Abstract
The importance of bile acid synthesis and metabolism to normal physiology and their role in pathophysiological states is well recognized. For such small and relatively simple molecules, bile acids have amazingly diverse properties and functions. Bile acid biosynthesis represents one of the major pathways for regulating cholesterol homeostasis – each day approximately 0.5 g of cholesterol is metabolized to bile acids [1]. These molecules are essential for providing the major driving force for the promotion and secretion of bile and therefore are key elements in the development and maintenance of an efficient enterohepatic circulation. Bile acids are essential for facilitating the solubilization and absorption of fats and fat-soluble vitamins from the small bowel, although in the large bowel these molecules if in excess are potentially harmful in that they are carthartic, membrane damaging, and promoters of colonic disease. More recently, bile acids have become of interest because of their hormone-like actions of relevance to obesity, glucose and insulin regulation where they are now regarded as important molecules that signal through orphan receptors to regulate metabolism. With regard to bile acid biosynthesis, comprehensive reviews on the topic have been published previously [1, 2], and in the fourth edition of this textbook a detailed description of the pathways for bile acid synthesis was described.
Introduction
The importance of bile acid synthesis and metabolism to normal physiology and their role in pathophysiological states is well recognized. For such small and relatively simple molecules, bile acids have amazingly diverse properties and functions. Bile acid biosynthesis represents one of the major pathways for regulating cholesterol homeostasis – each day approximately 0.5 g of cholesterol is metabolized to bile acids [1]. These molecules are essential for providing the major driving force for the promotion and secretion of bile and therefore are key elements in the development and maintenance of an efficient enterohepatic circulation. Bile acids are essential for facilitating the solubilization and absorption of fats and fat-soluble vitamins from the small bowel, although in the large bowel these molecules if in excess are potentially harmful in that they are carthartic, membrane damaging, and promoters of colonic disease. More recently, bile acids have become of interest because of their hormone-like actions of relevance to obesity, glucose and insulin regulation where they are now regarded as important molecules that signal through orphan receptors to regulate metabolism. With regard to bile acid biosynthesis, comprehensive reviews on the topic have been published previously [1, 2], and in the fourth edition of this textbook a detailed description of the pathways for bile acid synthesis was described. This chapter will therefore provide an overview of the pathways of bile acid synthesis and metabolism, and will focus on specific inborn errors in bile acid synthesis and metabolism that are now regarded as a cause of progressive cholestatic liver disease and of syndromes of fat-soluble vitamin malabsorption, or neurological disease.
Pathways of Bile Acid Synthesis from Cholesterol
Structurally, bile acids possess a cyclopentanoperhydrophenanthrene (ABCD-ring) nucleus and therefore belong to the chemical class of steroids [3]. They differ from steroid hormones and neutral sterols, such as cholesterol, in having a five-carbon atom side-chain with a terminal carboxylic acid (Figure 33.1). Bile acids are synthesized in the liver from cholesterol by a complex series of chemical reactions catalyzed by 17 different hepatic enzymes located in the endoplasmic reticulum, mitochondria, cytoplasm and peroxisomes [2, 3] and consequently there is considerable trafficking of intermediates between these subcellular compartments. Several of the enzymes are also found in extrahepatic tissues. The enzymes involved in bile acid biosynthesis have all been isolated and well characterized in pioneering work performed in the late 1960s–1970s (Table 33.1), and more recently the role that each enzyme plays in the regulation of bile acid synthesis has been elucidated from studies of gene knock-out animal models and humans with genetic defects in bile acid synthesis [2]. cDNAs have now been described for these enzymes, including the rate-limiting enzyme in the bile acid biosynthetic pathway, cholesterol 7α-hydroxylase and these have provided important tools to examine the regulation of bile acid synthesis and to confirm genetic defects in bile acid synthesis.
Figure 33.1 The 5β-cholanoic acid nucleus that is the basic structure of C24-bile acids of mammalian species. Indicated are the ABCD rings, the numbering system for the carbon atoms, and the metabolic sites of substitution of functional groups occurring under normal and pathophysiologic conditions. Unsaturation can also occur in the nucleus (mainly at positions Δ4, Δ5), and in the side chain. The smaller font size signifies the relative quantitative importance of the conjugation reactions.
Table 33.1 Genetic Defects in Bile Acid Synthesis Causing Liver Disease, Fat-Soluble Vitamin Malabsorption, or Neurological Disease Listing the Specific Enzyme Deficiencies, Gene and Chromosome Location and Reference to First Description in Humans
Bile Acid Disorder | Gene and Location | Genetic Defect First Described in Humans (reference) |
---|---|---|
Steroid ring disorders: | ||
cholesterol 7α-hydroxylase (CYP7A1) | CYP71A (8q11-q12) | Pullinger et al., 2002 [37] |
oxysterol 7α-hydroxylase (CYP7B1) | CYP7B1 (8q21.3) | Setchell et al., 1998 [7] |
12α-hydroxylase (CYP8B1)* | CYP8B (3p21.3-p22) | Iser et al., 1977 [41] |
3β-hydroxy-Δ5-C27-steroid oxidoreductase | HSD3B7 (16p11.2-p12) | Clayton et al., 1987 [11] |
Δ4-3-oxosteroid 5β-reductase deficiency (AKR1D1) | AKR1C4 (7q32-q33) | Setchell et al., 1988 [20] |
Side-chain modifications: | ||
sterol 27-hydroxylase (CYP27A1) | CYP27A1 (2p23.3-p24.1) | Salen et al., 1974 [61] |
2-methylacyl-CoA racemase (AMACR) | AMACR (5p13.2-q11.1) | Setchell et al., 2003 [26] |
sterol 25-hydroxylase | CH25 H (10q23.31) | Clayton et al., 1995 [71] |
bile acid-CoA ligase (BACL) | BACL (19q13.43) | Chong et al., 2011 [32] |
bile acid-CoA:N-acyl amino acid transferase (BAAT) | BAAT (9q22.3) | Setchell et al., 1994 [30] |
Secondary bile acid synthetic defects: | ||
peroxisomal biogenesis disorders (PEX defects) | multiple PEX genes | Steinberg et al., 2009 (see Chapter xx) |
Δ7-dehydrocholesterol reductase (Smith–Lemli–Opitz) | DHCR7 (11q13.4) | Smith et al., 1964 [80] |
Conjugated (glycine and taurine) cholic and chenodeoxycholic acids are the two primary bile acids synthesized in humans, but there is considerable variability in the qualitative pattern of bile acids among animal species. Although there is a tendency to illustrate the reactions in the bile acid synthetic pathway to occur in a linear fashion (Figure 33.2), moving from initiation of changes to the steroid nucleus through modification of the side-chain, in reality there is considerable substrate promiscuity for the enzymes catalyzing the various reactions, which consequently results in a vast number of different bile acids and intermediates being synthesized. This is especially evident during early development, a period of physiological cholestasis, and in pathological conditions that interfere with the integrity of the enterohepatic circulation [4]. Furthermore, intestinal bacterial modifications, resulting in the formation of “secondary” bile acids, adds a further level of complexity to the bile acid composition of biological fluids [5].
Figure 33.2 Metabolic pathways for the formation of the primary bile acids of cholic and chenodeoxycholic acids. The different enzymes catalyzing the reactions are indicated in italics. The broken arrows indicate multiple steps in the conversion. Shown by the shaded areas are the classical (neutral) pathway, the acidic pathway, and several alternative pathways for bile acid synthesis.
There are two main pathways leading to primary bile acid synthesis. These are termed the “neutral” and “acidic” pathways, the former being the classical one that is initiated by the rate-limiting cytochrome P450 liver-specific enzyme, cholesterol 7α-hydroxylase (CYP7A1) leading to cholic acid synthesis, and the latter being initiated by the action of cholesterol 27-hydroxylase (CYP27A1) on the side-chain to yield chenodeoxycholic acid [1, 2]. This acidic pathway leads to the formation of 3β-hydroxy-5-cholenoic and lithocholic acids, as intermediates to chenodeoxycholic acid and these markedly hepatotoxic monohydroxy-bile acids are increased in early life and in cholestatic liver diseases. It is now accepted that the “acidic” pathway [6] contributes significantly to overall total bile acid synthesis, and especially to chenodeoxycholic acid synthesis [1, 6]. Primary bile acid synthesis is not exclusively dependent on cholesterol 7α-hydroxylase, and under certain conditions, alternative pathways are induced. The CYP7B1 gene is localized to chromosome 8q21.3 and in close proximity to the CYP7A1 gene [2]. Extremely high levels of 27-hydroxy-cholesterol and hepatotoxic monohydroxy bile acids, 3β-hydroxy-5-cholenoic and 3β-hydroxy-5-cholestenoic acids were first reported in an infant with a genetic defect in oxysterol 7α-hydroxylase [7]. A mutation in the CYP7B1 gene will cause a phenotype of progressive and fatal liver disease [7–10] and indicates the quantitative importance of the “acidic” pathway in early human life.
Following the synthesis of 7α-hydroxycholesterol, modifications to the steroid nucleus take place that result in oxidoreduction and C-12 hydroxylation, consequently preparing the sterol intermediates for direction into either the cholic acid (3α,7α,12α-trihydroxy-5β-cholan-24-oic), or chenodeoxycholic acid (3α,7α-dihydroxy-5β-cholan-24-oic) pathways. According to convention, 7α-hydroxycholesterol is converted to 7α-hydroxy-4-cholesten-3-one, a reaction catalyzed by a microsomal NAD-dependent 3β-hydroxy-Δ5-C27-steroid oxidoreductase enzyme (HSD3B7), formerly referred to as a 3β-hydroxy-Δ5-C27-steroid dehydrogenase/isomerase. This enzyme shows substrate specificity toward 7α-hydroxylated sterols and bile acids possessing a 3β-hydroxy-Δ5 nucleus and is inactive on 7α-hydroxylated analogues [2]. Mutations in the gene encoding this enzyme are associated with progressive intrahepatic cholestasis [11, 12] and this can cause late-onset chronic cholestasis.
12α-Hydroxylation of the product of the above reaction will direct the Δ4-3-oxo intermediate into the cholic acid pathway. This reaction is catalyzed by a liver-specific microsomal cytochrome P450 12α-hydroxylase (CYP8B1). The activity of 12α-hydroxylase enzyme determines the relative proportion and synthetic rate of cholic relative to chenodeoxycholic acids [13, 14] and appears in man to be up-regulated by interruption of the enterohepatic circulation [15]. It is possible that in utero there may be reduced activity of this enzyme because fetal bile has a predominance of chenodeoxycholic acid [4]. By contrast, the ratio of cholic acid to chenodeoxycholic acid is very high in neonatal bile compared with adult bile [4]. The neonatal period of life is associated with a phase of physiological cholestasis, which may lead to an up-regulation in 12α-hydroxylase activity with a consequent increase in cholic acid synthesis.
7α-hydroxy-4-cholesten-3-one and 7α,12α-dihydroxy-4-cholesten-3-one both undergo reduction with formation of a 3-oxo-5β(H)-structure and this generates the basic trans-configuration of the A/B-rings of the steroid nucleus that is common to the majority of bile acids of most mammalian species. Allo(5α-H)− bile acids, are often major bile acid species of lower vertebrates but are only found in small proportions in biological fluids from humans. These are formed by an analogous reaction but catalyzed by a hepatic 5α-reductase. The Km of 5β-reductase is high and consequently under normal conditions 5β-reduction is favored. Δ4-3-oxosteroid 5β-reductase, a cytosolic aldo-keto reductase (AKR1D1), is a protein of approximately 38 kDa comprising 326 amino acids that differs significantly in structure from the 5α-reductase and has broad substrate specificity [16]. Although under normal conditions this enzyme does not appear to be of regulatory importance for bile acid synthesis, its activity parallels the activity of cholesterol 7α-hydroxylase and therefore measurement of the plasma concentration of 7α-hydroxy-4-cholesten-3-one (C4, or sterol-C4) can be used as an indirect assessment of hepatic cholesterol 7α-hydroxylase activity [17]. The finding of elevated proportions 3-oxo-Δ4 bile acids in biological fluids during early life and in advanced cholestatic liver disease [18, 19] suggests that under pathological conditions, rather than cholesterol 7α-hydroxylase, this enzyme becomes rate-limiting for bile acid synthesis. Mutations in the gene encoding AKR1D1 are clinically manifest as progressive intrahepatic cholestasis and biochemically by the production of large amounts of C24-3-oxo-Δ4-bile acids and allo-bile acids [20–23].
The enzyme catalyzing the conversion of the 3-oxo-5β(H)-sterols to the corresponding 3α-hydroxy-5β(H) intermediates is a soluble 3α-hydroxysteroid dehydrogenase (AKR1C4). This enzyme catalyzes the oxido-reduction of a number of substrates, and several cDNA clones with sequence similarity to other aldo-keto reductases have been described suggesting the existence of multiple isozymes. This final step in modification of the steroid nucleus results in the formation of the key intermediates, 5β-cholestane-3α,7α-diol and 5β-cholestane-3α,7α,12α-triol (bile alcohols) which then undergo a sequence of reactions leading to side-chain oxidation and consequent shortening by three carbon atoms (Figure 33.2).
The initial step in side-chain oxidation of the bile alcohols involves hydroxylation of the C-27 carbon atom, a reaction that is catalyzed by a mitochondrial cytochrome P450 27-hydroxylase (CYP27A1), and leads to the formation of 5β-cholestane-3α,7α,12α,27-tetrol. It is now known that CYP27A1 is also responsible for the complete oxidation reaction, that yields directly 3α,7α,12α-trihydroxy-5β-cholestanoic acid. 5β-Cholestane-3α,7α,12α,27-tetrol may also undergo oxidation by the combined actions of soluble or mitochondrial alcohol and aldehyde dehydrogenases, but the relative importance of these reactions compared with the complete 27-hydroxylase-catalyzed reaction is not known. This enzyme catalyzes the formation of 27-hydroxycholesterol, the first step in the “acidic” pathway [1, 2]. Mutations in this gene cause the rare lipid storage disease of cerebrotendinous xanthomatosis (CTX) and have only a modest effect on bile acid synthesis, partly because alternative pathways for bile acid synthesis support the production of compensatory levels of cholic acid [24]. Based on studies of patients with CTX, it was proposed that the C-25 hydroxylation pathway may be a major pathway for cholic acid synthesis but evidence shows that this pathway accounts for less than 5% of the total bile acids synthesized in healthy adults. Hydroxylation of cholesterol also occurs at the C-24 and C-25 positions in addition to the aforementioned 27-hydroxylation to yield oxysterols, which are potent repressors of cholesterol synthesis [24].
Following 27-hydroxylation, oxidation to the respective 25 R-enantiomeric long-chain cholestanoic (C27) acids, the next step is formation of CoA esters by the action of a bile acid-CoA ligase (synthetase), of which two forms have been identified; one that activates newly synthesized C27 cholestanoic acids, the other activates cholanoic acids formed as secondary bile acids returning to the liver for reconjugation. The products of this reaction are the formation of CoA esters of (25R)-3α, 7α-dihydroxy-cholestanoic and (25R)-3α, 7α,12α-trihydroxy-cholestanoic acids [1]. The (25R)- diastereoisomers must be racemized to the (25S)- forms in order to penetrate the peroxisome for subsequent oxidation. This reaction is catalyzed by a 2-methylacyl Coenzyme A racemase enzyme that is also active on branched-chain fatty acids such as phytanic acids. A mutation in the gene encoding this enzyme leads to the accumulation of (25R)-cholestanoic acids and phytanic acids and presents with neurological and liver disease [25, 26]. The final stage in modification of the side-chain involves the β-oxidation of the cholestanoic acids-CoA derivatives, which occurs by multiple-step reactions within peroxisomes leading to cleavage of propionic acid and the formation of the C24 bile acid CoA end-products [1]. With the exception of the acyl-CoA oxidase, defects in enzymes responsible for the β-oxidation of very long chain fatty acids (VLCFA) exhibit abnormalities in primary bile acid biosynthesis.
Some mention of allo (5α-reduced)-bile acids is warranted even though under physiological conditions these account for a relatively small proportion of the total bile acids in biological fluids of humans. These are major bile acid species of many lower vertebrates. In humans, 5α-reduced bile acids are usually formed by the action of intestinal microflora on 3-oxo-5β-bile acids during their enterohepatic circulation and consequently are found in significant amounts in feces [5]. In rodents, these can be formed in the liver from 5α-cholestanol. This pathway begins with 7α-hydroxylation of 5α-cholestanol and the product is then converted to 5α-cholestane-3α,7α-diol via the intermediate 7α-hydroxy-5α-cholestan-3-one. Hepatic 12α-hydroxylation of 5α-sterols is very efficient in the rat and readily leads to the formation of allocholic acid. A further pathway for allo-bile acid formation involves the hepatic 5α-reduction of 7α-hydroxy- and 7α,12α-dihydroxy-3-oxo-4-cholen-24-oic acids, a reaction catalyzed by a Δ4-3-oxosteroid 5α-reductase, and the finding of large proportions of allo-bile acids in infants with severe cholestatic liver disease due to a AKR1D1 deficiency indicates these to be primary bile acids of hepatic origin in man [20]. Both 5α-reductase isozymes are expressed in the liver beginning from birth [27].
A striking feature of bile acid synthesis and metabolism during early life is the relatively large proportion of polyhydroxylated, unsaturated and oxo-bile acids that are synthesized and these are not found in the bile of healthy adults. Although frequently referred to as “atypical,” this is a misnomer since they are in fact very typical of the developmental phase of hepatic metabolism [4, 18, 19]. Interestingly, the qualitative and quantitative bile acid composition of biological fluids in early life closely resembles that of adults with severe cholestatic liver disease, suggesting that in the diseased liver there is a reversion to more primitive pathways of synthesis and metabolism [4]. The most notable distinction in ontogeny is the prevalence of cytochrome P450 hydroxylation pathways that rapidly decline in importance over the first year of life. The most important hydroxylation reactions are 1β-, 4β- and 6α-hydroxylation that are of hepatic origin [28]. The concentrations of several of the metabolites, in particular hyocholic (3α,6α,7α-trihydroxy-5β-cholanoic) and 3α,4β,7α-trihydroxy-5β-cholanoic acids, exceed that of cholic acid in fetal bile [4, 28]. The role of these hydroxylation pathways is uncertain, but additional hydroxylation of the bile acid nucleus increases the polarity of the bile acid so facilitating renal clearance, while also decreasing its membrane-damaging potential. In early life, and particularly in the fetus, an immaturity in canalicular and ileal bile acid transport processes leads to a sluggish enterohepatic circulation and hydroxylation serves as a hepatoprotective mechanism.
Bile Acid Conjugation
Irrespective of the pathway by which cholic and chenodeoxycholic acids are synthesized, the CoA thioesters of the primary bile acids are ultimately conjugated to the amino acids glycine and taurine [1]. This two-step reaction is catalyzed by a rate-limiting bile acid-CoA ligase enzyme followed by a bile acid CoA:amino acid N-acyltransferase (EC 2.3.1.65) [29]. The genes encoding both enzymes, SLC27A5 and BAAT have been cloned [2]. The highest activity of the conjugating enzymes is found in peroxisomes.
Genetic defects in the bile acid amidation have been associated with fat-soluble vitamin malabsorption states with variable degrees of liver disease [30–32]. The bile acid CoA:amino acid N-acyltransferase enzyme utilizes glycine, taurine and interestingly, β-fluoroalanine, but not alanine, as substrates [29]. In man, the final products of this complex multi-step pathway are the two conjugated primary bile acids, cholic and chenodeoxycholic acids, and these are then secreted across the canaliculus and stored in gallbladder bile. In humans, glycine conjugation predominates with a ratio of glycine to taurine conjugates of 3.1:1 for normal adults. In early human life >80% of the bile acids in bile are taurine conjugated due to the abundance of hepatic stores of taurine [4].
Although the principal bile acids of man are amidated, other conjugates occur naturally (Figure 33.1) and these include sulfates, glucuronide ethers and esters, glucosides, N-acetylglucosaminides and conjugates of some drugs [1]. These conjugates account for a relatively large proportion of the total urinary bile acids. Conjugation significantly alters the physicochemical characteristics of the bile acid, lowering the pH, and serving to maintain and retain a high intraluminal concentration to facilitate lipid absorption [30]. It increases the polarity of the molecule to facilitate renal excretion, and minimize the membrane damaging potential of the more hydrophobic unconjugated species. Under physiological conditions, these alternative conjugation pathways are quantitatively less important. However, in cholestatic liver disease, or when the liver is subjected to a bile acid load, as with ursodeoxycholic acid (UDCA) therapy, the concentrations of these conjugates in biological fluids may dramatically change.
Sulfation of bile acids, most commonly at the C-3 position, but also at C-7, is catalyzed by a bile acid sulfotransferase. A relatively small proportion of bile acid sulfates in fetal bile suggest that hepatic sulfation is negligible in the fetus and neonate [4]. Indeed, it is most probable that urinary bile acid sulfates originate mainly by renal sulfation; 60–80% of urinary bile acids are sulfated and their excretion increases in cholestasis. Only traces of bile acid sulfates are found in bile despite efficient canalicular transport when sulfated bile acids are perfused.
A number of glucuronosyltransferases catalyze the formation of glucuronide ethers and esters. The enzymes show substrate selectivity in that bile acids possessing a 6α-hydroxyl group are preferentially conjugated at the C-6 position forming 6-O-ether glucuronides, while short chain bile acids form mainly glucuronides [1].
Glucosides and N-acetylglucosaminides of non-amidated and amidated bile acids have been identified in normal human urine with quantitative excretion comparable to that of glucuronide conjugates. A microsomal glucosyltransferase has been isolated and purified from human liver but is also present in extrahepatic tissues. The enzyme responsible for N-acetylglucosaminide formation exhibits remarkable substrate specificity in that it preferentially catalyzes the conjugation of bile acids having a 7β-hydroxyl group and consequently these conjugates account for >20% of the urinary metabolites of patients administered UDCA [1].
Formation of Secondary Bile Acids
Intestinal microflora play an important role in bile acid synthesis and metabolism. Bacterial enzymes metabolize primary bile acids, altering significantly their physicochemical characteristics and influencing their physiological actions during enterohepatic recycling. The result is the formation of a spectrum of secondary bile acids that are excreted in feces [5]. Deconjugation of conjugated bile acids followed by 7α-dehydroxylation are quantitatively the most important reactions, but bacterial oxido-reduction and epimerization at various positions of the bile acid nucleus also take place along the intestinal tract. High proportions of secondary bile acids in the proximal jejunum, mid-small bowel, ileum and cecum can be found when there is small bowel bacterial overgrowth [33, 34]. The enzymes that catalyze these reactions are found in a variety of organisms, such as Bacteroides, Clostridia, Bifidobacteria, Escherichia coli, and some of these reactions occur in the proximal small intestine. Deconjugation precedes 7α-dehydroxylation, and the bacterial peptidases responsible for this reaction exhibit remarkable substrate specificity in that the length of the side-chain is a crucial factor influencing this reaction. 7α-Dehydroxylation of cholic and chenodeoxycholic acids, a reaction that proceeds via a 3-oxo-Δ4-intermediate, results in the formation of deoxycholic and lithocholic acids respectively, and these secondary bile acids make up the largest proportion of total fecal bile acids [5]. Lithocholic and deoxycholic acids are relatively insoluble and consequently poorly absorbed. Serum concentrations of deoxycholic acid therefore provide a useful means of assessing the extent of impairment of the enterohepatic circulation in cholestatic liver diseases [33].
Regulation of Bile Acid Synthesis
The major factor influencing bile acid synthesis is negative feedback by bile acids returning to the liver via the portal vein during their enterohepatic recycling. There are marked differences in the ability of different bile acids to regulate cholesterol 7α-hydroxylase which occurs by signaling through the nuclear receptor farnesoid X receptor (FXR; NHR1H4). For example, while the primary bile acids cholic and chenodeoxycholic acids downregulate synthesis, bile acids possessing a 7β-hydroxy group, such as UDCA, do not, and the latter may actually increase synthesis rate. This has relevance to the treatment of inborn errors of bile acid synthesis. Interruption of the enterohepatic circulation, by biliary diversion or the feeding of anion exchange resins that bind bile acids in the intestinal lumen, results in an upregulation of cholesterol 7α-hydroxylase activity. In general, factors that influence cholesterol 7α-hydroxylase activity cause concomitant changes in the activity of HMG-CoA reductase, the rate-limiting enzyme for cholesterol synthesis and this serves to regulate cholesterol synthesis and maintain a constant cholesterol pool-size. Interestingly, cholesterol 7α-hydroxylase exhibits a diurnal rhythm that is synchronous with the activity of HMG-CoA reductase and is reflected by diurnal changes in bile acid synthesis rates. A significant nocturnal rise in bile acid synthesis takes place that may be regulated by glucocorticoids.
The mechanism involved in regulating cholesterol 7α-hydroxylase activity and therefore bile acid synthesis rate is complex. Bile acids and oxysterols signal through a number of nuclear receptors, including the key receptor, FXR, and its transcription factor, the short heterodimer partner (SHP, NR0B2) to repress CYP7A1 [2]. Bile acids have been shown to enter the nucleus of the hepatocyte and nuclear concentrations increase with bile acid feeding. Fibroblast growth factor-19 (FGF19) and its receptor FGFR4, and the G protein-coupled receptor TGR5 are also key elements of bile acid signaling and much has been learned about the regulation of cholesterol and bile acid synthesis from gene knock-out models of all these receptors and proteins. Modified, bile acid molecules are being developed that target these receptors in order to devise ways of regulating cholesterol homeostasis and glucose metabolism. One such example being the recently FDA-approved obeticholic acid, a potent FXR agonist. In addition to influencing the transcriptional regulation of cholesterol 7α-hydroxylase in the liver these receptors also induce transcription of IBABP (ASBT), the ileal bile acid binding protein that is involved in the ileal uptake and conservation of the bile acid pool. A number of drugs are now in clinical trials that bind to ASBT in order to interrupt the enterohepatic cycling of bile acids.
Defects in Bile Acid Synthesis Causing Metabolic Liver Disease and Syndromes of Fat-Soluble Vitamin Malabsorption
Defects in bile acid synthesis have profound effects on hepatic and gastrointestinal function and on cholesterol homeostasis, especially when the cause is a genetic mutation encoding enzymes responsible for primary bile acid synthesis [35]. Such defects lead to an overproduction of hepatotoxic atypical bile acids that are synthesized from intermediates accumulating in the pathway proximal to the inactive/missing enzyme, and a progressive cholestasis exacerbated by the lack of primary bile acids that are critical for promoting bile-flow. Marked alterations in urinary, serum, and biliary bile acids are found in all infants and children with liver disease and it can be difficult to determine whether such changes are primary or secondary to the liver dysfunction. The first bile acid synthetic defect causing liver disease was discovered as a result of applying the liquid secondary ionization mass spectrometry (LSIMS) technique of fast atom bombardment ionization mass spectrometry (FAB-MS) [11]. While FAB-MS is still a definitive technique for diagnosing bile acid synthetic defects [35] newer mass spectrometric approaches have since been used including electrospray ionization tandem mass spectrometry (ESI-MS) [36] and gene sequencing techniques. However, mass spectrometry continues to offer the fastest and most accurate method of screening for these disorders because the mass spectra generated (Figure 33.3) permit accurate identification of the lack of primary bile acids, the presence of atypical bile acids specific to each defect, and the potential to identify previously unidentified defects. To date, at least eight well-defined defects in bile acid synthesis have been described [7, 11, 20, 25, 26, 30, 31, 37, 38] and all have a highly variable phenotypic expression of familial and progressive infantile or late-onset cholestasis, of syndromes of fat-soluble vitamin malabsorption, and of variable degrees of neurological involvement (Table 33.2). Although the incidence of these defects is largely speculative it may be safe to estimate it as a group (including peroxisomal disorders) as approximately 1:100,000. In certain populations in which there is consanguinity, the incidence is likely to be considerably higher [39, 40]. Based on experience from an international screening program at Cincinnati Children’s Hospital Medical Center, bile acid synthesis defects have been identified in 2% of 16,000 screened cases of idiopathic cholestatic liver disease in infants and children over the last two decades [35]. Broadly, these defects can be categorized as deficiencies in the activity of enzymes responsible for catalyzing reactions to the steroid nucleus, or to the side-chain. All are inherited as autosomal recessive conditions.
Figure 33.3 Reconstructed negative ion mass spectra generated from FAB-MS analysis of urine extracts typically observed in patients with six different genetic defects in bile acid synthesis. For simplicity, only the dominant and key diagnostic ions are shown for the specific metabolites formed in each genetic defect and the depiction of the chemical structure that characterize these diagnostic ions in the spectra.
Table 33.2 Comparative Features of Patients with Intrahepatic Cholestasis Due to Bile Acid Synthesis Disorders and Byler Syndrome, Recently Separated into PFIC-1 and PFIC-2
Feature | Bile acid defects | PFIC-1,*,2†, 4#, 5+ |
---|---|---|
Age at presentation | Variable, late onset | <1 year*† |
Prognosis | Excellent | Fatal in first† to second* decade |
Liver | Hepatosplenomegaly | Hepatosplenomegaly*† |
Pruritus | Usually absent | Severe*† |
Growth failure | Mild | Severe*† |
Jaundice | +/- | Mild/severe*† |
Serum transaminases | Elevated | Slight* to substantial† elevations |
Serum GGT | Generally normal | Normal*† |
Serum cholesterol | Normal or low | Low*† |
Fat-soluble vitamins | Malabsorption | Malabsorption*† |
Bile acids | No primary bile acids | Primary bile acids*† |
Treatment | Bile acid therapy | Transplantation*† |
* caused by FIC1 mutations
† caused by BSEP mutations
# caused by TJP mutations
+ caused by FXR mutations
Defects Involving Reactions to the Steroid Nucleus
Four defects have been identified involving enzymes catalyzing reactions that modify the AB-rings of the steroid nucleus (Figure 33.4). A 12α-hydroxylase (CYP8B1) defect was once proposed [41, 42] but never definitively confirmed. The clinical presentations of the 3β-hydroxy-Δ5-C27-steroid oxidoreductase (HSD3B7), Δ5-3-oxosteroid 5β-reductase (AKR1D1), and oxysterol 7α-hydroxylase (CYP7B1) deficiencies are of progressive cholestatic liver disease. Typical biochemical abnormalities include elevations in serum liver enzymes, a conjugated hyperbilirubinemia, low serum γ-glutamyl transpeptidase (GGT) and evidence of fat-soluble vitamin malabsorption. Serum cholesterol concentrations are generally normal. The early clinical history of these patients often includes fat-soluble vitamin malabsorption, and in some cases rickets precedes any evidence of liver dysfunction. These abnormalities are usually responsive to oral vitamin supplementation, but these patients eventually present later in life with hepatosplenomegaly and elevated serum liver enzymes. The 3β-hydroxy-Δ5-C27-steroid oxidoreductase deficiency is the most common of the bile acid synthetic defects [35], frequently accounting for cases of late-onset chronic cholestasis [[43, 44].
Cholesterol 7α-Hydroxylase (CYP7A1) Deficiency
Although not presenting as cholestatic liver disease, a deficiency in cholesterol 7α-hydroxylase was found to be responsible for hypertriglyceridemia and gallstone disease in three related adults [37]. This finding followed a screening of the CYP7A1 gene for mutations in patients presenting with elevated LDL-cholesterol who were resistant to HMG-CoA reductase inhibitors. All three patients were homozygous for a frameshift mutation and had serum cholesterol concentrations >300 mg/dL, LDL-cholesterol >180 mg/dL, and elevated triglycerides [37]. The heterozygous relatives had elevated cholesterol levels. There was no cholestasis, fibrosis, or inflammation but fatty changes on liver biopsy. Fecal bile acid analysis revealed markedly reduced total bile acid output (6% of normal) and a high [chenodeoxycholic + lithocholic]/[cholic + deoxycholic] acid ratio, consistent with preferential synthesis of chenodeoxycholic acid via the “acidic pathway” for bile acid synthesis.
Oxysterol 7α-Hydroxylase (CYP7B1) Deficiency
The discovery of a genetic defect in oxysterol 7α-hydroxylase emphasizes the quantitative importance of the “acidic” pathway for bile acid synthesis in early life [7]. In the neonatal period of humans, CYP7B1 may be more important than CYP7A1 for bile acid synthesis. This genetic defect presents as severe progressive cholestatic liver disease. It was first described in a ten-week-old boy of parents who were first cousins [7], but has more recently been identified in additional patients in early infancy [8–10].
The index patient had severe cholestasis, cirrhosis, and liver synthetic failure in early infancy [7]. He became progressively more jaundiced by eight weeks of age, had hepatosplenomegaly and markedly elevated serum aminotransferases with normal serum GGT. Liver biopsy revealed cholestasis, bridging fibrosis, extensive giant-cell transformation and bile duct proliferation. A similar clinical picture and liver histology was reported for a five-month old Taiwanese infant [8], a six-month-old Japanese infant [9], and a Pakistani infant [10]. Oral UDCA therapy proved ineffective or led to a deterioration in liver function tests in all of these patients, and oral cholic acid therapy was also ineffective in the index case, and OLT was performed at 4.5 months of age but the patient died three weeks later from disseminated EBV-related lymphoproliferative disease [7]. The Taiwanese infant died at 11 months of age before OLT could be performed but the infant from Japan underwent living donor transplantation with a graft from the mother who had a compound heterozygous mutation (R112X/R417C) in the CYP7B1 gene [8] and was reportedly still alive two years after transplantation. In the Pakistani infant treated with CDCA, the child survived without transplantation [10]. These examples highlight the severity of this bile acid synthetic defect. It is possible that this cause of idiopathic liver disease may go unrecognized due to its rapid downhill course in the early months of life. The therapeutic strategy for patients with this defect should in the future target downregulation of sterol 27-hydroxylase. Diagnosis of all four cases was by mass spectrometry. Urine FAB-MS analysis from the index case revealed an absence of primary bile acids and large concentrations of unsaturated monohydroxy-C24 bile acids as sulfate (m/z 453) and glyco-sulfate (m/z 510) conjugates (Figure 33.3) which were confirmed by GC-MS [7]. These atypical bile acids were the unsaturated monohydroxy-bile acids, 3β-hydroxy-5-cholenoic and 3β-hydroxy-5-cholestenoic acids, which accounted for 97% and 86% respectively of the total serum and urinary bile acids. Additionally, 27-hydroxycholesterol concentrations in serum and urine were >4,500 times normal and no 7α-hydroxylated sterols were detected [7]. Similar GC-MS profiles were reported for the two Asian and Pakistani infants [8–10). The formation of 3β-hydroxy-5-cholenoic and 3β-hydroxy-5-cholestenoic acids occurs exclusively via the acidic pathway [1, 6] and mass spectrometry definitively established a CYP7B1 deficiency while illustrating the importance of this pathway for bile acid synthesis in early life. Monohydroxy-bile acids with the 3β-hydroxy-Δ5 structure and oxysterols are good ligands for FXR which would suppress CYP7A1 thereby preventing bile acids being synthesized by the classical pathway. In the original case, molecular studies of the liver tissue confirmed the CYP7A1 gene to be normal but there was no measurable enzyme activity, or mRNA [7]. Furthermore, these unsaturated bile acids are extremely cholestatic and the hepatotoxicity in these patients would be exacerbated by the lack of primary bile acids necessary to maintain bile flow. Oxysterol 7α-hydroxylase is essential for protecting the liver from hepatotoxic and cholestatic 3β-hydroxy-Δ5 monohydroxy bile acids (Figure 33.5).
Molecular studies on liver tissue from the first patient showed no CYP7B1 activity, or mRNA, and gene sequencing revealed a homozygous missense mutation in CYP7B1 providing unambiguous confirmation of the genetic defect in the oxysterol 7α-hydroxylase [7]. In the Taiwanese infant, the mutation in CYP7B1 gene caused a homozygous stop codon [8], while the Japanese infant had a compound heterozygous mutation of the CYP7B1 gene [9]. The Pakistani patient had a homozygous missense mutation [10]]. In all cases the parents were heterozygous for the respective mutations.
12α-Hydroxylase (CYP8B1) Deficiency
Two separate reports suggested the occurrence of a defect in bile acid synthesis involving 12α-hydroxylation, a reaction required for the synthesis of cholic acid. The first report [41] described a ten-year-old girl with celiac disease who from the age of three years had steatorrhea and chronic constipation (bowel movements once every three to six weeks). In affected patients, cholic acid accounted for only 17% of the biliary bile acids and there was a failure to detect measureable CYP8B1 activity in a wedge biopsy of liver tissue [42]. A later report of a premature infant with severe hypoglycemia and cholestasis diagnosed with panhypopituitarism, a low biliary cholic/chenodeoxycholic acid ratio led to speculation that the liver disease was related to an immaturity in CYP8B1 [45]. No confirmatory molecular evidence for mutations in the CYP8B1 gene were found, and whether these patients had a primary enzyme defect in CYP8B1 remains uncertain.
3β-Hydroxy-Δ5 C27-Steroid Oxidoreductase (HSD3B7) Deficiency
This is the most common of the bile acid disorders and involves the second step in pathway – the conversion of 7α-hydroxycholesterol into 7α-hydroxy-4-cholesten-3-one, a reaction catalyzed by a microsomal 3β-hydroxy-Δ5-C27-steroid oxidoreductase [1]. A deficiency of this sterol-specific enzyme results in the accumulation of 7α-hydroxycholesterol within the hepatocyte. The other enzymes involved in bile acid synthesis catalyze the remaining transformations, including side-chain oxidation so that in place of the normal primary bile acids, C24-bile acids are synthesized retaining the 3β-hydroxy-Δ5– structure characteristic of the substrate for the enzyme (Figure 33.6) [11, 46–48]. Patients with a 3β-hydroxy-Δ5-C27-steroid oxidoreductase deficiency usually present with hepatomegaly, fat-soluble vitamin malabsorption, mild steatorrhea, and without pruritus [49]. This inborn error is associated with elevated serum total and conjugated bilirubin and aminotransferases and normal γ-GT concentration. Serum bile acid concentrations, when measured by enzymatic or immunoassay methods, are normal and seemingly incompatible with the extent of cholestasis. Therefore, the finding of a normal serum bile acid determination in the presence of elevated serum conjugated bilirubin may provide a further clue to this defect [40]. Histological examination of the liver of these patients shows hepatitis with the giant-cell transformation and cholestasis with canalicular plugs, bile stasis and inflammatory changes [11, 48, 49].
As with most of the inborn errors involving the reactions responsible for nuclear modification, the 3β-hydroxy-Δ5-C27-steroid oxidoreductase (HSD3B7) deficiency is progressive and is fatal if untreated. Age at onset and diagnosis is variable, ranging from three months into the adult years [35, 44, 48–51]. The median age at diagnosis for this defect was three years in the registration trial leading to FDA approval of cholic acid for treatment [52]. It is clear that patients can present later in life as illustrated by a 24-year-old woman with cirrhosis of unknown etiology who was identified with a sister and first cousin who had died of cirrhosis at ages 19 and six years, respectively, and another 32-year old first cousin was also affected with a homozygous mutation in the HSD3B7 gene which was biochemically confirmed by FAB-MS analysis of the serum from family members [43].
Diagnosis of the 3β-hydroxyΔ-C27-steroid oxidoreductase deficiency is definitively established by urine FAB-MS or ESI-MS mass spectrometry analysis [11, 35, 36], which reveals an absence of the normal glycine and taurine conjugated primary bile and the presence of the sulfate and glyco-sulfate conjugates 3β,7α-dihydroxy- and 3β,7α,12α-trihydroxy-5-cholenoic acids [11] or by genetic analysis of HSD3B7 [12]. Tetrahydroxy- and pentahydroxy-bile alcohols with a 3β,7α-dihydroxy-Δ5– and 3β,7α,12α-trihydroxy-Δ5 nucleus are also found in greatly increased amounts in the urine, plasma and bile [47]. These bile alcohols are mainly sulfated, in contrast to the glucuronide conjugates of saturated bile alcohols observed in CTX [35]. Although primary bile acids are not detectable in the urine, the bile may contain small proportions of cholic acid resulting from intestinal bacterial metabolism of the 3β-hydroxy-Δ5 bile acids during enterohepatic recycling and this may facilitate bile secretion to explain the delay in onset of cholestasis and longer survival of these patients. Initial sequencing of the HSD3B7 gene localized to chromosome 16p11.2-12 has failed to find a common mutation for this disorder. In 16 patients 12 different mutations were identified that included point mutations, small insertions, and deletions [12], illustrating the diverse nature of the genetics.
The mechanism of liver injury is believed to be related to the accumulation of atypical bile acids concomitant with a lack of primary bile acids. In animal models, 3β-hydroxy-5-cholenoic acid produces cholestasis, but this is not the case for 3β,7α-dihydroxy-5-cholenoic acid which is rapidly metabolized to chenodeoxycholic acid. This conversion does not occur in patients lacking the 3β-hydroxy-Δ5-C27-steroid oxidoreductase enzyme. Studies using rat liver canalicular membrane vesicles have shown tauro-3β,7α-dihydroxy-5-cholenoic acid to be markedly cholestatic [53].
Oral administration of the primary bile acid, cholic acid (10–15 mg/kg bw/day), recently approved by the FDA and EMA, is now the recommended therapeutic approach for these patients and leads to resolution of the biochemical and histological abnormalities and to improve growth [35, 48, 49, 52, 54, 55]. Chenodeoxycholic acid has also been effective [47, 56–57), but is potentially hepatotoxic and more cathartic than cholic acid causing loose stools or even diarrhea in young infants. Some patients have been maintained temporarily on UDCA [48], which is choleretic but does not inhibit bile acid synthesis, or a combination of UDCA and chenodeoxycholic acid. Cholic acid is a ligand for FXR which downregulates hepatic CYP7A1 activity to limit production of hepatotoxic 3β-hydroxy-Δ5 bile acids, while additionally providing the stimulus for bile-flow.
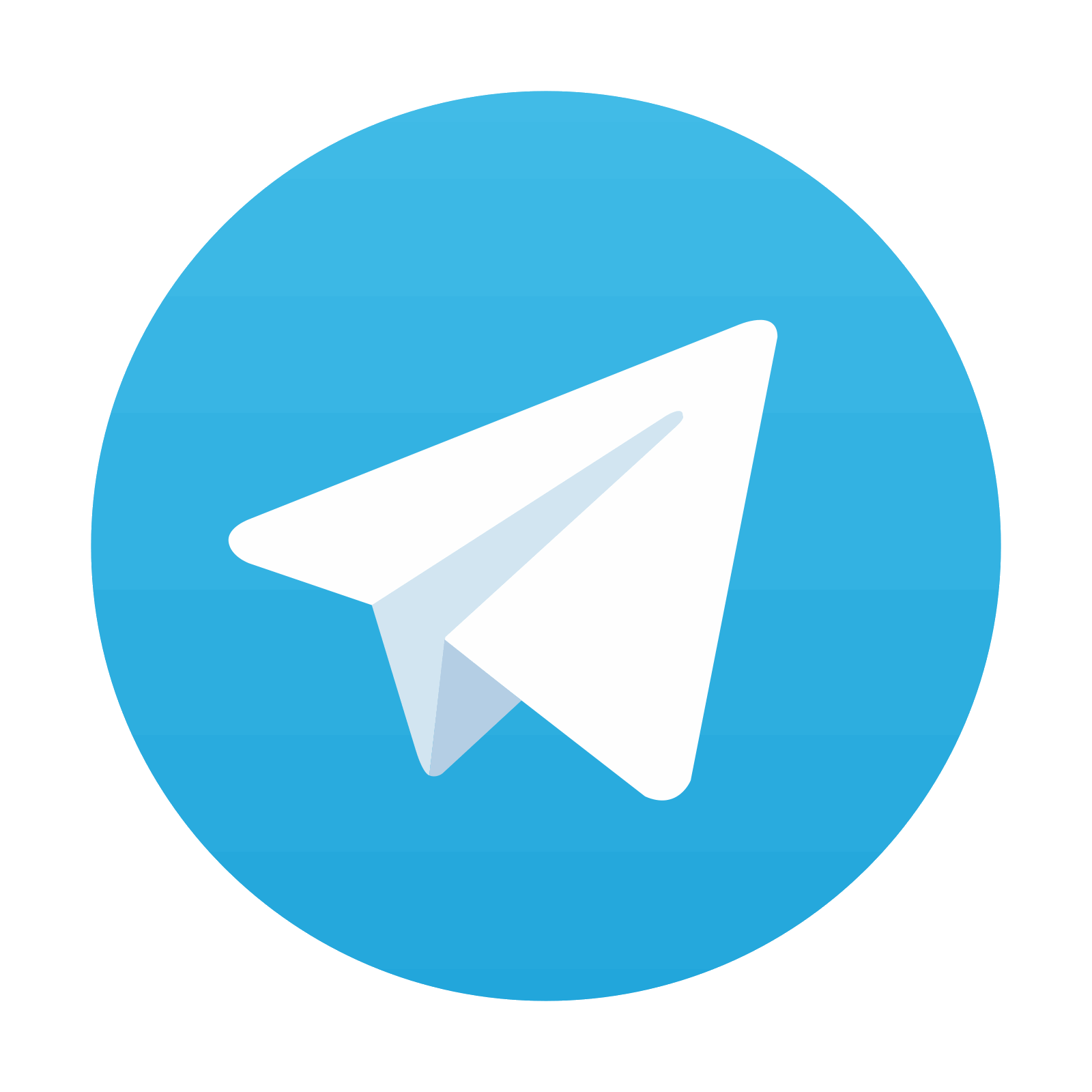
Stay updated, free articles. Join our Telegram channel
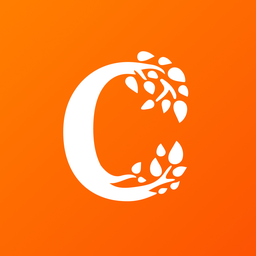
Full access? Get Clinical Tree
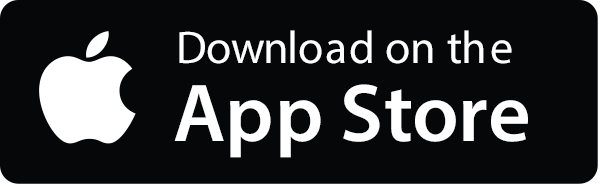
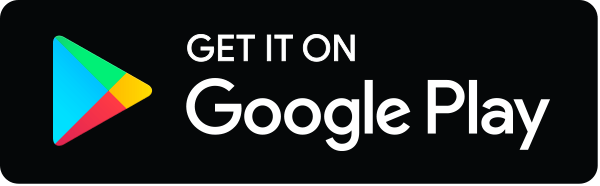
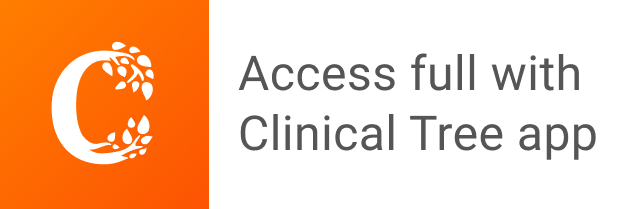