathy only in severe, early-onset disease | Progressive neurological disease |
SUCLG1 | Succinate Co-A ligase (TCA cycle enzyme) | I, III, IV | Elevated methylmalonic acid, lactic acidosis | Hepatopathy in about half of reported patients | Hypotonia, deafness, growth restriction, severe neurological disease |
TRMU | Mitochondrial tRNA modification | I, III, IV | Lactic acidosis, hypoglycemia, hyperammonemia | Liver failure in infancy that spontaneously resolves in some patients | Usually no extrahepatic manifestations |
SERAC1 (MEGDHEL) | Mitochondrial membrane lipid remodeling | Variable | Elevated 3-methylglutaconic acid, lactic acidosis | Neonatal liver dysfunction in half of patients | Deafness, dystonia, developmental regression, characteristic brain MRI findings |
QIL1 | Inner mitochondrial membrane organization | Variable | Elevated 3-methylglutaconic acid | Neonatal or infantile liver failure in most patients | Microcephaly, progressive neurological disease |
GFM1 | Mitochondrial translation elongation factor | I, III, IV | Lactic acidosis | Neonatal or infantile liver failure in some patients | Severe neurological disease, some with regression, epilepsy, characteristic brain MRI findings |
BCS1L (GRACILE) | Complex III assembly factor | III | Lactic acidosis | Cholestasis and liver iron accumulation | Growth failure, renal Fanconi syndrome, severe neurological disease |
Emphasis in this chapter will be placed on those mitochondrial diseases involving the electron transport proteins (respiratory chain) and mtDNA stability in the liver. These usually present either as neonatal liver failure (either a single life-threatening event or recurrent episodes), as a gradually progressive liver disease that may suddenly deteriorate in early childhood (frequently associated with neuromuscular symptoms), or as a chronic fibrosing liver disease leading to portal hypertension and its complications. In the secondary disorders, hepatic mitochondria undergo injury or their function is impaired secondary to another pathologic process. Among these disorders are diseases of uncertain etiology but clearly involving hepatic mitochondria (e.g., Reye syndrome); conditions caused by mitochondrial toxins, drugs or metals; and other conditions in which mitochondrial lipid peroxidation, microautophagy and/or abnormal electron transport have been observed and may be involved in the pathogenesis of liver dysfunction. The remainder of this chapter will focus on prototypic and important mitochondrial hepatopathies, particularly those involving electron transport and OXPHOS. The reader is referred to other chapters for detailed discussions about the deficiencies of other specific mitochondrial enzyme systems.
Primary Mitochondrial Hepatopathies
The liver and the gastrointestinal tract are frequently impacted in inherited defects of mitochondrial function. Reduced activity of respiratory chain complexes and OXPHOS has been associated with liver disease of varying severity and at different ages, however, neonatal and early childhood presentations predominate [2].
Respiratory chain defects in childhood can cause severe liver failure in the first weeks to months of life. This presentation is characterized by lactic acidosis, jaundice, conjugated hyperbilirubinemia, serum ALT values of two to 12 times normal, coagulopathy, hypoglycemia, and hyperammonemia [16]. Symptoms include lethargy, hypotonia, vomiting, poor feeding, seizures and failure to thrive. In other cases, an apparently healthy infant may have a viral infection or other inciting event that triggers hepatic and often neurologic deterioration. The key biochemical features in most of these infants are the markedly elevated plasma lactate concentration, elevated molar ratio of plasma lactate to pyruvate (>25 and frequently >30 mol/mol), and elevation of beta-hydroxybutyrate and the arterial ketone body ratio of beta-hydroxybutyrate to acetoacetate (>2.0 mol/mol). The lactic acidosis may worsen during the provision of intravenous glucose, a paradoxical finding that should increase suspicion of a respiratory chain defect. It is important to note that these biochemical features do increase the suspicion for mitochondrial hepatopathy but mitochondrial hepatopathies are certainly diagnosed in the absence of characteristic biochemical findings.
Primary mitochondrial disease is the etiology of acute liver failure for a significant minority of infants and was determined to be the etiological diagnosis for 5–20% of infants in several case series [17, 18, 19].
Liver histology shows predominantly microvesicular (sometimes combined with macrovesicular) steatosis, canalicular cholestasis with bile duct thrombi and bile ductular proliferation, and in some cases, hepatocellular cholestasis. Inflammation is usually absent or minimal. Periportal and centrilobular fibrosis may be extensive, with loss of broad bands of hepatocytes causing a micronodular cirrhotic appearance. Glycogen depletion is a near constant feature; and iron deposition, usually in hepatocytes, is often observed leading to confusion with neonatal hemochromatosis, particularly in DGOUK-related disease. Ultrastructural evidence of mitochondrial injury may be observed as swollen mitochondria, abnormal cristae, paracrystalline inclusions, and a fluffy matrix, although normal mitochondrial morphology may be present. Mitochondria density may be increased in each hepatocyte, presumably as a compensatory mechanism for impaired function of individual mitochondria.
These infants may progress rapidly from onset of symptoms to death from liver failure, progressive neurological disease or multisystemic illness in the first months of life despite supportive treatment. The majority of patients with infantile-onset mitochondrial hepatopathy have severe neurologic involvement in infancy with a weak cry, poor suck, hypotonia, recurrent apnea, myoclonic epilepsy, or a combination of these symptoms. The possibility of progressive neurological disease may preclude the option for liver transplantation. Other more variable aspects of the neonatal presentation include intrauterine growth retardation, hydrops fetalis, neonatal ascites, hypoalbuminemia, elevated alpha-fetoprotein, and renal tubular dysfunction.
Since the degree of expression of the underlying defect in different tissues is not uniform, a number of infants with mitochondrial hepatopathy have undergone successful liver transplantation in the absence of detectable extrahepatic manifestations. Others have developed progressive neurological symptoms and died following liver transplantation [20]. The primary factor determining expected prognosis after liver transplantation is the underlying genetic etiology, which underscores the importance of a thorough genetic evaluation prior to consideration of liver transplantation for infantile acute liver failure. For severely affected infants, nDNA mutations are much more likely to be the etiology of disease than mtDNA mutations.
mtDNA Deletions
Pearson marrow-pancreas syndrome was described in 1979 in four children with neonatal-onset severe macrocytic anemia, variable neutropenia and thrombocytopenia, vacuolization of erythroid and myeloid precursors and ringed-sideroblasts in the bone marrow [21]. Later in infancy or early childhood, diarrhea and fat malabsorption developed and the patients were found to have pancreatic insufficiency caused by extensive pancreatic fibrosis and acinar atrophy. Partial villous atrophy of the small intestine was noted in a number of patients. Marked hepatomegaly, hepatic steatosis, and cirrhosis has been associated with liver failure and death in some cases before the age of four years.
Single mtDNA deletions are the genetic etiology of Pearson syndrome as well as a broad spectrum of other manifestations that include Kearns Sayre syndrome and Chronic Progressive External Ophthalmoplegia (CPEO) [22]. In roughly half of patients, the deletion spans bases 8470–13446 of the mitochondrial genome and this is referred to as the “common deletion” [22, 23]. Larger and smaller deletions are also seen in affected patients. Studies conflict on the clinical implications of deletion size, but it is likely that both larger deletion size and higher heteroplasmy for the deletion convey poorer prognosis [24]. The vast majority (96%) of those with mtDNA deletion-mediated diseases have no family history of other affected individuals in their maternal lineage and mtDNA testing for relatives typically shows normal results [6].
Other clinical manifestations of Pearson syndrome include renal tubular disease (Fanconi syndrome), diabetes mellitus, cardiac conduction defects, adrenal insufficiency, tremor, ataxia, proximal muscle weakness, ptosis, external ophthalmoplegia and pigmentary retinopathy. Patients with Pearson syndrome that survive the first years of life typically do not require blood transfusions after the age of two years but non-hematological manifestations are progressive, including dementia in adolescence and young adulthood for many individuals.
When clinically suspected, Pearson syndrome (or Kearns Sayre) is diagnosed by analyzing for the characteristic mtDNA deletion. While this previously required special techniques (e.g., Southern Blot) to identify the deletion, next generation sequencing with deep read depth is an appropriately sensitive test with modern sequence analysis techniques. In those with hematological manifestations, the mtDNA deletion is detectable in blood. In those without hematological manifestations, the mtDNA deletion is often not detectable in blood due to selection against those hematopoietic precursor cells with mtDNA deletions and competitive advantage of those cells that lack the deletion. The competitive advantage of low heteroplasmy hematopoietic precursor cells is also the reason that the anemia associated with Pearson syndrome resolves after the first few years of life. Sequencing and deletion analysis of mtDNA can be performed in muscle or liver tissue, urine sediment, buccal swabs and other tissues in which the mtDNA deletion is more stable.
POLG
Alpers syndrome was initially described as a clinical entity causing progressive and intractable seizures in young children in 1931. In the 1970s, Huttenlocher recognized the association of that disease with hepatopathy. In the early 1990s, Alpers–Huttenlocher syndrome was better characterized with larger case series and in the early 2000s, POLG mutations were identified as the etiology of the disease [25].
The clinical course of Alpers syndrome typically entails an unremarkable infancy followed by onset of seizures with a peak incidence between the ages of two and four years, often in the context of a viral respiratory illness or other physiological stressor. Seizures progressively worsen with time and often become drug-refractory. Additional neurological findings include headaches, chorea, ataxia, hypotonia, loss of cognitive and motor skills and cortical vision loss. Specific features that may suggest POLG-related disease include partial seizures with secondary generalization and epilepsia partialis continua (EPC). MRI of the brain may demonstrate T2 hyperintensity of the deep grey nuclei or patchy cortical edema and restricted diffusion. Over time, progressive cerebral atrophy and gliosis develop.
Acute liver failure may be precipitated by use of valproate as an antiepileptic agent, though chronic progressive liver disease may also occur. Early in the course of the liver disease, liver pathology may only be notable for microvesicular steatosis, focal hepatocyte degeneration, and portal fibrosis (Figure 35.6). However, hepatic decompensation may ensue rapidly and lead to death in one to two months. At autopsy, the liver shows macrovesicular steatosis with accompanying micronodular cirrhosis, massive hepatocyte dropout (probably caused by apoptosis), parenchymal collapse, and bile ductular proliferation within broad bands of fibrous tissue (Figure 35.6B,C). On electron microscopy, there may be an increased number and density of normal appearing mitochondria in each hepatocyte or mitochondria may be swollen and pleomorphic with a less dense matrix and few cristae (Figure 35.7) [26]. Most children die by three years of age but some survive into their teenage years, often with significant neurological deficits. In those who show marked deterioration following the start of therapy for seizures, death has been attributed to hepatotoxicity caused by valproate, however, treatment with this drug may have only accelerated the natural history of the disease. Occasionally, this disorder is not recognized prior to liver transplantation in a child with acute liver failure; progressive neurologic deterioration commonly follows transplantation despite normal function of the liver allograft [20].
Figure 35.6 Liver histology obtained from a girl aged five years and seven months with Alpers-Huttenlocher disease. (A) Biopsy at the time of presentation with liver involvement, showing periportal inflammation (solid arrows), microvesicular steatosis and mild portal fibrosis. (B) Autopsy liver specimen obtained two months later demonstrating micronodular cirrhosis and extensive fibrosis, with prominent regenerative nodules and bile ductular proliferation. (C) Higher power view of edge of a regenerative nodule, demonstrating massive collapse of parenchyma with extensive portal fibrosis and bile ductular proliferation (solid arrows) and microvesicular steatosis in hepatocytes of regenerative nodules. (With permission from Narkewicz et al., J Pediatr 1990;119:260.)
Figure 35.7 Electron microscopy (original magnification 13,700×) of the liver from the child in Figure 35.6 with Alpers-Huttenlocher disease. Hepatocyte at onset of liver disease shows microvesicular steatosis (asterisk), normal peroxisomes (p), and morphologically normal mitochondria (m) but increased in number. Nu denotes nucleus. (With permission from Narkewicz et al., J Pediatr 1990;119:260.)
Definitive diagnosis is made via POLG sequencing, either as a single targeted gene when clinical suspicion is high or as part of a panel of genes for the indication of epilepsy or hepatopathy when the clinical scenario is less specific. Alpers syndrome is caused by biallelic mutations in POLG, though there are also specific disease-causing dominantly acting mutations that cause milder manifestations in a heterozygous state. The mutations that can cause POLG-related disease without a second mutation on the other allele are few in number and cluster in a few hotspots in the gene. Therefore, parents of a child diagnosed with Alpers syndrome due to biallelic POLG mutations are usually not at risk for any clinical manifestations. Dominant POLG disease manifests in adults with neurological and ophthalmological symptoms but not hepatopathy. There is a broad spectrum of neurological diseases (often including ataxia) that are less severe or later-onset than Alpers syndrome that also result from biallelic mutations in POLG. While the dominant and recessive mutations are distinct from one another, only rough genotype-phenotype correlations can be identified within the recessive mutations and individuals with identical genotypes may have significant differences in the severity of disease. The p.Ala467Thr mutation is a common cause of Alpers syndrome and has a carrier frequency of up to 1% in some European populations.
POLG encodes the enzyme polymerase gamma, which is responsible for replication and repair of mtDNA. In the absence of typical polymerase gamma function, there is depletion of mtDNA and accumulation of mtDNA mutations, which is the underlying pathophysiology of the mitochondrial dysfunction.
MPV17
MPV17-related disease was initially identified as Navajo neurohepatopathy (NNH), with an expanding understanding of the associated phenotype as the disease has been identified in other geographical areas and clinical contexts [12]. Common clinical features include liver failure, hepatomegaly, steatosis, developmental delay, hypotonia, peripheral neuropathy, abnormal brain MRI findings, failure to thrive, gastrointestinal dysmotility, lactic acidosis and hypoglycemia. Rarer manifestations of the disease include renal tubulopathy, retinopathy, keratopathy (due to lack of sensation), ataxia and dystonia.
Elevations of AST, ALT, alkaline phosphatase, and gamma glutamyl transpeptidase were present in all cases in the initial published NNH series. Liver histology demonstrated portal fibrosis or micronodular cirrhosis, macrovesicular and microvesicular steatosis, pseudoacinar formation, multinucleated giant cells, cholestasis, and periportal inflammation. Nonspecific mitochondrial changes, such as swollen mitochondria and ringed cristae, were seen in several patients. Blood lactate and pyruvate levels were normal in patients tested, and skin fibroblasts had normal respiration from one patient. The liver involvement in this disorder is progressive with liver failure developing within months to years in most patients. Three published individuals with MPV17-related disease have developed hepatocellular carcinoma.
Neurologic symptoms are progressive after liver transplant questioning the value of transplant in this disorder. While the vast majority of the >100 individuals who have been diagnosed and published had initial manifestations in the first months of life, it is now recognized that there are more mildly affected individuals with adult-onset disease and primarily neuromuscular manifestations [27].
The etiology of NNH is homozygosity for a founder mutation present in the Navajo population, p.R50Q. MPV17-related disease has now been identified in many different populations and most affected individuals have novel mutations including a variety of missense, nonsense, frameshift and splicing mutations as well as deletions [27].
MPV17 encodes a protein that localizes to the inner mitochondrial membrane and has four transmembrane domains. The protein likely forms a channel that allows deoxynucleotides to cross the inner mitochondrial membrane. The deficiency of mitochondrial deoxynucleotides results in mtDNA depletion and accumulation of mutations, which is the underlying basis of the mitochondrial dysfunction that causes the clinical manifestations in those with MPV17 mutations. The identification of this pathophysiology raises the possibility of nucleoside supplementation as a treatment strategy that could be developed for MPV17-related disease [28].
TYMP
TYMP encodes the enzyme thymidine phosphorylase, an enzyme responsible for maintaining balanced nucleoside pools for DNA replication. In the setting of thymidine phosphorylase deficiency due to biallelic mutations in TYMP, there is an excess of deoxythymidine and deoxyuridine, which are measurable metabolites in blood and are one of the characteristic biochemical signs of thymidine phosphorylase deficiency. The imbalanced nucleoside pools lead to mtDNA depletion as well as accumulation of point mutations and deletions in mtDNA over time. The progressive depletion and mutations of mtDNA lead to mitochondrial dysfunction and to the clinical manifestations of the disease [29].
In 1994, Hirano et al. labeled the syndrome now known to result from thymidine phosphorylase deficiency as mitochondrial neurogastrointestinal encephalomyopathy (MNGIE) [30]. MNGIE is characterized by myopathy with ragged-red fibers, peripheral sensorimotor neuropathy, progressive external ophthalmoplegia, ptosis, leukoencephalopathy, and chronic intestinal pseudo-obstruction. The disease onset ranges from five months to 43 years of age with the typical age of onset in the second or third decade of life. Non-specific gastrointestinal signs and symptoms of nausea, vomiting, abdominal pain, borborygmi, diarrhea, constipation and abdominal distention often lead to a diagnosis of intestinal dysmotility or “pseudo-obstruction.” Gastrointestinal symptoms typically have onset in childhood, and were the presenting complaint in the majority of patients. Sensory neuropathy, hearing loss, or ocular symptoms are also common presenting manifestations. Thin body habitus and short stature are common, presumably secondary to chronic malnutrition and malabsorption. Small bowel diverticulosis, presumably secondary to markedly delayed intestinal motility, appears in early adult years in many patients [31]. The chronic intestinal pseudo-obstruction is attributed to visceral smooth muscle myopathy as well as autonomic neuropathy, with corresponding pathology noted on post-mortem evaluations [32].
Progressive hepatopathy has been identified in a significant minority of individuals with MNGIE and is occasionally the presenting manifestation of disease [33]. TYMP-related disease has even been identified as the etiology of acute liver failure in infancy (author’s personal observation). Hematopoietic stem cell transplantation (HSCT) has been employed as a therapy for MNGIE. HSCT is effective in restoring normal balance of circulating nucleosides. Published accounts with up to eight years of follow-up after HSCT demonstrate some clinical improvement for patients. However, more than half of patients that underwent HSCT for MNGIE died, some from complications of HSCT, thus this treatment has largely been abandoned. Presence of liver disease at the time of HSCT resulted in poor prognosis [34]. This observation, as well as the recognition that expression of TYMP is high in liver tissue, have led to the use of liver transplantation as a therapeutic modality in a few individuals with MNGIE [35–37]. With many fewer patients and shorter follow-up for those patients that underwent liver transplantation as opposed to HSCT, it is currently not known which treatment conveys better prognosis. MNGIE is unusual among mitochondrial diseases in that the primary driver of pathology appears to be a circulating metabolite as opposed to the local effect of the enzyme in cells, which may make the disease amenable to treatment with administration of exogenous enzyme replacement therapy.
DGUOK
DGUOK encodes deoxyguanosine kinase, the enzyme responsible for mitochondrial purine nucleotide salvage. In the setting of deoxyguanosine kinase deficiency due to biallelic mutations in DGUOK, the resultant imbalanced nucleotide pools cause depletion of mtDNA and accumulation of mtDNA mutations, a similar pathogenesis to several of the other mitochondrial hepatopathies discussed in this chapter.
DGUOK-related disease typically presents in infancy with lactic acidosis, hypoglycemia, hypotonia and nystagmus. Hepatic manifestations include cholestasis, hepatomegaly and elevated aminotransferases evolving to coagulopathy and ascites. Many patients have elevated GGT, AFP, and ferritin. Liver biopsy histology may show cholestasis, steatosis, fibrosis, giant cell hepatitis or cirrhosis, and increased iron deposition. Mitochondrial proliferation may be demonstrated as well as abnormal mitochondrial ultrastructure [38]. For infants presenting with neurological and hepatic manifestations, the disease is usually fatal in the first few years of life [39]. Other patients may have isolated hepatic manifestations without significant neurological involvement. There is at least once case of hepatocellular carcinoma in an individual with DGUOK-related disease [40]. The most mildly affected patients with DGUOK-related disease and hepatic involvement are individuals, currently in adolescence, with isolated non-cirrhotic portal hypertension [41]
There are 14 published instances of liver transplantation for DGUOK-related disease with up to 22 years of follow up post-transplantation. In general, those patients that had overt neurological manifestations at the time of liver transplantation had poor outcomes. Many of those with absent or mild neurological manifestations have had a stable course with subtle or minimal neurological progression in the years after liver transplantation [42].
Nucleoside supplementation has demonstrated efficacy in an animal model of DGUOK-related disease and may be relevant as a therapeutic approach in the future for patients with DGUOK and other nucleoside imbalance diseases [43].
TWNK
TWNK was previously referred to as c10orf2. The gene encodes a protein that localizes to the mitochondrion and acts as a DNA helicase. The protein colocalizes with POLG and is a component of the mtDNA replication and maintenance system. Like defects in other components of the system, TWNK mutations result in mtDNA depletion and accumulation of mtDNA mutations with resultant mitochondrial dysfunction. Also like POLG, there are specific dominant mutations in TWNK that cause an adult onset disorder with symptoms limited to neurological and ocular findings.
Several infants with biallelic mutations in TWNK have been reported to have hepatopathy in addition to neurological disease. Some of these patients could be considered to have a clinical diagnosis of Alpers syndrome. Biochemical findings included elevated aminotransferases and AFP but no cholestasis or coagulopathy. mtDNA depletion was documented. All of the reported patients with this presentation of TWNK-related disease died within the first few years of life of progressive neurological manifestations [44, 45].
SUCLG1
SUCLG1 encodes a subunit of the enzyme succinate-CoA ligase, which catalyzes a step in the TCA cycle. The other subunit that comprises the heterodimeric protein is SUCLA2. Patients with biallelic mutations in either gene have an mtDNA depletion syndrome with the characteristic finding of elevated methylmalonic acid in blood and urine detected on organic acid analysis. SUCLG1 has higher expression in the liver and only SUCLG1-related disease patients have been reported to have hepatopathy and nearly half of reported patients had liver disease [46].
Patients generally have significant neurological disease, hypotonia, deafness, lactic acidosis and growth restriction. MRI of the brain commonly shows abnormal signal of the basal ganglia. Patients with hepatopathy have elevated aminotransferases, coagulopathy, hepatomegaly and steatosis on biopsy [47].
TRMU
TRMU encodes an enzyme that modifies mitochondrial tRNAs, specifically by 2-thiolation of uridine at the wobble position of the tRNAs for lysine, glutamine and glutamate. In the absence of TRMU activity, mitochondrial translation is impaired.
Patients with biallelic mutations in TRMU have been identified due to acute fulminant liver failure in the first months of life. Patients have had coagulopathy, cholestasis and elevated AFP. All have had marked lactic acidosis. Liver biopsies have had variable findings including cholestasis with bile duct proliferation, macrovesicular steatosis, fibrosis and nodular cirrhosis. Mitochondrial respiratory chain studies showed the expected pattern in the setting of an mtDNA translational disorder with decreases in the activity of complexes I, III and IV [48, 49]. One patient was reported to have conspicuous copper deposition in periportal hepatocytes [50]. Unlike other mitochondrial hepatopathies, many of the patients with TRMU-related disease have had spontaneous resolution of liver failure with subsequent good health for up to 14 years. A significant proportion of patients died due to liver failure in infancy.
A recent report documented a sibship in which the older sibling died in the setting of liver failure due to biallelic TRMU mutations and a younger sibling was also found to have biallelic mutations in utero. The younger sibling was treated from birth with cysteine, N-acetylcysteine, selenium, vitamin C and vitamin E supplementation and survived the first year of life with transient liver failure but did not undergo transplantation [51].
SERAC1
SERAC1 encodes a protein that mediates the interaction between the endoplasmic reticulum and the mitochondrial outer membrane and catalyzes the remodeling of phosphatidylglycerol. Like several other disorders that specifically impact the mitochondrial membrane, SERAC1-associated disease results in elevation of the metabolite 3-methylglutaconic acid in blood and urine, which can be an important diagnostic clue. SERAC1-associated disease has been referred to as MEGDHEL, which stands for 3-methylglutaconic aciduria type IV, sensorineural deafness, hepatopathy, encephalopathy and Leigh-like syndrome. A published series reporting 67 cases showed that nearly half of patients had neonatal liver dysfunction and a quarter had liver failure. The majority of patients also had hypotonia, developmental regression, severe intellectual disability, dystonia, hearing loss, lactic acidosis and almost all had abnormal signal in the basal ganglia on MRI of the brain [52].
Most did not have hepatopathy outside of the neonatal period. Biochemical findings included mildly elevated aminotransferases, elevated GGT, elevated AFP, coagulopathy and hyperammonemia in the setting of lactic acidosis and 3-methylglutaconic aciduria (detectable on urine organic acid analysis). Biopsy findings included steatosis, bridging fibrosis and mitochondrial ultrastructural abnormalities [53].
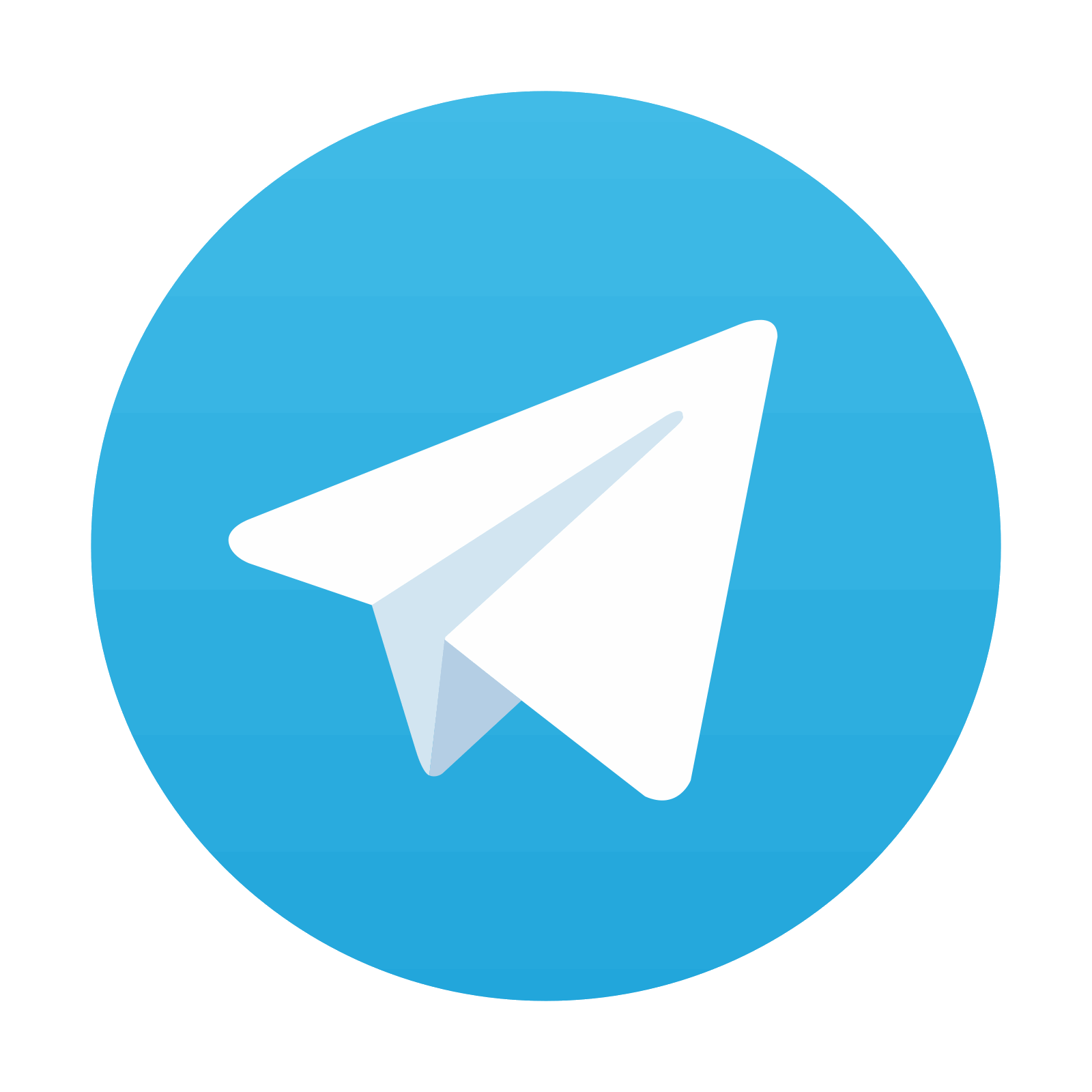
Stay updated, free articles. Join our Telegram channel
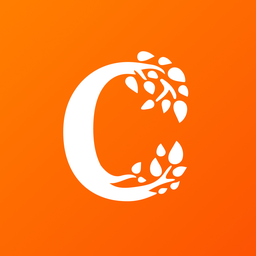
Full access? Get Clinical Tree
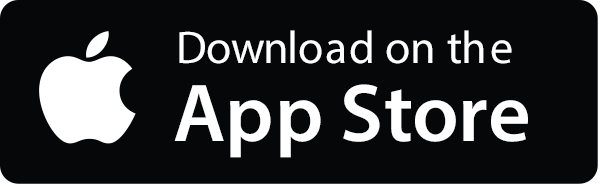
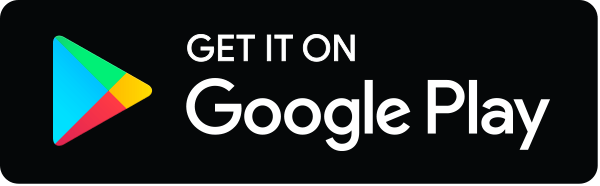
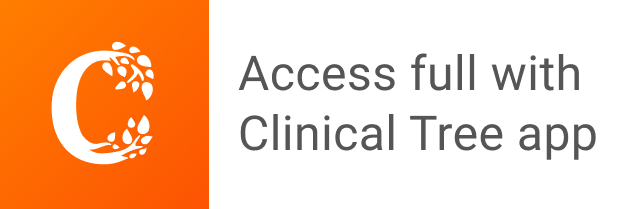