Chapter Outline
CELLULAR ORIGIN OF EICOSANOIDS, 355
CYCLO-OXYGENASE PATHWAY, 355
Molecular Biology, 355
Regulation of Cyclo-Oxygenase Gene Expression, 357
Regulation of Cyclo-Oxygenase Expression by Antiinflammatory Steroids, 357
Enzymatic Chemistry, 357
RENAL COX-1 AND COX-2 EXPRESSION, 357
COX-2 Expression in the Kidney, 357
COX-2 Expression in the Renal Cortex, 357
COX-1 Expression in the Kidney, 361
RENAL COMPLICATIONS OF NONSTEROIDAL ANTIINFLAMMATORY DRUGS, 361
Na + Retention, Edema, and Hypertension, 361
Hyperkalemia, 362
Papillary Necrosis, 362
Acute Kidney Injury, 362
Interstitial Nephritis, 363
Nephrotic Syndrome, 363
Renal Dysgenesis, 363
CARDIOVASCULAR EFFECTS OF COX-2 INHIBITORS, 363
PROSTANOID SYNTHASES, 364
Sources and Nephronal Distribution of Cyclo-Oxygenase Products, 364
PROSTANOID RECEPTORS, 366
EFFECTS OF COX-1 AND COX-2 METABOLITES ON SALT AND WATER TRANSPORT, 373
METABOLISM OF PROSTAGLANDINS, 374
15-Ketodehydrogenase, 374
ω/ω-1 Hydroxylation of Prostaglandins, 374
Cyclopentenone Prostaglandins, 374
Nonenzymatic Metabolism of Arachidonic Acid, 375
Prostaglandin Transport and Urinary Excretion, 375
INVOLVEMENT OF CYCLO-OXYGENASE METABOLITES IN RENAL PATHOPHYSIOLOGY, 375
Experimental and Human Glomerular Injury, 375
Acute Kidney Injury, 377
Urinary Tract Obstruction, 377
Allograft Rejection and Cyclosporine Nephrotoxicity, 378
Hepatic Cirrhosis and Hepatorenal Syndrome, 378
Pregnancy, 378
Lithium Nephrotoxicity, 379
Role of Reactive Oxygen Species as Mediators of COX-2 Actions, 379
LIPOXYGENASE PATHWAY, 379
Biologic Activities of Lipoxygenase Products in the Kidney, 381
Involvement of Lipoxygenase Products in Renal Pathophysiology, 381
CYTOCHROME P450 PATHWAY, 382
Vasculature, 383
Tubules, 384
Role in Acute and Chronic Kidney Disease, 385
Role in Hypertension, 385
Cellular Origin of Eicosanoids
Eicosanoids comprise a family of biologically active, oxygenated arachidonic acid (AA) metabolites. Arachidonic acid is a polyunsaturated fatty acid possessing 20 carbon atoms and four double bonds (C20:4) and is formed from linoleic acid (C18:2) by the addition of two carbons to the chain and further desaturation. In mammals, linoleic acid is derived strictly from dietary sources. Essential fatty acid (EFA) deficiency occurs when dietary fatty acid precursors, including linoleic acid, are omitted, thereby depleting the hormone-responsive pool of AA. EFA deficiency thereby reduces the intracellular availability of AA in response to hormonal stimulation and abrogates many biologic actions of hormone-induced eicosanoid release.
Of an approximate 10 g of linoleic acid ingested/day, only about 1 mg/day is eliminated as an end product of AA metabolism. Following its formation, AA is esterified into cell membrane phospholipids, principally at the 2 position of the phosphatidylinositol fraction (i.e. sn-2–esterified AA), the major hormone-sensitive pool of AA that is susceptible to release by phospholipases.
Multiple stimuli lead to the release of membrane-bound AA via activation of cellular phospholipases, principally isoforms of phospholipase A 2 (PLA 2 ). This cleavage step is rate-limiting in the production of biologically relevant arachidonate metabolites. In the case of PLA 2 activation, membrane receptors activate guanine nucleotide–binding (G) proteins, leading to the release of AA directly from membrane phospholipids. Activation of phospholipase C or D, on the other hand, releases AA via the sequential action of the phospholipase-mediated production of diacylglycerol (DAG), with subsequent release of AA from DAG by DAG lipase. This pathway may also lead to the formation of the esterified AA metabolites arachidonoylethanolamide (AEA) and 2-arachidonoylglycerol (2-AG), which are endocannabinoids. These endocannabinoids can subsequently be converted to free AA by the action of monoacylgycerol lipase. When considering eicosanoid formation, the physiologic significance of AA release by these other phospholipases remains uncertain because, at least in the setting of inflammation, PLA 2 action appears essential for the generation of biologically active AA metabolites.
More than 15 proteins with PLA 2 activity are known to exist, including secreted (sPLA 2 ) and cytoplasmic PLA 2 (cPLA 2 ) isoforms. A mitogen-activated cytoplasmic PLA 2 has been found to mediate AA release in a calcium-calmodulin–dependent manner. Other hormones and growth factors, including epidermal growth factor (EGF) and platelet-derived growth factors, activate PLA 2 directly through tyrosine residue kinase activity, allowing the recruitment of co-activators to the enzyme without an absolute requirement for the intermediate action of Ca 2+ -calmodulin or other cellular kinases.
Following de-esterification, AA is rapidly re-esterified into membrane lipids or avidly bound by intracellular proteins, in which case it becomes unavailable to further metabolism. Should it escape re-esterification and protein binding, free AA becomes available as a substrate for one of three major enzymatic transformations, the common result of which is the incorporation of oxygen atoms at various sites of the fatty acid backbone, with accompanying changes in its molecular structure (e.g., ring formation). This results in the formation of biologically active molecules, referred to as eicosanoids. The specific nature of the products generated is a function of the initial stimuli for AA release, as well as the metabolic enzyme available, which is determined in part by the cell type involved.
These products, in turn, mediate or modulate the biologic actions of the agonist in question. AA release may also result from nonspecific stimuli, such as cellular trauma, including ischemia and hypoxia, oxygen free radicals, or osmotic stress. The identity of the specific AA metabolite generated in a particular cell system depends on the proximate stimulus and availability of the downstream AA-metabolizing enzymes present in that cell.
Three major enzymatic pathways of free AA metabolism are present in the kidney: cyclo-oxygenases, lipoxygenases, and cytochrome P450 ( Figure 14.1 ). The cyclo-oxygenase (COX) pathway mediates the formation of prostaglandins (PGs) and thromboxanes, the lipoxygenase (LO) pathway mediates the formation of mono-, di-, and tri-hydroxyeicosatetraenoic acids (HETEs), leukotrienes (LTs), and lipoxins (LXs), and the cytochrome P450–dependent oxygenation of AA mediates the formation of epoxyeicosatrienoic acids (EETs), their corresponding diols, HETEs, and monooxygenated AA derivatives. Fish oil diets, rich in ω-3 polyunsaturated fatty acids, interfere with metabolism via all three pathways by competing with AA oxygenation, resulting in the formation of biologically inactive end products. Interference with the production of proinflammatory lipids has been hypothesized to underlie the beneficial effects of fish oil in immunoglobulin A (IgA) nephropathy and other cardiovascular diseases. The following sections deal with the current understanding of the chemistry, biosynthesis, renal metabolism, mechanisms of release, receptor biology, signal transduction pathways, biologic activities, and functional significance of each of the metabolites generated by the three major routes of AA metabolism in the kidney.

Cyclo-Oxygenase Pathway
Molecular Biology
The cyclo-oxygenase enzyme system is the major pathway for AA metabolism in the kidney ( Figure 14.2 ). Cyclo-oxygenase (prostaglandin [PG] synthase G 2 /H 2 ) is the enzyme responsible for the initial conversion of arachidonic acid to PGG 2 and subsequently to PGH 2 . COX was first purified from ram seminal vesicles and cloned in 1988. The protein is widely expressed, and the level of activity is not dynamically regulated. Other studies supported the presence of a COX that was dynamically regulated and responsible for increased prostanoid production in inflammation. This second inducible COX isoform was identified shortly after the cloning of the initial enzyme and designated COX-2, whereas the initially isolated isoform is now designated COX-1. COX-1 and COX-2 are encoded by distinct genes located on different chromosomes. The human COX-1 gene ( PTGS1, PG synthase 1) is on chromosome 9, whereas COX-2 is localized on chromosome 1. The genes are also subject to dramatically different regulatory signals.

Regulation of Cyclo-Oxygenase Gene Expression
At the cellular level, COX-2 expression is highly regulated by several processes that alter its transcription rate, message export from the nucleus, message stability, and efficiency of message translation. These processes tightly control the expression of COX-2 in response to many of the same cellular stresses that activate arachidonate release (e.g., cell volume changes, shear stress, hypoxia), as well as a variety of cytokines and growth factors, including tumor necrosis factor (TNF), interleukin-1β, EGF, and platelet-derived growth factor (PDGF). Activation of COX-2 gene transcription is mediated via the coordinated activation of several transcription factors that bind to and activate consensus sequences in the 5′ flanking region of the COX-2 gene for nuclear factor-kappaB (NF-κB), and NF–interleukin-6 (IL-6)/CCAAT-enhancer binding proteins (C-EBP), and a cyclic adenosine monophosphate (cAMP) response element (CRE). Induction of COX-2 messenger RNA (mRNA) transcription by endotoxin (lipopolysaccharide) may also involve CRE sites and NF-κB sites.
Regulation of Cyclo-Oxygenase Expression By Antiinflammatory Steroids
A molecular basis linking the antiinflammatory effects of COX-inhibiting nonsteroidal antiinflammatory drugs (NSAIDs) and antiinflammatory glucocorticoids has long been sought. A novel mechanism for the suppression of arachidonate metabolism by corticosteroids involving translational inhibition of COX formation had been suggested prior to the molecular recognition of COX-2. With the cloning of COX-2, it became well established that glucocorticoids suppress COX-2 expression and prostaglandin synthesis, an effect now viewed as central to the antiinflammatory effects of glucocorticoids. Posttranscriptional control of COX-2 expression represents another robust mechanism whereby adrenal steroids regulate COX-2 expression. Accumulating evidence has suggested that COX-2 is modulated at multiple steps in addition to transcription rate, including stabilization of the mRNA and enhanced translation. Glucocorticoids, including dexamethasone, downregulate COX-2 mRNA in part by destabilizing the mRNA. The 3′ untranslated region of COX-2 mRNA contains 22 copies of an AUUUA motif, which are important in destabilizing the COX-2 message in response to dexamethasone, whereas other 3′ sequences appear important for COX-2 mRNA stabilization in response to IL-1β. Effects of the 3′ untranslated region (UTR), as well as other factors regulating the efficiency of COX-2 translation, have also been suggested. The factors determining the expression of COX-1 are more obscure.
Enzymatic Chemistry
Despite these differences, both PG synthases catalyze a similar reaction, resulting in cyclization of carbons 8 to 12 of the AA backbone, forming cyclic endoperoxide, accompanied by the concomitant insertion of two oxygen atoms at carbon 15 to form PGG 2 (a 15-hydroperoxide). In the presence of a reduced glutathione-dependent peroxidase, PGG 2 is converted to the 15-hydroxy derivative, PGH 2 . The endoperoxides (PGG 2 and PGH 2 ) have very short half-lives, about 5 minutes, and are biologically active in inducing aortic contraction and platelet aggregation. However, under some circumstances, the formation of these endoperoxides may be strictly limited via the self-deactivating properties of the enzyme.
The expression of recombinant enzymes and determination of the crystal structure of COX-2 have provided further insight into the observed physiologic and pharmacologic similarities to, and differences from, COX-1. It is now clear that COX-inhibiting NSAIDs work by sterically blocking access of AA to the heme-containing, active enzymatic site. Particularly well-conserved are sequences surrounding the aspirin-sensitive serine residues, at which acetylation by aspirin irreversibly inhibits activity. More recent evidence showed that COX-1 and COX-2 are capable of forming heterodimers and sterically modulating each other’s function. The substrate-binding pocket of COX-2 is larger and therefore accepting of bulkier inhibitors and substrates. This difference has allowed the development and marketing of relatively highly selective COX-2 inhibitors for clinical use as analgesics, antipyretics, and antiinflammatory agents. In addition to its central role in inflammation, aberrantly upregulated COX-2 expression has been implicated in the pathogenesis of a number of epithelial cell carcinomas and in Alzheimer’s disease and other degenerative neurologic conditions.
Renal COX-1 and COX-2 Expression
COX-2 Expression in the Kidney
There is now definitive evidence for significant COX-2 expression in the mammalian kidney ( Figure 14.3 ). COX-2 mRNA and immunoreactive COX-2 are present at low but detectable levels in normal adult mammalian kidney, where in situ hybridization and immunolocalization have demonstrated localized expression of COX-2 mRNA and immunoreactive protein in the cells of the macula densa and a few cells in the cortical thick ascending limb cells immediately adjacent to the macula densa. COX-2 expression is also abundant in the lipid-laden medullary interstitial cells in the tip of the papilla. Some investigators have reported that COX-2 may be expressed in inner medullary collecting duct cells or intercalated cells in the renal cortex. Nevertheless, COX-1 expression is constitutive and clearly the most abundant isoform in the collecting duct, so the potential existence and physiologic significance of COX-2 coexpression in this segment remains uncertain.

COX-2 Expression in the Renal Cortex
It is now well documented that COX-2 is expressed in the macula densa and cortical thick ascending limb (CTAL). It is expressed in human kidney, especially in kidneys of older adults, and in patients with diabetes mellitus, congestive heart failure, and Bartter-like syndrome.
The presence of COX-2 in the unique group of cells comprising the macula densa points to a potential role for COX-2–derived prostanoids in regulating glomerular function. Studies of the prostanoid-dependent control of glomerular filtration rate by the macula densa have suggested effects via vasodilator and vasoconstrictor effects of prostanoids contributing to tubuloglomerular feedback (TGF). Some studies have suggested COX-2–derived prostanoids are predominantly vasodilators. By inhibiting production of dilator prostanoids contributing to the patency of the adjacent afferent arteriole, COX-2 inhibition may contribute to the decline in glomerular filtration rate (GFR) observed in patients taking NSAIDs or selective COX-2 inhibitors (see later).
The volume-depleted state is typified by low NaCl delivery to the macula densa, and COX-2 expression in the macula densa is also increased in states associated with volume depletion ( Figure 14.4 ). Of note, COX-2 expression in cultured macula densa cells and CTAL cells is also increased in vitro by reducing extracellular Cl − concentration. Studies in which cortical thick limbs and associated glomeruli were removed and perfused from rabbits pretreated with a low- salt diet to upregulate macula densa COX-2 demonstrated COX-2–dependent release of PGE 2 from the macula densa in response to decreased chloride perfusate. Furthermore the induction of COX-2 by a low Cl − level can be blocked by a specific p38 mitogen-activated protein (MAP) kinase inhibitor. Finally , in vivo, renal cortical immunoreactive pp38 expression (the active form of p38) is predominantly localized to the macula densa and CTAL and increases in response to a low-salt diet. These findings point to a molecular pathway whereby enhanced COX-2 expression occurring in circumstances associated with intracellular volume depletion could result from decreased luminal Cl − delivery. Some studies have also indicated that the carbonic anhydrase inhibitor acetazolamide and dopamine may both indirectly regulate macula densa COX-2 expression by inhibiting proximal reabsorption and thereby increasing luminal macula densa Cl − delivery. In mice deficient in the Na + -H + exchanger subtype 2 (NHE2), the macula densa is shrunken, accompanied by increased COX-2 expression and juxtaglomerular renin expression, suggesting that NHE2 appears to be the major isoform associated with macula densa cell volume regulation.

In the mammalian kidney, the macula densa is involved in regulating renin release by sensing alterations in luminal chloride via changes in the rate of Na + -K + -2Cl − cotransport ( Figure 14.5 ). In vivo measurements in isolated perfused kidney and isolated perfused juxtaglomerular preparation have all indicated that administration of nonspecific COX inhibitors prevents the increases in renin release mediated by macula densa sensing of decreases in luminal NaCl. Induction of a high renin state by imposition of a salt-deficient diet, angiotensin-converting enzyme (ACE) inhibition, diuretic administration, or experimental renovascular hypertension all significantly increase macula densa–CTAL COX-2 mRNA and immunoreactive protein. COX-2–selective inhibitors blocked elevations in plasma renin activity, renal renin activity, and renal cortical renin mRNA in response to loop diuretics, ACE inhibitors, or a low-salt diet and, in an isolated perfused juxtaglomerular preparation, increased renin release in response to lowering the perfusate NaCl concentration was blocked by COX-2 inhibition. In COX-2 knockout mice, increases in renin in response to low salt or ACE inhibitors were significantly blunted but were unaffected in COX-1 knockout mice. COX-2-derived PGE 2 , activating the type 4 (EP4) receptors on juxtaglomerular cells, has been shown to be important for the macula densa regulation of renin release. Macula densa COX-2–derived prostanoids appear to be predominantly involved in setting tonic levels of juxtaglomerular renin expression rather than necessarily mediating acute renin release. There is evidence that the effect of ACE inhibitors and angiotensin receptor blockers (ARBs) to increase macula densa COX-2 expression is mediated by feedback of angiotensin II (Ang II) on the macula densa, with Ang II type 1 (AT 1 ) receptor activation inhibiting and AT 2 receptor activation stimulating COX-2 expression. In addition, prorenin and/or renin may stimulate macula densa COX-2 expression through activation of the prorenin receptor.

COX-2 inhibitors have also been shown to decrease renin production in models of renovascular hypertension, and studies in mice with targeted deletion of the prostacyclin receptor have suggested a predominant role for prostacyclin in mediating renin production and release in these models. In a model of sepsis, COX-2 expression increased in the macula densa and both cortical and medullary TAL. This increased COX-2 expression was mediated by Toll-like receptor 4 (TLR4) and in TLR4 −/− mice, juxtaglomerular apparatus renin expression was absent.
In addition to mediating juxtaglomerular renin expression, COX-2 metabolites may also modulate tubuloglomerular feedback (TGF). However, using different methodologies, investigators have reported that COX-2 metabolites predominantly modulate TGF by the production of vasodilatory prostanoids or mediate afferent arteriolar vasoconstriction by activating thromboxane receptors through the generation of thromboxane A 2 (TXA 2 ) and/or PGH 2 . Further studies will be required to reconcile these divergent results.
There is evidence that macula densa COX-2 expression is sensitive not only to alterations in intravascular volume, but also to alterations in renal metabolism. Specifically, the G protein–coupled receptor, GPR91, has been shown to be a receptor for succinate, an intermediate of the citric acid cycle. GPR91 is expressed in the macula densa, and GPR91, and intrarenal production of succinate are increased in diabetes. Studies have suggested that succinate activation of GPR91 leads to increased macula densa COX-2 expression.
COX-2 Expression in the Renal Medulla
The renal medulla is a major site of prostaglandin synthesis and abundant COX-1 and COX-2 expression ( Figure 14.6 ). COX-1 and COX-2 exhibit differential compartmentalization within the medulla, with COX-1 predominating in the medullary collecting ducts and COX-2 predominating in medullary interstitial cells. In the collecting duct, COX-2 has also been localized to intercalated cells but is absent in principal cells. COX-2 may also be expressed in endothelial cells of the vasa recta supplying the inner medulla.

In medullary interstitial cells, dynamic regulation of COX-2 expression appears to be an important adaptive response to physiologic stresses, including water deprivation, increased dietary sodium, and exposure to endotoxins. In contrast, COX-1 expression is unaffected by water deprivation. Although hormonal factors could also contribute to COX-2 induction, shifting cultured renal medullary interstitial cells to hypertonic media (using NaCl or mannitol) is sufficient to induce COX-2 expression directly. Because prostaglandins play an important role in maintaining renal function during volume depletion or water deprivation, induction of COX-2 by hypertonicity provides an important adaptive response.
As is the case for the macula densa, medullary interstitial cell COX-2 expression is transcriptionally regulated in response to renal extracellular salt and tonicity. Water deprivation and a high-sodium diet both induce COX-2 expression in medullary interstitial cells by activating the NF-κB pathway. There is also evidence that nitric oxide may modulate medullary COX-2 expression through MAP kinase–dependent pathways. The mechanisms underlying the upregulation of medullary COX-2 expression in response to volume expansion are probably multifactorial. There is clear evidence that increased medullary tonicity increases medullary COX-2 expression. Different studies have indicated a role for NF-κB, EGF receptor (EGFR) transactivation, and mitochondrial-generated ROS. Whether these represent parallel pathways or are all interrelated is not yet clear; however, it should be noted that the described EGFR transactivation is mediated by cleavage of the EGFR ligand, transforning growth factor-α, by ADAM17 (known as TACE [tumor necrosis factor-α–converting enzyme]). This is known to be activated by src, which can be activated by reactive oxygen species (ROS). In addition to medullary COX-2, cortical COX-2 expression increases in salt-sensitive hypertension, especially in the glomerulus and is inhibited by the superoxide dismutase mimetic, tempol, or an ARB.
COX-1 Expression in the Kidney
Although well-defined factors regulating COX-2 and determining the role of COX-2 expression in the kidney are being delineated, the role of renal COX-1 remains more obscure. COX-1 is constitutively expressed in platelets in renal microvasculature and glomerular parietal epithelial cells ( Figure 14.7 ). In addition, COX-1 is abundantly expressed in the collecting duct, but there is little COX-1 expressed in the proximal tubule or TAL. Although COX-1 expression levels do not appear to be dynamically regulated and, consistent with this observation, the COX-1 promoter does not possess a TATA box, vasopressin does increase COX-1 expression in collecting duct epithelial cells and in interstitial cells in the inner medulla. The factors accounting for the tissue-specific expression of COX-1 are uncertain but may involve histone acetylation and the presence of two tandem Sp1 sites in the upstream region of the gene.

Renal Complications of Nonsteroidal Antiinflammatory Drugs
Na + Retention, Edema, and Hypertension
Use of nonselective NSAIDs may be complicated by the development of significant Na + retention, edema, congestive heart failure and hypertension. These complications are also apparent in patients using COX-2–selective NSAIDs. Studies with celecoxib and rofecoxib have demonstrated that like nonselective NSAIDs, these COX-2–selective NSAIDs reduce urinary Na + excretion and are associated with modest Na + retention in otherwise healthy subjects. COX-2 inhibition likely promotes salt retention via multiple mechanisms ( Figure 14.8 ). A reduced GFR may limit the filtered Na + load and salt excretion. In addition, PGE 2 directly inhibits Na + absorption in the thick ascending limb (TAL) and collecting duct. The relative abundance of COX-2 in medullary interstitial cells places this enzyme adjacent to both these nephron segments, allowing for COX-2–derived PGE 2 to modulate salt absorption. COX-2 inhibitors decrease renal PGE 2 production and thereby may enhance renal sodium retention. Finally, reduction in renal medullary blood flow by the inhibition of vasodilator prostanoids may significantly reduce renal salt excretion and promote the development of edema and hypertension. COX-2–selective NSAIDs have been demonstrated to exacerbate salt-dependent hypertension. Similarly, patients with preexisting treated hypertension commonly experience hypertensive exacerbations with COX-2–selective NSAIDs. Taken together, these data suggest that COX-2–selective NSAIDs have effects similar to those of nonselective NSAIDs with respect to salt excretion.

Hyperkalemia
Nonselective NSAIDs cause hyperkalemia due to suppression of the renin angiotensin aldosterone axis. Both a decreased GFR and inhibition of renal renin release may compromise renal K + excretion. Patients on a salt-restricted diet also have decreased urinary potassium excretion when treated with a COX-2–selective inhibitor, and COX-2–selective inhibitors may pose an equal or greater risk as nonselective NSAIDs for the development of hyperkalemia.
Papillary Necrosis
Acute and subacute forms of papillary necrosis have been observed with NSAID use. Acute NSAID-associated renal papillary injury is more likely to occur in the setting of volume depletion, suggesting a critical dependence of renal function on COX metabolism in this setting. Long-term use of NSAIDs has been associated with papillary necrosis and progressive renal structural and functional deterioration, much like the syndrome of analgesic nephropathy observed with an acetaminophen, aspirin, and caffeine combination. Experimental studies have suggested that renal medullary interstitial cells are an early target of injury in analgesic nephropathy. COX-2 has been shown to be an important survival factor for cells exposed to a hypertonic medium. The coincident localized expression of COX-2 in these interstitial cells raises the possibility that, like nonselective NSAIDs, long-term use of COX-2–selective NSAIDs may contribute to the development of papillary necrosis.
Acute Kidney Injury
Acute kidney injury (AKI) is a well-described complication of NSAID use. This is generally considered to be a result of altered intrarenal microcirculation and glomerular filtration secondary to the inability to produce beneficial endogenous prostanoids when the kidney is dependent on them for normal function. Like the traditional, nonselective NSAIDs, COX-2– selective NSAIDs will also reduce glomerular filtration in susceptible patients. Although generally rare, NSAID-associated renal insufficiency occurs in a significant proportion of patients with underlying volume depletion, renal insufficiency, congestive heart failure, diabetes, and advanced age. These risk factors are additive and rarely are present in patients included in study cohorts used for safety assessment of these drugs. It is therefore relevant that celecoxib and rofecoxib caused a slight but significant fall in the GFR in salt-depleted but otherwise healthy subjects. Similar to nonselective NSAIDs, AKI can occur secondary to the use of COX-2–selective NSAIDs. Preclinical studies have supported the concept that inhibition of COX-2–derived prostanoids generated in the macula densa contributes to a fall in GFR by reducing the diameter of the afferent arteriole. In vivo video microscopy studies have documented reduced afferent arteriolar diameter following administration of a COX-2 inhibitor. These animal data not only support the concept that COX-2 plays an important role in regulating the GFR, but also the clinical observations that COX-2–selective inhibitors can cause renal insufficiency similar to that reported with nonselective NSAIDs.
Interstitial Nephritis
The gradual development of renal insufficiency characterized by a subacute inflammatory interstitial infiltrate may occur after several months of continuous NSAID ingestion. Less commonly, the interstitial nephritis and renal failure may be fulminant. The infiltrate is typically accompanied by eosinophils; however, the clinical picture is typically much less dramatic than that of classic drug-induced allergic interstitial nephritis, lacking fever or rash. This syndrome has also been reported with the COX-2–selective drug celecoxib. Dysregulation of the immune system is thought to play an important role in the syndrome, which typically abates rapidly following discontinuation of the NSAID or COX-2 inhibitor.
Nephrotic Syndrome
Like interstitial nephritis, nephrotic syndrome typically occurs in patients chronically ingesting any one of a myriad of NSAIDs over a course of months. The renal pathology is usually consistent with minimal change disease, with foot process fusion of glomerular podocytes observed on electron microscopy (EM), but membranous nephropathy has also been reported. Typically, the nephrotic syndrome occurs together with the interstitial nephritis. Nephrotic syndrome without interstitial nephritis may occur, as well as immune complex glomerulopathy, in a small subset of patients receiving NSAIDs. It remains uncertain whether this syndrome results from mechanism-based COX inhibition by these drugs, an idiosyncratic immune drug reaction, or a combination of both.
Renal Dysgenesis
Reports of renal dysgenesis and oligohydramnios in the offspring of women given nonselective NSAIDs during the third trimester of pregnancy have implicated prostaglandins in the process of normal renal development. A similar syndrome of renal dysgenesis has been reported in mice with targeted disruption of the COX-2 gene, as well as mice treated with the specific COX-2 inhibitor SC58236. Because neither COX-1 −/− mice nor mice treated with the COX-1– selective inhibitor SC58560 exhibited altered renal development, a specific role for COX-2 in nephrogenesis has been suggested. A report of renal dysgenesis in the infant of a woman exposed to the COX-2–selective inhibitor nimesulide suggested that COX-2 also plays a role in renal development in humans.
The intrarenal expression of COX-2 in the developing kidney peaks in the mouse at postnatal day 4 and in the rat in the second postnatal week. It has not yet been determined if a similar pattern of COX-2 is seen in humans. Although the most intense staining is observed in a small subset of cells in the nascent macula densa and CTAL, expression in the papilla has also been observed. Considering the similar glomerular developmental defects observed in rodents treated with the COX-2 inhibitor and in mice with targeted disruption of the COX-2 gene, it seems likely that prostanoids or other products resulting from COX-2 activity in the CTAL (and macula densa) act in a paracrine manner to influence glomerular development. The identity of the COX-2–derived prostanoids that promote glomerulogenesis remains uncertain. In vitro studies have shown that exogenous PGE 2 promotes renal metanephric development and is a critical growth factor for renal epithelia cells. Nevertheless, none of the prostaglandin receptor knockout mice recapitulated the phenotype of the COX-2 knockout mice.
Cardiovascular Effects of COX-2 Inhibitors
Effects of COX-2 Inhibition on Vascular Tone
In addition to their propensity to reduce renal salt excretion and decrease medullary blood flow, NSAIDs and selective COX-2 inhibitors have been shown to exert direct effects on systemic resistance vessels. The acute pressor effect of angiotensin infusion in human subjects was significantly increased by pretreatment with the nonselective NSAID indomethacin at all Ang II doses studied. Administration of selective COX-2 inhibitors or COX-2 gene knockout has been shown to accentuate the pressor effects of angiotensin II in mice. These studies also demonstrated that Ang II–mediated blood pressure increases were markedly reduced by administration of a selective COX-1 inhibitor or in COX-1 gene knockout mice. These findings support the conclusion that COX-1–derived prostaglandins participate in, and are integral to, the pressor activity of Ang II, whereas COX-2–derived prostaglandins are vasodilators that oppose and mitigate the pressor activity of Ang II. Other animal studies have more directly shown that NSAIDs and COX-2 inhibitors blunt arteriolar dilation and decrease flow through resistance vessels.
Increased Cardiovascular Thrombotic Events
COX-2 is known to be induced in vascular endothelial cells in response to shear stress, and selective COX-2 inhibition reduces circulating prostacyclin levels in normal human subjects. Therefore, increasing evidence has indicated that COX-2–selective antagonism may carry increased thrombogenic risks due to selective inhibition of the endothelial-derived, antithrombogenic prostacyclin without any inhibition of the prothrombotic, platelet-derived thromboxane generated by COX-1. Although animal studies have provided conflicting results about the role of COX-2 inhibition on development of atherosclerosis, there have been indications that COX-2 inhibition may destabilize atherosclerotic plaques. This has been suggested by studies indicating increased COX-2 expression and colocalization with microsomal PGE synthase 1 and metalloproteinases-2 and -9 in carotid plaques from individuals with symptomatic disease before endarterectomy. Because of the concerns about increased cardiovascular risk, two selective COX-2 inhibitors, rofecoxib and valdecoxib, were withdrawn from the market, and remaining coxibs and other NSAIDs have been relabeled to highlight the increased risk for cardiovascular events.
Prostanoid Synthases
Once PGH 2 is formed in the cell, it can undergo a number of possible transformations, yielding biologically active prostaglandins and TXA 2 . As seen in Figure 14.9 , in the presence of isomerase and reductase enzymes, PGH 2 is converted to PGE 2 and PGF 2α , respectively. Thromboxane synthase converts PGH 2 into a bicyclic oxetane-oxane ring metabolite, TXA 2 , a prominent reaction product in the platelet and an established synthetic pathway in the glomerulus. Prostacyclin synthase, a 50-kDa protein located in plasma and nuclear membranes and found mostly in vascular endothelial cells, catalyzes the biosynthesis of prostacyclin, PGI 2 . PGD 2 , the major prostaglandin product in mast cells, is also derived directly from PGH 2 , but its role in the kidney is uncertain. The enzymatic machinery and their localization in the kidney are discussed in detail later.

Sources and Nephronal Distribution of Cyclo-Oxygenase Products
COX activity is present in arterial and arteriolar endothelial cells, including glomerular afferent and efferent arterioles. The predominant metabolite from these vascular endothelial cells is PGI 2 . Whole glomeruli generate PGE 2 , PGI 2 , PGF 2α and TXA 2 . The predominant products in rat and rabbit glomeruli are PGE 2 , followed by PGI 2 and PGF 2α and, finally, TXA 2 .
Analysis of individual cultured glomerular cell subpopulations has also provided insight into the localization of prostanoid synthesis. Cultured mesangial cells are capable of generating PGE 2 , and, in some cases, PGF 2α and PGI 2 have also been detected. Other studies have suggested that mesangial cells may produce the endoperoxide PGH 2 as a major COX product. Glomerular epithelial cells also appear to participate in prostaglandin synthesis, but the profile of COX products generated in these cells remains controversial. Immunocytochemical studies of rabbit kidney have demonstrated intense staining for COX-1, predominantly in parietal epithelial cells. Glomerular capillary endothelial cell PG generation profiles remain undefined but may include prostacyclin.
The predominant synthetic site of prostaglandin synthesis along the nephron is the cortical collecting duct (CCD), particularly its medullary portion (MCT). In the presence of exogenous arachidonic acid, PGE 2 is the predominant PG formed in the collecting duct, with variations among the other products being insignificant. PGE 2 is also the major COX metabolite generated in medullary interstitial cells. The role that specific prostanoid synthases may play in the generation of these products is outlined below.
Thromboxane Synthase
TXA 2 is produced from PGH 2 by thromboxane synthase (TXAS), a microsomal protein of 533 amino acids with a predicted molecular weight of approximately 60 kDa. The amino acid sequence of the enzyme exhibits homology to the cytochrome P450s and is now classified as CYP5A1. The human gene is localized on chromosome 7q and spans 180 kb. TXAS mRNA is highly expressed in hematopoietic cells, including platelets, macrophages, and leukocytes. TXAS mRNA is expressed in the thymus, kidney, lung, spleen, prostate, and placenta. Immunolocalization of TXAS demonstrates high expression in the dendritic cells of the interstitium, with lower expression in glomerular podocytes of human kidney. TXA 2 synthase expression is regulated by dietary salt intake. Furthermore experimental use of ridogrel, a specific thromboxane synthase inhibitor, reduced blood pressure in spontaneously hypertensive rats. The clinical use of TXA 2 synthase inhibitors is complicated by the fact that its endoperoxide precursors (PGG 2 , PGH 2 ) are also capable of activating its downstream target, the TP receptor.
Prostacyclin Synthase
There are many biologic effects of prostacyclin and include nociception and antithrombotic and vasodilator actions, which have been targeted therapeutically to treat pulmonary hypertension.
Prostacyclin (PGI 2 ) is derived by the enzymatic conversion of PGH 2 via prostacyclin synthase (PGIS). The cloned cDNA contains a 1500-bp open reading frame that encodes a 500–amino acid protein of approximately 56 kDa. The human prostacyclin synthase gene is present as a single copy per haploid genome and is localized on chromosome 20q. Northern blot analysis shows that prostacyclin synthase mRNA is widely expressed in human tissues and is particularly abundant in the ovary, heart, skeletal muscle, lung, and prostate. PGI synthase expression exhibits segmental expression in the kidney, especially in kidney inner medulla tubules and interstitial cells.
Recently, PGI 2 synthase null mice were generated. PGI 2 levels in the plasma, kidneys, and lungs were reduced, documenting the role of this enzyme as an in vivo source of PGI 2 . Blood pressure and blood urea nitrogen and creatinine levels in the PGI 2 synthase knockout mice were significantly increased; renal pathologic findings included surface irregularity, fibrosis, cysts, arterial sclerosis, and hypertrophy of vessel walls. Thickening of the thoracic aortic media and adventitia were observed in aged PGI 2 synthase null mice. Interestingly, this is a phenotype different from that reported for the IP receptor knockout mouse. These differences indicate the presence of additional IP-independent, PGI 2 -activated signaling pathways. Regardless, these findings demonstrate the importance of PGI 2 to the maintenance of blood vessels and the kidney.
Prostaglandin D Synthase
Prostaglandin D 2 is derived from PGH 2 via the action of specific enzymes designated PGD synthases (PGDSs). Two major enzymes are capable of transforming PGH 2 to PGD 2 , including a lipocalin-type PGD synthase and a hematopoietic-type PGDS. Mice lacking the lipocalin D synthase gene exhibit altered sleep and pain sensation. PGD 2 is the major prostanoid released from mast cells following challenge with immunoglobulin E. The kidney also appears capable of synthesizing PGD 2 . RNA for the lipocalin-type PGD synthase has been reported to be widely expressed along the rat nephron, whereas the hematopoietic-type PGD synthase is restricted to the collecting duct. Urinary excretion of lipocalin D synthase has been proposed as a biomarker predictive of renal injury, and lipocalin D synthase knockout mice appear to be more prone to diabetic nephropathy. However, the physiologic roles of these enzymes in the kidney remain less certain. Once synthesized, PGD 2 is available to interact with the DP1 or DP2 receptor (see later) or undergo further metabolism to a PGF 2 -like compound.
Prostaglandin F Synthesis
Prostaglandin F 2α is a major urinary COX product. Its synthesis may derive directly from PGH 2 via a PGF synthase or indirectly by metabolism of PGE 2 via a 9-ketoreductase. Another more obscure pathway for PGF formation is by the action of a PGD 2 ketoreductase, yielding a stereoisomer of PGF 2 , 9a,11β-PGF 2 (11-epi-PGF 2α ). This reaction, and conversion of PGD 2 into an apparently biologically active metabolite (9a,11β-PGF 2α ) has been documented in vivo . Interestingly this isomer can also ligate and activate the FP receptor. The physiologically relevant enzymes responsible for renal PGF 2α formation remain incompletely characterized.
Prostaglandin 9-Ketoreductase
Physiologically relevant transformations of COX products occur in the kidney via a reduced nicotinamide adenine dinucleotide phosphate (NADPH)–dependent 9-ketoreductase, which converts PGE 2 into PGF 2α . This enzymatic activity is typically cytosolic and may be detected in homogenates from the renal cortex, medulla, or papilla. The activity appears to be particularly robust in suspensions from the TALH. Renal PGE 2 9-ketoreductase also exhibits 20α- hydroxysteroid reductase activity that could affect steroid metabolism. This enzyme appears to be a member of the aldo-keto reductase family 1C.
Interestingly, some studies have suggested that activity of a 9-ketoreductase may be modulated by salt intake and Ang II type 2 (AT 2 ) receptor activation and may play an important role in hypertension. Mice deficient in the AT 2 receptor exhibit salt-sensitive hypertension, increased PGE 2 production, and reduced production of PGF 2α , consistent with reduced 9-ketoreductase activity. Other studies have suggested that dietary potassium intake may also enhance the activity of conversion from PGE 2 to PGF 2α . The intrarenal sites of expression of this enzymatic activity remain to be characterized.
Prostaglandin E Synthases
PGE 2 is the other major product of COX-initiated arachidonic acid metabolism in the kidney and is synthesized at high rates along the nephron, particularly in the collecting duct. Two membrane-associated PGE 2 synthases have been identified, 33-kDa and 16-kDa membrane-associated enzymes. The initial report describing the cloning of a glutathione-dependent microsomal enzyme (the 16-kDa form) that specifically converts PGH 2 to PGE 2 showed that mRNA for this enzyme is highly expressed in reproductive tissues, as well as in the kidney. Genetic disruption confirms that microsomal PGE synthase 1 (mPGES-1) −/− mice exhibit a marked reduction in inflammatory responses compared with mPGES-1 +/+ mice and indicates that mPGES-1 is also critical for the induction of inflammatory fever.
Intrarenal expression of mPGES1 has been demonstrated and mapped to the collecting duct, with lower expression in the medullary interstitial cells and macula densa (see Figure 14.3 ). Thus, in the kidney, this isoform co-localizes with COX-1 and COX-2. In contrast, in inflammatory cells, this PGE synthase is co-induced with COX-2 and appears to be functionally coupled to it. Notably, the kidneys of mPGES-1 −/− mice are normal and do not exhibit the renal dysgenesis observed in COX-2 −/− mice, nor do these mice exhibit perinatal death from patent ductus arteriosus observed with the prostaglandin EP4 receptor knockout mouse.
Another membrane-associated PGE synthase with a relative mass of about 33 kDa was purified from heart. The recombinant enzyme was activated by several sulfhydryl (SH)-reducing reagents, including dithiothreitol, glutathione (GSH), and β-mercaptoethanol. Moreover, the mRNA distribution was high in the heart and brain and was also expressed in the kidney, but the mRNA was not expressed in the seminal vesicles. The intrarenal distribution of this enzyme is, at present, uncharacterized.
Other cytosolic proteins exhibit lower PGE synthase activity, including a 23-kDa glutathione S-transferase (GST) requiring cytoplasmic PGE synthase that is expressed in the kidney and lower genitourinary tract. Some evidence has suggested that this isozyme may constitutively couple to COX-1 in inflammatory cells. In addition, several cytosolic glutathione S-transferases have the capability to convert PGH 2 to PGE 2 ; however, their physiologic role in this process remains uncertain
Prostanoid Receptors
See Figures 14-10 and 14-11 .


TP Receptors
The TP receptor was originally purified by chromatography using a high-affinity ligand to capture the receptor. This was the first eicosanoid receptor cloned and is a G protein–coupled transmembrane receptor capable of activating a calcium-coupled signaling mechanism ( Figure 14.12 ). The cloning of other prostanoid receptors was achieved by finding cDNAs homologous to this TP receptor cDNA. Two alternatively spliced variants of the human thromboxane receptor have been described that differ in their C-terminal tail distal to Arg. Similar patterns of alternative splicing have been described for the EP3 and FP receptors. Heterologous cAMP-mediated signaling of the thromboxane receptor may occur via its heterodimerization with the prostacyclin (IP) receptor.

The endoperoxide PGH 2 or its metabolite, TXA 2 , can activate the TP receptor. Competition radioligand binding studies have demonstrated a rank order of potency on the human platelet TP receptor of the ligands I-BOP, S145 > SQ29548 > STA 2 > U-46619. Whereas I-BOP, STA 2 , and U-46619 are agonists, SQ29548 and S145 are high-affinity TP receptor antagonists. Studies have suggested that the TP receptor may mediate some of the biologic effects of the nonenzymatically derived isoprostanes, including modulation of tubuloglomerular feedback. This latter finding may have significance in pathophysiologic conditions associated with increased oxidative stress. Signal transduction studies have shown that the TP receptor activates phosphatidylinositol bisphosphate (PIP 2 ) hydrolysis–dependent Ca 2+ influx . Northern blot analysis of mouse tissues has revealed that the highest level of TP mRNA expression is in the thymus, followed by the spleen, lung, and kidney, with lower levels of expression in the heart, uterus, and brain.
Thromboxane is a potent modulator of platelet shape change and aggregation, as well as smooth muscle contraction and proliferation. Moreover, a point mutation (Arg to Leu) in the first cytoplasmic loop of the TXA 2 receptor was identified in a dominantly inherited bleeding disorder in humans, characterized by a defective platelet response to TXA 2 . Targeted gene disruption of the murine TP receptor also resulted in prolonged bleeding times and reduction in collagen-stimulated platelet aggregation ( Figure 14.13 ). Conversely, overexpression of the TP receptor in vascular tissue increases the severity of vascular pathology following injury. Increased thromboxane synthesis has been linked to cardiovascular diseases, including acute myocardial ischemia, heart failure, and inflammatory renal diseases.

In the kidney, TP receptor mRNA has been reported in glomeruli and vasculature. Radioligand autoradiography using 125 I-BOP has suggested a similar distribution of binding sites in the mouse renal cortex, but additional renal medullary binding sites were observed. These medullary TXA 2 binding sites are absent following disruption of the TP receptor gene, suggesting that they also represent authentic TP receptors. Glomerular TP receptors may participate in potent vasoconstrictor effects of TXA 2 analogs on the glomerular microcirculation associated with a reduced GFR. Mesangial TP receptors coupled to phosphatidylinositol hydrolysis, protein kinase C activation, and glomerular mesangial cell contraction may contribute to these effects.
An important role for TP receptors in regulating renal hemodynamics and systemic blood pressure has also been suggested. Administration of a TP receptor antagonist reduces blood pressure in spontaneously hypertensive rats (SHRs) and in angiotensin-dependent hypertension. The TP receptor also appears to modulate renal blood flow in Ang II–dependent hypertension and in endotoxemia-induced renal failure. Modulation of renal TP receptor mRNA expression and function by dietary salt intake has also been reported. These studies also suggested an important role for luminal TP receptors in the distal tubule to enhance glomerular vasoconstriction indirectly via effects on the macula densa TGF. However, other studies revealed no significant difference in TGF between wild-type and TP receptor knockout mice.
A major phenotype of TP receptor disruption in mice and humans appears to be reduced platelet aggregation and prolonged bleeding time. Thromboxane may also modulate the glomerular fibrinolytic system by increasing the production of an inhibitor of plasminogen activator (PAI-1) in mesangial cells. Although a specific renal phenotype in the TP receptor knockout mouse has not yet been reported, important pathogenic roles for TXA 2 and glomerular TP receptors in mediating renal dysfunction in glomerulonephritis, diabetes mellitus, and sepsis seem likely.
In an Ang II–dependent mouse model of hypertension, deletion of the TP receptor gene ameliorated hypertension and reduced cardiac hypertrophy, but had no effect on proteinuria. In contrast, although blockade of NO synthase in an l -NAME ( N G -nitro- l -arginine methyl ester) model of hypertension, in which deletion of the TP receptor also ameliorated hypertension and did not decrease GFR, it led to an increased worsening of histopathology and significant renal hypertrophy. This suggests that the TP receptor may play a renal protective role in some settings.
IP Receptors
The cDNA for the IP receptor encodes a transmembrane protein of approximately 41 kDa. The IP receptor is selectively activated by the analog cicaprost. Iloprost and carbaprostacyclin potently activate the IP receptor but also activate the EP1 receptor. Most evidence suggests that the PGI 2 receptor signals via stimulation of cAMP generation; however, at 1000-fold higher concentrations, the cloned mouse PGI 2 receptor also signaled via PIP 2 . It remains unclear whether PIP 2 hydrolysis plays any significant role in the physiologic action of PGI 2 .
IP receptor mRNA is highly expressed in the mouse thymus, heart, and spleen and in human kidney, liver, and lung. In situ hybridization shows IP receptor mRNA predominantly in neurons of the dorsal root ganglia and vascular tissue, including the aorta, pulmonary artery, and renal interlobular and glomerular afferent arterioles. The expression of IP receptor mRNA in the dorsal root ganglia is consistent with a role for prostacyclin in pain sensation. Mice with IP receptor gene disruption exhibit a predisposition to arterial thrombosis, diminished pain perception, and inflammatory responses.
PGI 2 has been demonstrated to play an important vasodilator role in the kidney, including in the glomerular microvasculature, as well as regulating renin release. The capacity of PGI 2 and PGE 2 to stimulate cAMP generation in the glomerular microvasculature is distinct and additive, demonstrating that the effects of these two prostanoids are mediated via separate receptors. IP receptor knockout mice also exhibit salt-sensitive hypertension. Prostacyclin is a potent stimulus of renal renin release, and studies using IP −/− mice have confirmed an important role for the IP receptor in the development of renin-dependent hypertension of renal artery stenosis.
Renal epithelial effects of PGI 2 in the thick ascending limb have also been suggested, and IP receptors have been reported in the collecting duct, but the potential expression and role of prostacyclin in these segments are less well established. Of interest, in situ hybridization also demonstrated significant expression of prostacyclin synthase in medullary collecting ducts, consistent with a role for this metabolite in this region of the kidney. Thus, although IP receptors appear to play an important role regulating renin release and as a vasodilator in the kidney, their role in regulating renal epithelial function remains to be firmly established.
DP Receptors
The DP1 receptor has been cloned and, like the IP and EP2/4 receptors, the DP receptor predominantly signals by increasing cAMP generation. The human DP receptor binds PGD 2 with a high-affinity binding of 300 pM and a lower affinity site of 13.4 nM. DP-selective PGD 2 analogs include the agonist BW 245C. DP receptor mRNA is highly expressed in leptomeninges, retina, and ileum but was not detected in the kidney. Northern blot analysis of the human DP receptor demonstrated mRNA expression in the small intestine and retina, whereas in the mouse the DP receptor mRNA was detected in the ileum and lung. PGD 2 has also been shown to affect the sleep-wake cycle, pain sensation, and body temperature. Peripherally, PGD 2 has been shown to mediate vasodilation as well as possibly inhibiting platelet aggregation. Consistent with this latter finding, the DP receptor knockout displayed reduced inflammation in the ovalbumin model of allergic asthma. Development of the antagonist laropiprant was undertaken to inhibit the niacin-induced vasodilation flushing response. Although the kidney appears capable of synthesizing PGD 2 , its role in the kidney remains poorly defined. Intrarenal infusion of PGD 2 resulted in a dose-dependent increase in renal artery flow, urine output, creatinine clearance, and sodium and potassium excretion.
A second G protein–coupled receptor capable of binding and being activated by PGD 2 was cloned as an orphan chemoattractant receptor from eosinophils and T cells (type 2 helper T cell subset) and designated the CRTH2 receptor. This receptor, now referred to as the DP2 receptor, bears no significant sequence homology to the family of prostanoid receptors discussed earlier, and couples to G i /G o inhibition of intracellular cAMP. It binds agonists with an order of potency PGD 2 ≫ PGF 2α , PGE 2 > PGI 2 , TXA 2 . DP2 receptor action is blocked by the antagonist ramatroban, a drug used to treat allergic rhinitis and originally described as a TP receptor antagonist. Deletion of the DP2 receptor gene was protective in a mouse UUO model of fibrosis. The recognition of this molecularly unrelated receptor allows for the possibility of the existence of a distinct and new family of prostanoid-activated membrane receptors.
FP Receptors
The cDNA encoding the PGF 2α receptor (FP receptor) was cloned from a human kidney cDNA library and encodes a protein of 359 amino acid residues. The bovine and murine FP receptors, cloned from corpora lutea, similarly encode proteins of 362 and 366 amino acid residues, respectively. Transfection of HEK293 cells with the human FP receptor cDNA conferred preferential 3 H-PGF 2α binding with a K D of 4.3 ± 1.0 nM. Selective activation of the FP receptor may be achieved using fluprostenol or latanoprost. 3 H-PGF 2α binding was displaced by a panel of ligands with a rank order potency of PGF 2α = fluprostenol > PGD 2 > PGE 2 > U46619 > iloprost. When expressed in oocytes, PGF 2α or fluprostenol induced a Ca 2+ -dependent Cl − current. Increased cell calcium has also been observed in fibroblasts expressing an endogenous FP receptor. Other studies have suggested that FP receptors may also activate protein kinase C (PKC)–dependent and Rho -mediated/PKC–independent signaling pathways. An alternatively spliced isoform with a shorter C-terminal tail has been identified that appears to signal via a similar manner as the originally described FP receptor, and other studies have suggested that these two isoforms may exhibit differential desensitization and may also activate a glycogen synthase kinase/β-catenin–coupled signaling pathway.
Tissue distribution of FP receptor mRNA shows highest expression in ovarian corpus luteum followed by kidney, with lower expression in the lung, stomach, and heart. Expression of the FP receptor in corpora lutea is critical for normal birth, and homozygous disruption of the mouse FP receptor gene results in failure of parturition in females, apparently due to failure of the normal preterm decline in progesterone levels. PGF 2α is a potent constrictor of smooth muscle in the uterus, bronchi, and blood vessels; however, an endothelial FP receptor may also play a dilator role. The FP receptor is also highly expressed in skin, where it may play an important role in carcinogenesis. A clinically important role for the FP receptor in the eye has been demonstrated to increase uveoscleral outflow and reduce ocular pressure. The FP–selective agonist latanoprost has been used clinically as an effective treatment for glaucoma.
The role of FP receptors in regulating renal function is only partially defined. FP receptor expression has been mapped to the CCD in mouse and rabbit kidney. FP receptor activation in the collecting duct inhibits vasopressin-stimulated water absorption via a pertussis toxin–sensitive (presumably G i ) dependent mechanism. Although PGF 2α increases cell Ca 2+ in cortical collecting duct, the FP–selective agonists latanoprost and fluprostenol did not increase calcium. Because PGF 2α can also bind to EP1 and EP3 receptors, these data suggest that the calcium increase activated by PGF 2α in the collecting duct may be mediated via an EP receptor. PGF 2α also increases Ca 2+ in cultured glomerular mesangial cells and podocytes, suggesting that an FP receptor may modulate glomerular contraction. In contrast to these findings, glomerular FP receptors at the molecular level have not been demonstrated. Other vascular effects of PGF 2α have been described, including selective modulation of renal production of PGF 2α by sodium or potassium loading and AT 2 receptor activation.
Some studies have uncovered a role for the FP receptor in regulating renin expression. Interestingly, FP agonists increased renin mRNA expression in the JGA in a dose-dependent manner but, unlike IP receptor agonists, did not increase intracellular cAMP. Deletion of the FP receptor resulted in decreased renin levels and decreased systemic blood pressure. These data suggest that FP receptor blockade may be a novel target for the treatment of hypertension.
Multiple EP Receptors
Four EP receptor subtypes have been identified. Although these four receptors uniformly bind PGE 2 with a higher affinity than other endogenous prostanoids, the amino acid homology of each is more closely related to other prostanoid receptors that signal through similar mechanisms. Thus, the relaxant/cAMP–coupled EP2 receptor is more closely related to other relaxant prostanoid receptors, such as the IP and DP receptors, whereas the constrictor/Ca 2+ –coupled EP1 receptor is more closely related to the other Ca 2+ –coupled prostanoid receptors (e.g., TP and FP receptors). These receptors may also be selectively activated or antagonized by different analogs. EP receptor subtypes also exhibit differential expression along the nephron, suggesting distinct functional consequences of activating each EP receptor subtype in the kidney.
EP1 Receptors
The human EP1 receptor cDNA encodes a 402–amino acid polypeptide that signals via inositol 1,4,5-trisphosphate (IP 3 ) generation and increased cell Ca 2+ with IP 3 generation. Studies of EP1 receptors may use one of several relatively selective antagonists, including ONO-871, SC19220, and SC53122. EP1 receptor mRNA has widespread expression, presumably from its vascular expression, and is expressed in the kidney much more than in the gastric muscularis mucosae and more than in the adrenal gland. Renal EP1 mRNA expression determined by in situ hybridization is expressed primarily in the collecting duct and increases from the cortex to the papillae. Activation of the EP1 receptor increases intracellular calcium and inhibits Na + and water reabsorption in the collecting duct, suggesting that renal EP1 receptor activation might contribute to the natriuretic and diuretic effects of PGE 2 .
Hemodynamic microvascular effects of EP1 receptors have also been reported. The EP1 receptor was originally described as a smooth muscle constrictor. One report suggested that the EP1 receptor may also be present in cultured glomerular mesangial cells, where it could play a role as a vasoconstrictor and stimulus for mesangial cell proliferation. Although a constrictor PGE 2 effect has been reported in the afferent arteriole of rat, apparently produced by EP1 receptor activation, there does not appear to be very high expression of the EP1 receptor mRNA in preglomerular vasculature or other arterial resistance vessels in mice or rabbits. Other reports have suggested that EP1 receptor knockout mice exhibit hypotension and hyperreninemia, supporting a role for this receptor in maintaining blood pressure.
EP2 Receptors
Two cAMP-stimulating EP receptors, designated EP2 and EP4, have been identified. The EP2 receptor can be pharmacologically distinguished from the EP4 receptor by its sensitivity to butaprost. In the literature prior to 1995, the cloned EP4 receptor was designated the EP2 receptor, but then a butaprost-sensitive EP receptor was cloned ; the original receptor was reclassified as the EP4 receptor and the newer, butaprost-sensitive protein was designated the EP2 receptor. A pharmacologically defined EP2 receptor has now also been cloned for the mouse, rat, rabbit, dog, and cow. The human EP2 receptor cDNA encodes a 358–amino acid polypeptide, which signals through increased cAMP. The EP2 receptor may also be distinguished from the EP4 receptor, the other major relaxant EP receptor, by its relative insensitivity to the EP4 agonist PGE 1 -OH and insensitivity to the weak EP4 antagonist AH23848 and high- affinity EP4 antagonists ONO-AE3-208 and L-161982. Recently, two EP2 antagonists have been described, PF-04418948 and TG4-155, which should greatly facilitate the characterization of EP2 versus EP4 effects in vivo.
The precise distribution of the EP2 receptor mRNA has been partially characterized. This reveals a major mRNA species of about 3.1 kb that is most abundant in the uterus, lung, and spleen, exhibiting only low levels of expression in the kidney. Studies using polymerase chain reaction (PCR) analysis across a range of tissue have demonstrated highest expression in the bone marrow more than in the ovary and more than in the lung, consistent with these earlier findings. EP2 mRNA is expressed at much lower levels than EP4 mRNA in most tissues. There is scant evidence to suggest segmental distribution of the EP2 receptor along the nephron. Interestingly, it is expressed in cultured renal interstitial cells, supporting the possibility that the EP2 receptor is predominantly expressed in this portion of the nephron. Studies in knockout mice have demonstrated a critical role for the EP2 receptor in ovulation and fertilization, and these studies have also suggested a potential role for the EP2 receptor in salt-sensitive hypertension. This latter finding supports an important role for the EP2 receptor in protecting systemic blood pressure, perhaps via its vasodilator effect or effects on renal salt excretion. Evidence for the latter role has been revealed in studies demonstrating that a high-salt diet increases PGE 2 production, and infusion of EP-selective agonists identified the EP2 receptor as mediating PGE 2 -evoked natriuresis. Moreover, deletion of the EP2 receptor ablated the natriuretic effect of PGE 2 .
EP3 Receptors
The EP3 receptor generally acts as a constrictor of smooth muscle. Nuclease protection and Northern blot analysis demonstrate relatively high levels of EP3 receptor expression in several tissues, including kidney, uterus, adrenal, and stomach, with riboprobes hybridizing to major mRNA species at approximately 2.4 and approximately 7.0 kb. A metabolic pattern of expression was found by PCR testing, with high levels of expression in the pancreas and white and brown fat tissue in addition to expression in the kidney. This receptor is unique in that there are multiple (more than eight) alternatively spliced variants differing only in their C-terminal cytoplasmic tails. The EP3 splice variants bind PGE 2 , and the EP3 agonists MB28767 and sulprostone with similar affinity. Also, although they exhibit common inhibition of cAMP generation via a pertussis toxin–sensitive G i -coupled mechanism, the tails may recruit different signaling pathways, including Ca 2+ -dependent signaling and the small G protein Rho. Recently, a Ptx-insensitive pathway for the inhibition of cAMP generation via guanine nucleotide-binding protein G(z) subunit alpha has also been described. Differences in agonist-independent activity have been observed for several of the splice variants, suggesting that they may play a role in constitutive regulation of cellular events. The physiologic roles of these different C-terminal splice variants and sites of expression within the kidney remain uncertain.
In situ hybridization has demonstrated that EP3 receptor mRNA is abundant in the TAL and collecting duct (CD). This distribution has been confirmed by reverse transcriptase PCR (RT-PCR) testing on microdissected rat and mouse collecting ducts and corresponds to the major binding sites for radioactive PGE 2 in the kidney. An important role for a G i -coupled PGE receptor in regulating water and salt transport along the nephron has been recognized for many years. PGE 2 directly inhibits salt and water absorption in microperfused TALs and CDs. PGE 2 directly inhibits Cl − absorption in the mouse or rabbit medullary TAL from luminal or basolateral surfaces. PGE 2 also inhibits hormone-stimulated cAMP generation in the TAL. Good and George have demonstrated that PGE 2 modulates ion transport in the rat TAL by a pertussis toxin–sensitive mechanism. Interestingly, these effects also appear to involve PKC activation, possibly reflecting activation of a novel EP3 receptor signaling pathway, possibly corresponding to alternative signaling pathways, as described earlier. Taken together, these data support a role for the EP3 receptor in regulating transport in both the CCD and TAL.
Blockade of endogenous PGE 2 synthesis by NSAIDs enhances urinary concentration. It is likely that PGE 2 -mediated antagonism of vasopressin-stimulated salt absorption in the TAL and water absorption in the CCD contributes to its diuretic effect. In the in vitro microperfused collecting duct, PGE 2 inhibits vasopressin-stimulated osmotic water absorption and vasopressin-stimulated cAMP generation. Furthermore, PGE 2 inhibition of water absorption and cAMP generation are both blocked by pertussis toxin, suggesting effects mediated by the inhibitory G protein, G i . When administered in the absence of vasopressin, PGE 2 actually stimulates water absorption in the CCD from the luminal or basolateral side. These stimulatory effects of PGE 2 on transport in the CCD appear to be related to activation of the EP4 receptor. Despite the presence of this absorption-enhancing EP receptor, in vivo studies have suggested that in the presence of vasopressin, the predominant effects of endogenous PGE 2 on water transport are diuretic. Based on the preceding functional considerations, one would expect EP3 −/− mice to exhibit inappropriately enhanced urinary concentration. Surprisingly, EP3 −/− mice exhibited a comparable urinary concentration following desmopressin (DDAVP) administration, similar 24-hour water intake, and similar maximal and minimal urinary osmolality. The only clear difference was that in mice allowed free access to water, indomethacin increased urinary osmolality in normal mice but not in the knockout animals. These findings raise the possibility that some of the renal actions of PGE 2 normally mediated by the EP3 receptor have been co-opted by other receptors (e.g., EP1 or FP receptor) in the EP3 knockout mouse. This remains to be formally tested.
The function of EP3 receptor activation in animal physiology has been significantly advanced by the availability of mice with targeted disruption of this gene. Mice with targeted deletion of the EP3 receptor exhibit an impaired febrile response, suggesting that EP3 receptor antagonists could be effective antipyretic agents. Other studies have suggested that the EP3 receptor plays an important vasopressor role in the peripheral circulation of mice. In the intrarenal circulation, PGE 2 has variable effects, acting as a vasoconstrictor in the larger, proximal portion of the intralobular arteries and changing to a vasodilator effect in the smaller, distal intralobular arteries and afferent arterioles.
EP4 Receptor
Although the EP4 receptor signals through increased cAMP, like the EP2 receptor, it has been appreciated to signal though a number of other pathways as well. These include arrestin-mediated signaling, phosphatidylinositol-3-kinase, signaling β-catenin and G i coupling. The human EP4 receptor cDNA encodes a 488–amino acid polypeptide with a predicted molecular mass of about 53 kDa. Note that care must be taken in reviewing the literature prior to 1995, when this receptor was generally referred to as the EP2 receptor. In addition to the human receptor, EP4 receptors for the mouse, rat, rabbit, and dog have been cloned. EP4 receptors can be pharmacologically distinguished from EP1 and EP3 receptors by insensitivity to sulprostone and from EP2 receptors by insensitivity to butaprost and relatively selective activation by PGE 1 -OH. EP4–selective agonists (ONO-AE1-329, ONO-4819) and antagonists (ONO-AE3-208, L-161,982) have been generated and used to investigate the role of EP4 in vivo. Activation of the EP4 receptor was able to ameliorate the phenotype of a mouse model of nephrogenic diabetes insipidus.
EP4 receptor mRNA is highly expressed relative to the EP2 receptor and is widely distributed, with a major species of approximately 3.8 kb detected by Northern blot analysis in the thymus, ileum, lung, spleen, adrenal, and kidney. Dominant vasodilator effects of EP4 receptor activation have been described in venous and arterial beds. A critical role for the EP4 receptor in regulating the perinatal closure of the pulmonary ductus arteriosus has also been suggested by studies of mice with targeted disruption of the EP4 receptor gene. On a 129- strain background, EP4 −/− mice had an almost 100% perinatal mortality due to persistent patent ductus arteriosus. Interestingly, when bred on a mixed genetic background, only 80% of EP4 −/− mice died, whereas about 21% underwent closure of the ductus and survived. Preliminary studies in these survivors have supported an important role for the EP4 receptor as a systemic vasodepressor ; however, their heterogeneous genetic background complicates the interpretation of these results, because survival may select for modifier genes that not only allow ductus closure, but also alter other hemodynamic responses.
Other roles for the EP4 receptor in controlling blood pressure have been suggested, including the ability to stimulate aldosterone release from zona glomerulosa cells. In the kidney, EP4 receptor mRNA expression is primarily in the glomerulus, where its precise function is uncharacterized but might contribute to regulation of the renal microcirculation and renin release. Studies in mice with genetic deletion of selective prostanoid receptors have indicated that EP4 −/− mice, as well as IP −/− mice to a lesser extent, failed to increase renin production in response to loop diuretic administration, indicating that macula densa–derived PGE 2 increased renin primarily through EP4 activation. This corresponds to studies suggesting that EP4 receptors are expressed in cultured podocytes and JGA cells. PGE 2 may mediate increased podocyte COX-2 expression through EP4-mediated increased cAMP, which activates P38 through a PKA-independent process. Finally, the EP4 receptor in the renal pelvis may participate in the regulation of salt excretion by altering afferent renal nerve output.
Regulation of Renal Function by EP Receptors
PGE 2 exerts myriad effects in the kidney, presumably mediated by EP receptors. PGE 2 not only dilates the glomerular microcirculation and vasa recta, supplying the renal medulla, but also modulates salt and water transport in the distal tubule (see Figure 14.5 ). The maintenance of normal renal function during physiologic stress is particularly dependent on endogenous PG synthesis. In this setting, the vasoconstrictor effects of Ang II, catecholamines, and vasopressin are more effectively buffered by prostaglandins in the kidney than in other vascular beds, preserving normal renal blood flow, GFR, and salt excretion. Administration of COX-inhibiting NSAIDs in the setting of volume depletion interferes with these dilator effects and may result in a catastrophic decline in the GFR, resulting in overt renal failure.
Other evidence points to vasoconstrictor and prohypertensive effects of endogenous PGE 2 . PGE 2 stimulates renin release from the JGA, leading to a subsequent increase in the vasoconstrictor Ang II. In conscious dogs, chronic intrarenal PGE 2 infusion increases renal renin secretion, resulting in hypertension. Treatment of salt-depleted rats with indomethacin not only decreases plasma renin activity, but also reduces blood pressure, suggesting that PGs support blood pressure during salt depletion via their capacity to increase renin. Direct vasoconstrictor effects of PGE 2 on vasculature have also been observed. It is conceivable that these latter effects might predominate in circumstances in which the kidney is exposed to excessively high perfusion pressures. Thus, depending on the setting, the primary effect of PGE 2 may be to increase or decrease vascular tone, effects that appear to be mediated by distinct EP receptors.
Renal Cortical Hemodynamics
The expression of the EP4 receptor in the glomerulus suggests that it may play an important role in regulating renal hemodynamics. PGs regulate the renal cortical microcirculation and, as noted, both glomerular constrictor and dilator effects of prostaglandins have been observed. In the setting of volume depletion, endogenous PGE 2 helps maintain the GFR by dilating the afferent arteriole. Some data have suggested roles for EP and IP receptors coupled to increased cAMP generation in mediating vasodilator effects in the preglomerular circulation. PGE 2 exerts a dilator effect on the afferent arteriole but not the efferent arteriole, consistent with the presence of an EP2 or EP4 receptor in the preglomerular microcirculation.
Renin Release
Other data have suggested that the EP4 receptor may also stimulate renin release. Soon after the introduction of NSAIDs, it was recognized that endogenous PGs play an important role in stimulating renin release. Treatment of salt-depleted rats with indomethacin not only decreases plasma renin activity, but also causes blood pressure to fall, suggesting that PGs support blood pressure during salt depletion via their capacity to increase renin. Prostanoids also play a central role in the pathogenesis of renovascular hypertension, and administration of NSAIDs lowers blood pressure in animals and humans with renal artery stenosis. PGE 2 induces renin release in isolated preglomerular juxtaglomerular apparatus cells. Like the effect of β-adrenergic agents, this effect appears to be through a cAMP-coupled response, supporting a role for an EP4 or EP2 receptor. EP4 receptor mRNA has been detected in microdissected JGAs, supporting the possibility that renal EP4 receptor activation contributes to enhanced renin release. Finally, regulation of plasma renin activity and intrarenal renin mRNA does not appear to be different in wild-type and EP2 knockout mice, arguing against a major role for the EP2 receptor in regulating renin release. Conversely, one report has suggested that EP3 receptor mRNA is localized to the macula densa, suggesting that this cAMP-inhibiting receptor may also contribute to the control of renin release.
Renal Microcirculation
The EP2 receptor also appears to play an important role in regulating afferent arteriolar tone. In the setting of systemic hypertension, the normal response of the kidney is to increase salt excretion, thereby mitigating the increase in blood pressure. This so-called pressure natriuresis plays a key role in the ability of the kidney to protect against hypertension. Increased blood pressure is accompanied by increased renal perfusion pressure and enhanced urinary PGE 2 excretion. Inhibition of PG synthesis markedly blunts (although it does not eliminate) pressure natriuresis. The mechanism whereby PGE 2 contributes to pressure natriuresis may involve changes in resistance of the renal medullary microcirculation. PGE 2 directly dilates the descending vasa recta, and increased medullary blood flow may contribute to the increased interstitial pressure observed as renal perfusion pressure increases, leading to enhanced salt excretion. The identity of the dilator PGE 2 receptor controlling the contractile properties of the descending vasa recta remains uncertain, but EP2 or EP2 receptors seem likely candidates. Results of studies demonstrating salt-sensitive hypertension in mice with targeted disruption of the EP2 receptor have suggested that the EP2 receptor facilitates the ability of the kidney to increase sodium excretion, thereby protecting systemic blood pressure from a high-salt diet. Given its defined role in vascular smooth muscle, these effects of the EP2 receptor disruption seem more likely to relate to its effects on renal vascular tone. In particular, loss of a vasodilator effect in the renal medulla might modify pressure natriuresis and could contribute to hypertension in EP2 knockout mice. Nonetheless, a role for the EP2 or EP4 receptor in regulating renal medullary blood flow remains to be established. In conclusion, direct vasomotor effects of EP4 receptors, as well as effects on renin release, may play critical roles in regulating systemic blood pressure and renal hemodynamics.
Effects of COX-1 and COX-2 Metabolites on Salt and Water Transport
COX-1 and COX-2 metabolites of arachidonate have important direct epithelial effects on salt and water transport along the nephron. Thus, functional effects can be observed that are thought to be independent of any hemodynamic changes produced by these compounds. Because biologically active arachidonic acid metabolites are rapidly metabolized, they act predominantly in an autocrine or paracrine fashion and, thus, their locus of action will be quite close to their point of generation. Thus, one can expect that direct epithelial effects of these compounds will result when they are produced by the tubule cells themselves or the neighboring interstitial cells, and the tubules possess an appropriate receptor for the ligand.
Proximal Tubule
Neither the proximal convoluted tubule nor the proximal straight tubule appears to produce amounts of biologically active COX metabolites of arachidonic acid. As will be discussed in a subsequent section, the dominant arachidonate metabolites produced by proximal convoluted and straight tubules are metabolites of the cytochrome P450 pathway.
Early whole-animal studies suggested that PGE 2 might have an action in the proximal tubule because of its effects on urinary phosphate excretion. PGE 2 blocked the phosphaturic action of calcitonin infusion in thyroparathyroidectomized rats. Nevertheless, studies using in vitro perfused proximal tubules failed to show an effect of PGE 2 on sodium chloride or phosphate transport in the proximal convoluted tubule. More recent studies have suggested that PGE 2 may play a key role in the phosphaturic action of fibroblast growth factor-23 because phosphaturia in hyp mice with X-linked hyperphosphaturia is associated with markedly increased urine PGE 2 excretion, and phosphaturia was normalized by indomethacin. Nevertheless, there are very little data on the actions of other COX metabolites in proximal tubules and scant molecular evidence for the expression of classic G protein–coupled prostaglandin receptors in this segment of the nephron.
Loop of Henle
The nephron segments making up the loop of Henle also display limited metabolism of exogenous arachidonic acid through the COX pathway although, given the realization that COX-2 is expressed in this segment, it is of note that PGE 2 was uniformly greater in the cortical segment than the medullary thick ascending limb. The TAL has been shown to exhibit high- density PGE 2 receptors. Studies have also demonstrated high expression levels of mRNA for the EP3 receptor in medullary TAL of both rabbit and rat (see earlier, “ EP3 Receptors ”). Subsequent to the demonstration that PGE 2 inhibits sodium chloride absorption in the medullary TAL of the rabbit perfused in vitro, it was shown that PGE 2 blocks vasopressin (AVP) but not cAMP-stimulated sodium chloride absorption in the medullary TAL of the mouse. It is likely that the mechanism involves activation of G i and inhibition of adenyl cyclase by PGE 2 , possibly via the EP3 receptors expressed in this segment.
Collecting Duct System
In vitro perfusion studies of rabbit cortical collecting tubule have demonstrated that PGE 2 directly inhibits sodium transport in the collecting duct when applied to the basolateral surface of this nephron segment. It is now apparent that PGE 2 uses multiple signal transduction pathways in the cortical collecting duct, including those that modulate intracellular cAMP levels and Ca 2+ . PGE 2 can stimulate or suppress cAMP accumulation. The latter may also involve stimulation of phosphodiesterase. Although modulation of cAMP levels appears to play an important role in PGE 2 effects on water transport in the CCD (see following section), it is less clear that PGE 2 affects sodium transport via modulation of cAMP levels. PGE 2 has been shown to increase cell calcium, possibly coupled with PKC activation, in in vitro perfused cortical collecting ducts. This effect may be mediated by the EP1 receptor subtype coupled to phosphatidylinositol hydrolysis.
Water Transport
AVP-regulated water transport in the collecting duct is markedly influenced by COX products, especially prostaglandins. When COX inhibitors are administered to humans, rat, or dog, the antidiuretic action of AVP is markedly augmented. Because vasopressin also stimulates endogenous PGE 2 production by the collecting duct, these results suggest that PGE 2 participates in a negative feedback loop, whereby endogenous PGE 2 production dampens the action of AVP. In agreement with this model, the early classic studies of Grantham and Orloff directly demonstrated that PGE 1 blunted the water permeability response of the CCD to vasopressin. In these early studies, the action of PGE 1 appeared to be at a pre-cAMP step. Interestingly, when administered by itself, PGE 1 modestly augmented basal water permeability. These earlier studies have been confirmed with respect to PGE 2 . PGE 2 also stimulates basal hydraulic conductivity and suppresses the hydraulic conductivity response to AVP in the rabbit cortical collecting duct. Inhibition of AVP-stimulated cAMP generation and water permeability appears to be mediated by the EP1 and EP3 receptors, whereas the increase in basal water permeability may be mediated by the EP4 receptor. These data are evidence of consistent functional redundancy between the EP1 and EP3 with respect to their effects on AVP-stimulated water absorption in the collecting duct.
Metabolism of Prostaglandins
15-Ketodehydrogenase
The half-life of prostaglandins is 3 to 5 minutes and that of TXA 2 is approximately 30 seconds. Elimination of PGE 2 , PGF 2α , and PGI 2 proceeds through enzymatic and nonenzymatic pathways, whereas that of TXA 2 is nonenzymatic. The end products of all these degradative reactions generally possess minimal biologic activity, although this is not uniformly true (see below). The principal enzyme involved in the transformation of PGE 2 , PGI 2 , and PGF 2α is 15-hydroxyprostaglandin dehydrogenase (15-PGDH), which converts the 15 alcohol group to a ketone.
15-PGDH is an oxidized nicotinamide adenine dinucleotide–oxidized nicotinamide adenine dinucleotide phosphate (NAD + /NADP + )–dependent enzyme that is 30 to 49 times more active in the kidney of the young rat (3 weeks of age) than in the adult. Its K m for PGE 2 is 8.4 µmol/L and 22.6 µmol/L for PGF 2α . It is mainly localized in cortical and juxtamedullary zones, with little activity detected in papillary slices. At baseline, it is found in the proximal tubule, TAL, and CD. However, it was present in the macula densa in COX-2 knockout mice and in the presence of high-salt diet and, in cultured macula densa cells, COX inhibition increased expression. Disruption of the 15-PGDH gene in mice results in persistent patent ductus arteriosus (PDA), thought to be a result of failure of circulating PGE 2 levels to fall in the immediate peripartum period. Thus, administration of COX-inhibiting NSAIDs rescues the knockout mice by decreasing PGs and allowing the animals to survive.
Subsequent catalysis of 15-hydroxy products by a δ-13 reductase leads to the formation of 13,14-dihydro compounds. PGI 2 and TXA 2 undergo rapid degradation to 6-keto-PGF 1α and TXB 2 , respectively. These stable metabolites are usually measured and their rates of formation taken as representative of those of the parent molecules.
ω/ω-1 Hydroxylation of Prostaglandins
Both PGA 2 and PGE 2 have been shown to undergo hydroxylation of their terminal or subterminal carbons by a cytochrome P450–dependent mechanism. This reaction may be mediated by CYP4A family members or a CYP4F enzyme. CYP4A and CYP4F members have been mapped along the nephron. Some of these derivatives have been shown to exhibit biologic activity.
Cyclopentenone Prostaglandins
The cyclopentenone prostaglandins include PGA 2 , a PGE 2 derivative, and PGJ 2 , a derivative of PGD 2 . Although it remains uncertain whether these compounds are actually produced in vivo, this possibility has received increasing attention because some cyclopentenone prostanoids have been shown to be activating ligands for nuclear transcription factors, including peroxisome proliferator–activated receptors δ and γ (PPARδ and PPARγ). The realization that the antidiabetic thiazolidinedione drugs act through PPARγ to exert their antihyperglycemic and insulin-sensitizing effects has generated intense interest in the possibility that the cyclopentenone PGs might serve as the endogenous ligands for these receptors. Interestingly, DP2, unlike DP1 or other members of the prostaglandin GPCR family, binds and is activated by PGD 2 metabolites such as 15-deoxy-Δ12,14-PGJ 2 , which acts at nanomolar concentrations. An alternative biologic activity of these compounds has been recognized in their capacity to modify thiol groups covalently, forming adducts with cysteine of several intracellular proteins, including thioredoxin 1, vimentin, actin, and tubulin. Studies regarding the biologic activity of cyclopentenone prostanoids abound and the reader is referred to several excellent sources in the literature. Although there is evidence supporting the presence of these compounds in vivo, it remains uncertain whether they can form enzymatically or are an unstable spontaneous dehydration product of the E and D ring prostaglandins.
Nonenzymatic Metabolism of Arachidonic Acid
It has long been recognized that oxidant injury can result in peroxidation of lipids. In 1990, Morrow and coworkers reported that a series of prostaglandin-like compounds could be produced by free radical catalyzed peroxidation of arachidonic acid that is independent of COX activity. These compounds, termed isoprostanes, have been increasingly used as sensitive markers of oxidant injury in vitro and in vivo. In addition, at least two of these compounds, 8-iso-PGF 2α (15-F 2 -isoprostane) and 8-iso-PGE 2 (15-E 2 -isoprostane) are potent vasoconstrictors when administered exogenously. 8-Iso-PGF 2α has been shown to constrict the renal microvasculature and decrease GFR, an effect that is prevented by thromboxane receptor antagonism. However, the role of endogenous isoprostanes as mediators of biologic responses remains unclear.
Prostaglandin Transport and Urinary Excretion
It is notable that most of the prostaglandin synthetic enzymes have been localized to the intracellular compartment, yet extracellular prostaglandins are potent autocoids and paracrine factors. Thus, prostanoids must be transported extracellularly to achieve efficient metabolism and termination of their signaling. Similarly, enzymes that metabolize PGE 2 to inactive compounds are also intracellular, requiring uptake of the PG for its metabolic inactivation. The molecular basis of these extrusion and uptake processes are now being defined.
As a fatty acid, prostaglandins may be classified as an organic anion at physiologic pH. Early microperfusion studies documented that basolateral PGE 2 could be taken up into proximal tubule cells and actively secreted into the lumen. Furthermore this process could be inhibited by a variety of inhibitors of organic anion transport, including p -aminohippurate (PAH), probenecid, and indomethacin. Studies of basolateral renal membrane vesicles also supported the notion that this transport process occurs via an electroneutral anion exchanger. These studies are of note because renal prostaglandins enter the urine in Henle’s loop, and late proximal tubule secretion could provide an important entry mechanism.
A molecule that mediates PGE 2 uptake in exchange for lactate has been cloned and termed prostaglandin transporter (PGT). PGT is a member of the SLC21/SLCO organic anion transporting family, and its cDNA encodes a transmembrane protein of 100 amino acids that exhibits broad tissue distribution (heart, placenta, brain, lung, liver, skeletal muscle, pancreas, kidney, spleen, prostate, ovary, small intestine, and colon). Immunocytochemical studies of PGT expression in rat kidneys have suggested expression primarily in glomerular endothelial and mesangial cells, arteriolar endothelial and muscularis cells, principal cells of the CD, medullary interstitial cells, medullary vasa recta endothelia, and papillary surface epithelium. PGT appears to mediate PGE 2 uptake rather than release, allowing target cells to metabolize this molecule and terminate signaling. PGT expression is decreased with low salt and increased with high salt in the CD, which may allow regulation of PG excretion by taking up more prostaglandins excreted from luminal surface, the site of the PGT, thereby allowing more accumulation at the basolateral surface.
Other members of the organic cation, anion, and zwitterion transporter family SLC22 have also been shown to transport prostaglandins and have been suggested to mediate prostaglandin excretion into the urine. Specifically, OAT1 and OAT3 are localized on the basolateral proximal tubule membrane, where they likely participate in urinary excretion of PGE 2 . Conversely members of the multidrug resistance protein (MRP) have been shown to transport PGs in an adenosine triphosphate–dependent fashion. MRP2 (also designated ABBC2) is expressed in kidney proximal tubule brush borders and may contribute to the transport (and urinary excretion) of glutathione-conjugated prostaglandins. This transporter has more limited tissue expression, restricted to the kidney, liver, and small intestine, and could contribute not only to renal PAH excretion but also to prostaglandin excretion.
Involvement of Cyclo-Oxygenase Metabolites in Renal Pathophysiology
Experimental and Human Glomerular Injury
Glomerular Inflammatory Injury
COX metabolites have been implicated in functional and structural alterations in glomerular and tubulointerstitial inflammatory diseases. Essential fatty acid deficiency totally prevents the structural and functional consequences of the administration of nephrotoxic serum (NTS) to rats, an experimental model of anti–glomerular basement membrane glomerulonephritis. Changes in arteriolar tone during the course of this inflammatory lesion are mediated principally by locally released COX and LO metabolites of AA.
TXA 2 release appears to play an essential role in mediating the increased renovascular resistance observed during the early phase of this disease. Subsequently, increasing rates of PGE 2 generation may account for the progressive dilation of renal arterioles and increases in renal blood flow at later stages of the disease. Consistent with this hypothesis, TXA 2 antagonism ameliorated the falls in renal blood flow (RBF) and GFR 2 hours post-NTS administration, but not after 24 hours. During the latter, heterologous, phase of NTS, COX metabolites mediate the renal vasodilation and reduction in K f that characterize this phase. The net functional result of COX inhibition during this phase of experimental glomerulonephritis, therefore, would depend on the relative importance of renal perfusion versus the preservation of K f to the maintenance of the GFR. Evidence has also indicated that COX metabolites are mediators of pathologic lesions and the accompanying proteinuria in this model. COX-2 expression in the kidney increases in experimental anti-GBM (glomerular basement membrane) glomerulonephritis and after systemic administration of lipopolysaccharide.
A beneficial effect of fish oil diets (enriched in eicosapentaneoic acid), with an accompanying reduction in the generation of COX products, has been demonstrated on the course of genetic murine lupus (in MRL-lpr mice). In subsequent studies, enhanced renal TXA 2 and PGE 2 generation was demonstrated in this model, as well as in NZB mice, another genetic model of lupus. In addition, studies in humans have demonstrated an inverse relation between TXA 2 biosynthesis and GFR and improvement of renal function following short-term therapy with a thromboxane receptor antagonist in patients with lupus nephritis. Other studies have indicated that in humans, as well as NZB mice, COX-2 expression is upregulated in patients with active lupus nephritis, with co-localization to infiltrating monocytes, suggesting that monocytes infiltrating the glomeruli contribute to the exaggerated local synthesis of TXA 2 . COX-2 inhibition selectively decreased thromboxane production, and chronic treatment of NZB mice with a COX-2 inhibitor and mycophenolate mofetil significantly prolonged survival. Taken together, these results, as well as others from animal and human studies, support a major role for the intrarenal generation of TXA 2 in mediating renal vasoconstriction during inflammatory and lupus-associated glomerular injury. In contrast, an EP4–selective agonist was shown to reduce glomerular injury in a mouse model of anti-GBM disease.
The demonstration of a functionally significant role for COX metabolites in experimental and human inflammatory glomerular injury has raised the question of the cellular sources of these eicosanoids in the glomerulus. In addition to infiltrating inflammatory cells, resident glomerular macrophages, glomerular mesangial cells, and glomerular epithelial cells represent likely sources for eicosanoid generation. In the anti-Thy1.1 model of mesangioproliferative glomerulonephritis, COX-1 staining was transiently increased in diseased glomeruli at day 6 and was localized mainly to proliferating mesangial cells. COX-2 expression in the macula densa region also transiently increased at day 6. Glomerular COX-2 expression in this model has been controversial, with one group reporting increased podocyte COX-2 expression and two other groups reporting minimal, if any, glomerular COX-2 expression. However, it is of interest that selective COX-2 inhibitors have been reported to inhibit glomerular repair in the anti-Thy1.1 model. In both anti-Thy1.1 and anti-GBM models of glomerulonephritis, the nonselective COX inhibitor, indomethacin, increased monocyte chemoattractant protein-1 (MCP-1), suggesting that prostaglandins may repress recruitment of monocytes and macrophages in experimental glomerulonephritis.
A variety of cytokines have been reported to stimulate PGE 2 synthesis and COX-2 expression in cultured mesangial cells. Furthermore, complement components, in particular C5b-9, which are known to be involved in the inflammatory models described above, have been implicated in the stimulation of PGE 2 synthesis in glomerular epithelial cells. Cultured glomerular epithelial cells (GECs) express predominantly COX-1, but exposure to C5b-9 significantly increases COX-2 expression.
Glomerular Noninflammatory Injury
Studies have suggested that prostanoids may also mediate altered renal function and glomerular damage following subtotal renal ablation, and glomerular prostaglandin production may be altered in such conditions. Glomeruli from remnant kidneys, as well as animals fed a high-protein diet, have increased prostanoid production. These studies have suggested an increase in COX enzyme activity per se rather than, or in addition to, increased substrate availability, because increases in prostanoid production were noted when excess exogenous AA was added.
Following subtotal renal ablation, there are selective increases in renal cortical and glomerular COX-2 mRNA and immunoreactive protein expression, without significant alterations in COX-1 expression. This increased COX-2 expression was most prominent in the macula densa and surrounding TAL. In addition, COX-2 immunoreactivity was also present in podocytes of remnant glomeruli, and increased PG production in isolated glomeruli from remnant kidneys was inhibited by a COX-2–selective inhibitor but was not decreased by a COX-1–selective inhibitor. Of interest, in the fawn-hooded rat, which develops spontaneous glomerulosclerosis, there is increased TAL–macula densa COX-2 and neuronal nitric oxide synthase (nNOS) and juxtaglomerular cell renin expression preceding development of sclerotic lesions. Studies have indicated that selective overexpression of COX-2 in podocytes in mice increases sensitivity to the development of glomerulosclerosis, an effect that is mediated by thromboxane receptor activation.
When given 24 hours after subtotal renal ablation, a nonselective NSAID, indomethacin, normalized increases in renal blood flow and single-nephron GFR; similar decreases in hyperfiltration were noted when indomethacin was given acutely to rats 14 days after subtotal nephrectomy although, in this latter study, the increased glomerular capillary pressure (P GC ) was not altered because afferent and efferent arteriolar resistances increased. Previous studies also suggested that nonselective COX inhibitors may acutely decrease hyperfiltration in diabetes and inhibit proteinuria and/or structural injury ; other studies have indicated that selective COX-2 inhibitors will decrease the hyperfiltration seen in experimental diabetes or increased dietary protein. Of note, NSAIDs have also been reported to be effective in reducing proteinuria in patients with refractory nephrotic syndrome. Similarly, selective COX-2 inhibition decreased proteinuria in patients with diabetic or nondiabetic renal disease, without alterations in blood pressure.
The prostanoids involved have not yet been completely characterized, although it is presumed that vasodilatory prostanoids are involved in mediation of the altered renal hemodynamics. Defective autoregulation of renal blood flow due to decreased myogenic tone of the afferent arteriole is seen after subtotal ablation or excessive dietary protein and is corrected by inhibition of COX activity. In these hyperfiltering states, TGF is reset at a higher distal tubular flow rate. Such a resetting dictates that afferent arteriolar vasodilation will be maintained in the presence of increased distal solute delivery. It has previously been shown that alterations in TGF sensitivity after reduction in renal mass are prevented with the nonselective COX inhibitor indomethacin. An important role has been suggested for nNOS, which is localized to the macula densa, in the vasodilatory component of TGF. Of interest, studies by Ichihara and colleagues have determined that this nNOS-mediated vasodilation is inhibited by the selective COX-2 inhibitor, NS398, suggesting that COX-2–mediated prostanoids may be essential for arteriolar vasodilation.
Administration of COX-2 selective inhibitors decreased proteinuria and inhibited development of glomerular sclerosis in rats with reduced functioning renal mass. In addition, COX-2 inhibition decreased mRNA expression of transforming growth factor-β 1 (TGF-β 1 ) and types III and IV collagen in the remnant kidney. Similar protection was observed with administration of nitroflurbiprofen (NOF), a nitric oxide (NO)–releasing NSAID without gastrointestinal toxicity. Prior studies also demonstrated that thromboxane synthase inhibitors retarded progression of glomerulosclerosis, with decreased proteinuria and glomerulosclerosis in rats with remnant kidneys, and in diabetic nephropathy in association with increased renal prostacyclin production and lower systolic blood pressure. Studies in models of types 1 and 2 diabetes have indicated that COX-2–selective inhibitors retard progression of diabetic nephropathy. Schmitz and associates have confirmed increases in TXB 2 excretion in the remnant kidney and correlated decreased arachidonic and linoleic acid levels with increased thromboxane production, because the thromboxane synthase inhibitor U63557A restored fatty acid levels and retarded progressive glomerular destruction.
Enhanced glomerular synthesis and/or urinary excretion of PGE 2 and TXA 2 have been demonstrated in passive Heymann nephritis (PHN) and Adriamycin-induced glomerulopathies in rats. Both COX-1 and COX-2 expression are increased in glomeruli with PHN. Thromboxane synthase inhibitors and selective COX-2 inhibitors also decreased proteinuria in PHN.
In contrast to the putative deleterious effects of thromboxane, the prostacyclin analog cicaprost retarded renal damage in uninephrectomized dogs fed a high-sodium and high-protein diet, an effect that was not mediated by amelioration of systemic hypertension. Similarly, EP2 and EP4 agonists decreased glomerular and tubulointerstitial fibrosis in a model of subtotal renal ablation. Other studies have also indicated that in models of polycystic kidney disease, there is increased COX-2 expression and increased PGE 2 and thromboxane in cyst fluid. Either COX-2 inhibition or EP2 receptor inhibition decreased cyst growth and interstitial fibrosis.
Prostanoids have also been shown to alter extracellular matrix production by mesangial cells in culture. TXA 2 stimulates matrix production by both TGF-β–dependent and TGF-β–independent pathways. PGE 2 has been reported to decrease steady-state mRNA levels of alpha 1(I) and alpha 1(III) procollagens, but not alpha 1(IV) procollagen and fibronectin mRNA, and to reduce secretion of all studied collagen types into the cell culture supernatants. Of interest, this effect did not appear to be mediated by cAMP. PGE 2 has also been reported to increase production of matrix metalloproteinase-2 (MMP-2) and to mediate Ang II–induced increases in MMP-2. Whether vasodilatory prostaglandins mediate decrease in fibrillar collagen production and increase in matrix-degrading activity in glomeruli in vivo has not yet been studied; however, there is compelling evidence in nonrenal cells that prostanoids may mediate or modulate matrix production. Cultured lung fibroblasts isolated from patients with idiopathic pulmonary fibrosis exhibit a decreased ability to express COX-2 and synthesize PGE 2 .
Acute Kidney Injury
When cardiac output is compromised, as in extracellular fluid volume depletion or congestive heart failure, systemic blood pressure is preserved by the action of high circulating levels of systemic vasoconstrictors (e.g., norepinephrine, Ang II, AVP). Amelioration of their effects in the renal vasculature serves to blunt the development of otherwise concomitant marked depression of renal blood flow. Intrarenal generation of vasodilator products of AA, including PGE 2 and PGI 2 , is a central part of this protective adaptation. Increased renal vascular resistance induced by exogenously administered Ang II or renal nerve stimulation (increased adrenergic tone) is exaggerated during concomitant inhibition of prostaglandin synthesis. Experiments in animals with volume depletion have demonstrated the existence of intrarenal AVP-prostaglandin interactions, similar to those described earlier for Ang II. Studies in patients with congestive heart failure have confirmed that enhanced prostaglandin synthesis is crucial in protecting the kidneys from various vasoconstrictor influences in this condition.
Renal dysfunction accompanying the acute administration of endotoxin in rats is characterized by progressive reductions in RBF and GFR in the absence of hypotension. Renal histology in such animals is normal, but cortical generation of COX metabolites is markedly elevated. A number of reports have provided evidence for a role for TXA 2 -induced renal vasoconstriction in this model of renal dysfunction. In addition, roles for PGs and TXA 2 in modulating or mediating renal injury have been suggested in ischemia and reperfusion and models of toxin-mediated acute tubular injury, including those induced by uranyl nitrate, amphotericin B, aminoglycosides, and glycerol. In experimental acute renal failure, administration of vasodilator PGs has been shown to ameliorate injury. Similarly, administration of nonselective or COX-2–selective NSAIDs exacerbates experimental ischemia-reperfusion injury.
COX-2 expression decreases in the kidney in response to acute ischemic injury. There is some controversy about the role of COX products in ischemia-reperfusion injury. Furthermore, fibrosis resulting from prolonged ischemic injury has been shown to be ameliorated by nonspecific COX inhibition. In contrast, renal injury in response to ischemia-reperfusion is worsened by COX-2–selective inhibitors or in COX-2 −/− mice, and administration of vasodilator PGs has been shown to ameliorate injury, possibly through a PPARδ-dependent mechanism.
Urinary Tract Obstruction
Following the induction of chronic (>24 hours) ureteral obstruction, renal PG and TXA 2 synthesis is markedly enhanced, particularly in response to stimuli such as endotoxins or bradykinins. Enhanced prostanoid synthesis, especially thromboxane, likely arises from infiltrating mononuclear cells, proliferating fibroblast-like cells, interstitial macrophages, and interstitial medullary cells. Selective COX-2 inhibitors may prevent renal damage in response to unilateral ureteral obstruction (UUO). However, PGE 2 acting through the EP4 receptor can limit tubulointerstitial fibrosis resulting from UUO. Prostaglandins derived from medullary COX-2 are mediators of the early phase of diuresis seen after relief of ureteral obstruction, because COX-2 inhibition prevents the acute (24-hour) phase of postobstructive diuresis. However, more persistent, chronic, postobstructive diuresis is not prostaglandin- dependent but results from downregulation of NKCC2 (Na + -K + -2Cl − cotransporter type 2) and decreases aquaporin-2 (AQP2) phosphorylation and translocation to the CCD membrane.
Allograft Rejection and Cyclosporine Nephrotoxicity
Allograft Rejection
Acute administration of a TXA 2 synthesis inhibitor is associated with significant improvement in rat renal allograft function. A number of other experimental and clinical studies have also demonstrated increased TXA 2 synthesis during allograft rejection, leading some to suggest that increased urinary TXA 2 excretion may be an early indicator of renal and cardiac allograft rejection.
Calcineurin Inhibitor Nephrotoxicity
Numerous investigators have demonstrated effects for cyclosporine A (CsA) on renal prostaglandin-TXA 2 synthesis and provided evidence for a major role for renal and leukocyte TXA 2 synthesis in mediating acute and chronic CsA nephrotoxicity in rats. Fish oil–rich diets, TXA 2 antagonists, or administration of CsA in fish oil as a vehicle have all been shown to reduce renal TXA 2 synthesis and may therefore afford protection against nephrotoxicity. Moreover, CsA has been reported to decrease renal COX-2 expression.
Hepatic Cirrhosis and Hepatorenal Syndrome
Patients with cirrhosis of the liver show an increased renal synthesis of vasodilating PGs, as indicated by the high urinary excretion of PGs and/or their metabolites. Urinary excretion of 2,3-dinor 6-keto-PGF 1α , an index of systemic PGI 2 synthesis, is increased in patients with cirrhosis and hyperdynamic circulation, thus raising the possibility that systemic synthesis of PGI 2 may contribute to the arterial vasodilatation of these patients. Inhibition of COX activity in these patients may cause a profound reduction in RBF and GFR, a reduction in sodium excretion, and an impairment of free water clearance. The sodium-retaining properties of NSAIDs are particularly exaggerated in patients with cirrhosis of the liver, attesting to the dependence of renal salt excretion on vasodilatory PGs. In the kidneys of rats with cirrhosis, COX-2 expression increases but COX-1 expression is unchanged; however, in these animals, selective inhibition of COX-1 leads to impaired renal hemodynamics and natriuresis, whereas COX-2 inhibition has no effect.
Diminished renal PG synthesis has been implicated in the pathogenesis of the severe sodium retention seen in hepatorenal syndrome, as well as in the resistance to diuretic therapy. There is reduced renal synthesis of vasodilating PGE 2 when there is activation of endogenous vasoconstrictors and a maintained or increased renal production of TXA 2 . Therefore, an imbalance between vasoconstricting systems and the renal vasodilator PGE 2 has been proposed as a contributing factor to the renal failure observed in this condition. However, administration of exogenous prostanoids to patients with cirrhosis is not effective for ameliorating renal function or preventing the deleterious effect of NSAIDs.
Diabetes Mellitus
In the streptozotocin-induced model of diabetes in rats, COX-2 expression is increased in the TAL–macula densa region, as well as in podocytes, possibly mediated by epigenetic processes. COX-2 immunoreactivity has also been detected in the macula densa region in human diabetic nephropathy. Studies have suggested that COX-2–dependent vasodilator prostanoids play an important role in the hyperfiltration seen early in diabetes mellitus, as well as in response to a high-protein diet. The increased COX-2 expression appears to be mediated, at least in part, by increased ROS production in diabetes, because the superoxide dismutase analog, tempol, blocks the increased expression.
Chronic administration of a selective COX-2 inhibitor significantly decreases proteinuria and reduces extracellular matrix deposition, as indicated by decreases in immunoreactive fibronectin expression and mesangial matrix expansion. In addition, COX-2 inhibition reduced expression of TGF-β, plasminogen activator inhibitor-1(PAI-1) and vascular endothelial growth factor (VEGF) in the kidneys of the diabetic hypertensive animals. Increasing intrarenal dopamine production also ameliorates diabetic nephropathy progression, at least in part by inhibiting renal cortical COX-2 expression. The vasoconstrictor TXA 2 may play a role in the development of albuminuria and basement membrane changes with diabetic nephropathy (DN). Also, administration of a selective PGE 2 EP1 receptor antagonist prevented development of experimental diabetic nephropathy, whereas EP4 receptor activation may exacerbate DN.
Pregnancy
Most, but not all, investigators do not report increases in vasodilator PG synthesis or suggest an essential role for prostanoids in the mediation of the increased GFR and RBF of normal pregnancy ; however, diminished synthesis of PGI 2 has been demonstrated in humans and in animal models of pregnancy-induced hypertension, which is associated with decreased expression of COX-2 and PGI 2 synthase in the placental villi. In animal models, inhibition of TXA 2 synthetase has been associated with resolution of the hypertension, suggesting a possible pathophysiologic role. A moderate beneficial effect of reducing TXA 2 generation, while preserving PGI 2 synthesis, by low-dose aspirin therapy (60 to 100 mg/day) has been demonstrated in patients at high risk for pregnancy-induced hypertension and preeclampsia.
Lithium Nephrotoxicity
Lithium chloride is a mainstay of treatment in the psychiatric treatment of bipolar illness. However, it is routinely complicated by polyuria and even frank nephrogenic diabetes insipidus. In vitro and in vivo studies have demonstrated lithium-induced renal medullary interstitial cell COX-2 protein expression via inhibition of glycogen synthase kinase-3β (GSK-3β). COX-2 inhibition prevented lithium-induced polyuria and also resulted in the upregulation of AQP2 and NKCC2.
Role of Reactive Oxygen Species as Mediators of COX-2 Actions
In addition to NADPH oxidase, nitric oxide synthase, and xanthine oxidase, COX-2 can also be a source of oxygen radicals. COX-2 enzymatic activity is commonly accompanied by associated oxidative mechanisms (co-oxidation) and free radical production. The catalytic activity of COX consists of a series of radical reactions that use molecular oxygen and generate intermediate ROS. Elevated levels of COX-2 protein are associated with increased ROS production and apoptosis in cultured renal cortical cells and human mesangial cells . It has been suggested that COX-2–mediated lipid peroxidation, rather than prostaglandins, can induce DNA damage via adduct formation. A COX-2 specific inhibitor, NS-398, was able to reduce the oxidative activity, with prevention of oxidant stress.
In addition to ROS generated by cyclo-oxygenase per se, prostanoids may also activate intracellular pathways that generate ROS. Locally generated ROS may damage cell membranes, leading to lipid peroxidation and release of arachidonic acid. Prostanoids released during inflammatory reactions cause rapid degenerative changes in some cultured cells, and their potential cytotoxic effect has been suggested to occur by accelerating intracellular oxidative stress. Thromboxane and PGE 2 acting through the EP1 receptor have been reported to induce NADPH oxidase and ROS production. Of interest, PGE 2 acting through the EP4 receptor inhibits macrophage oxidase activity. As noted, there is also evidence for cross-talk between COX-2 and ROS, such that ROS may induce COX-2 expression. Interestingly, during aging there is ROS-mediated NF-kB expression, which increases COX-2 expression in the kidney. Furthermore, this appears to induce a vicious cycle, since COX-2 then serves as a source of ROS. This interaction of COX-derived prostaglandin and ROS production has posited to play a role in development of hypertension. The amount of renal ROS resulting from COX activity increases with age, such that up to 25% of total kidney ROS production in aged rat kidneys is inhibited by NSAID administration.
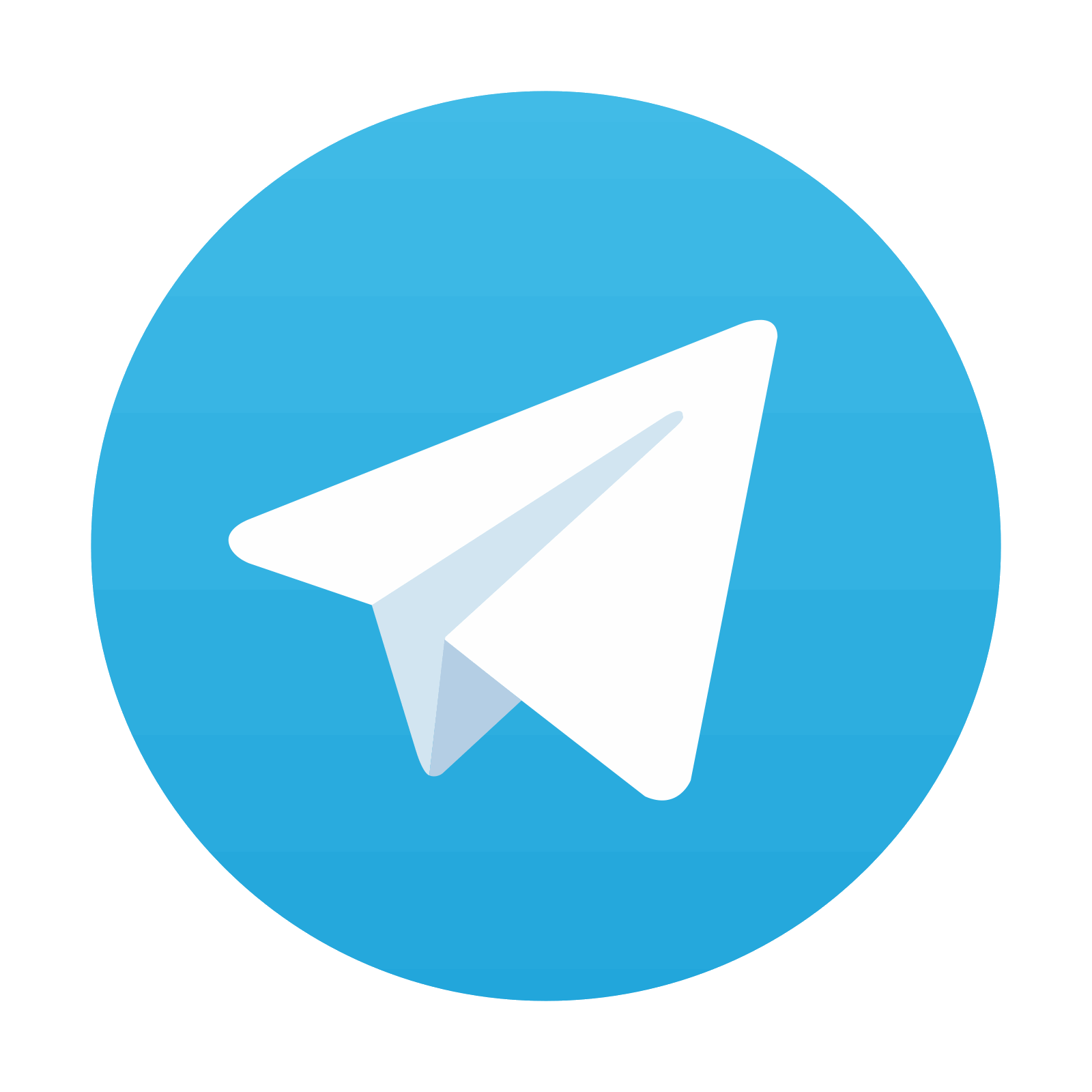
Stay updated, free articles. Join our Telegram channel
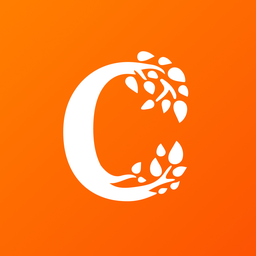
Full access? Get Clinical Tree
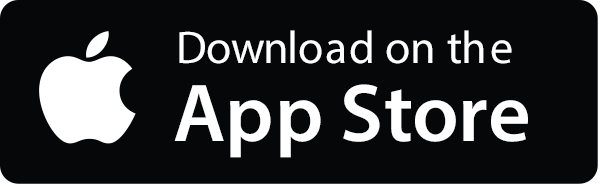
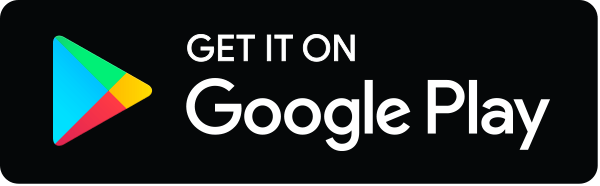