Chapter Outline
GENERAL INTRODUCTION TO ALDOSTERONE AND MINERALOCORTICOID RECEPTORS, 304
ALDOSTERONE SYNTHESIS, 305
MECHANISMS OF MINERALOCORTICOID RECEPTOR FUNCTION AND GENE REGULATION, 306
Mineralocorticoid Receptor Function as a Hormone-Regulated Transcription Factor: General Features and Subcellular Localization, 306
Domain Structure of Mineralocorticoid Receptors, 307
Mineralocorticoid Receptor Regulation of Transcription Initiation: Coactivators and Corepressors, 310
REGULATION OF SODIUM ABSORPTION AND POTASSIUM SECRETION, 310
General Model of Aldosterone Action, 310
Aldosterone and Epithelial Sodium Channel Trafficking, 311
Basolateral Membrane Effects of Aldosterone, 312
Activation of the Epithelial Sodium Channel by Proteolytic Cleavage, 312
Potassium Secretion and Aldosterone, 312
Separation of Sodium Absorption and Potassium Secretion by the Aldosterone-Sensitive Distal Nephron, 313
Aldosterone-Independent ENaC-Mediated Sodium Reabsorption in the Distal Nephron, 314
Sites of Mineralocorticoid Receptor Expression and Locus of Action Along the Nephron, 315
NONRENAL ALDOSTERONE-RESPONSIVE TIGHT EPITHELIA, 315
ROLE OF SERUM- AND GLUCOCORTICOID-REGULATED KINASE IN MEDIATING ALDOSTERONE EFFECTS, 317
Induction of SGK1 by Aldosterone, 317
Molecular Mechanisms of SGK1 Action in the Aldosterone-Sensitive Distal Nephron, 318
11β-HYDROXYSTEROID DEHYDROGENASE TYPE 2, 320
11β-HSD2: An Essential Determinant of Mineralocorticoid Specificity, 320
Sites of 11β-HSD2 Expression, 320
Impact of 11β-HSD2 on Mineralocorticoid Receptor Activity, 320
Apparent Mineralocorticoid Excess: A Disease of Defective 11β-HSD2, 320
Role of 11β-HSD2 in Blood Vessels, 320
Summary of 11β-HSD2 Roles, 321
NONGENOMIC EFFECTS OF ALDOSTERONE, 321
DISEASE STATES, 321
NONEPITHELIAL ACTIONS OF ALDOSTERONE, 322
In mammals, the control of extracellular fluid volume and blood pressure is intimately intertwined with the regulation of epithelial ion transport. Aldosterone, which is essential for survival, is the central hormone regulating the relevant epithelial transport processes, particularly of ions such as Na + , K + , and Cl − . All circulating aldosterone is generated in the adrenal glomerulosa, where its synthesis and secretion are under the control of angiotensin II and potassium, and its major epithelial actions occur in the distal nephron and colon. The former extends from the late distal convoluted tubule (DCT) through the connecting segment and the entire cortical and medullary collecting ducts. These segments, rich in mineralocorticoid receptor (MR), are often referred to as the aldosterone-sensitive distal nephron (ASDN). Most if not all effects of aldosterone are mediated by MR, a hormone-regulated transcription factor related closely to the glucocorticoid receptor and more distantly to other members of the nuclear receptor superfamily. The physiologic effects of aldosterone on epithelia entail direct gene regulatory actions of MR. Thus, a sound foundation for understanding aldosterone’s physiologic effects on the extracellular fluid, blood pressure, and electrolyte concentrations can be had through familiarity with MR-dependent effects on the transcription of various genes, which, in turn, alter epithelial ion transport. Aldosterone actions in certain disease states involve both genomic and nongenomic effects in both epithelial and nonepithelial tissues. This chapter addresses the cellular and molecular mechanisms underlying aldosterone action, focusing primarily on its effects on ion transport in epithelia but also highlighting key aspects of nonepithelial actions, which are of particular importance to its pathophysiologic effects.
General Introduction to Aldosterone and Mineralocorticoid Receptors
Steroid hormones are derived from cholesterol and produced in systemically relevant amounts in a relatively narrow range of tissues (adrenal glands, gonads, placenta, and skin). In mammalian physiology, six classes of steroid hormones are commonly recognized: mineralocorticoid, glucocorticoid, androgen, estrogen, progestin, and the secosteroid vitamin D 3 . This classification was based on observed effects of these hormones and has proven robust despite current appreciation of a much more diverse physiology of steroid hormones over and above their classical roles. In further support of this original classification is the characterization of six intracellular receptors (mineralocorticoid, MR; glucocorticoid, GR; androgen, AR; estrogen, ER; progestin, PR; vitamin D 3 , VDR). As further addressed later, it is now appreciated that a one-to-one relationship between receptor and hormone does not hold, and this is particularly the case for MR. Aldosterone was isolated and characterized in 1953. Crucial for its isolation was the application of radioisotopic techniques to measure [Na + ] and [K + ] flux across epithelia in the laboratory of Sylvia Simpson, a biologist, and Jim Tait, a physicist. Because of this, the active principle was initially called electrocortin; the name was soon changed to aldosterone when its unique aldehyde (rather than methyl) group at carbon 18 was discovered in collaborative studies between investigators in London and Basel. Aldosterone is commonly depicted so as to highlight this aldehyde group ( Figure 12.1 , right ). In vivo, the very reactive aldehyde group cyclizes with the β-hydroxyl group at carbon 11 to form the 11,18 hemiacetal and, in addition, may exist in an 11,18 hemiketal form. This cyclization of the 11β-hydroxyl group protects aldosterone from dehydrogenation by the enzyme 11β-hydroxysteroid dehydrogenase in epithelial tissue, which enables it to activate epithelial MR and thus regulate ion transport at very low (subnanomolar) circulating levels. There is broad evidence that aldosterone is not the only cognate ligand for MR, its essential effects via MR on epithelial ion transport notwithstanding. MR is found in high abundance in the hippocampus and cardiomyocytes, and in such nonepithelial tissues—which lack 11β-hydroxysteroid dehydrogenase type 2 (11β-HSD2; see “ 11β-Hydroxysteroid Dehydrogenase Type 2 ” section)—are essentially constitutively occupied by glucocorticoids (cortisol in humans, corticosterone in rodents). This is due to the comparable affinity and markedly higher plasma free levels (≥100-fold) of glucocorticoids compared with those of aldosterone. In terms of evolution, MR appeared well before aldosterone synthase (e.g., in fish). It was commonly assumed that MR and GR share a common immediate evolutionary precursor, although this has recently been challenged on sequence grounds, which implicate MR as the first of the MR/GR/AR/PR subfamily to branch off an ancestral receptor. A final reason not to equate MR and aldosterone action derives from a comparison of the MR knockout and aldosterone synthase knockout (AS −/− ) phenotypes. MR knockout mice (which lack all functional MR) cannot survive sodium restriction; AS −/− mice (which have no detectable aldosterone) survive even stringent sodium restriction but die when their fluid intake is restricted to that of wild-type animals. The survival of AS −/− mice on a low Na + intake may reflect, in part, Na + retention via renal tubular intercalated cells, in which MRs (not 11β-HSD2 protected) are activated by glucocorticoids in the context of high ambient angiotensin concentrations. Their inability to survive fluid restriction suggests an as yet poorly defined dependence on aldosterone for vasopressin action.

Aldosterone Synthesis
Aldosterone is synthesized in the adrenal cortex, which has three functional zones. The outermost layer of cells represents the zona glomerulosa, which is the unique site of aldosterone biosynthesis. Cortisol is synthesized in the middle zone, the zona fasciculata, and the innermost zona reticularis secretes adrenal androgens in many species, including humans, but not in rats or mice. Normally the glomerulosa secretes aldosterone at the rate of 50 to 200 µg/day, to give plasma levels of 4 to 21 µg/dL; in contrast, secretion of cortisol is at levels 200- to 500-fold higher. Underlying the separate synthesis of cortisol and aldosterone is expression of the enzyme 17α-hydroxylase uniquely in the zona fasciculata and that of aldosterone synthase uniquely in the glomerulosa.
In most species, aldosterone synthase, or cytochrome P450 (CYP) enzyme 11B2, is responsible for the conversion of deoxycorticosterone to aldosterone in a three-step process of sequential 11β-hydroxylation, 18-hydroxylation, and 18-methyl oxidation, to produce the characteristic C18-aldehyde from which aldosterone derives its name ( Figure 12.2 , left ). Although CYP11B2 is distinct from CYP11B1 (11β-hydroxylase) in most species, in some species (e.g., bovine), only a single CYP11B is expressed. How this enzyme is responsible for the three-step process of aldosterone synthesis in the glomerulosa but not the fasciculata is yet to be determined.

Figure 12.2 also shows key steps in biosynthesis of cortisol to illustrate the overlap and similarities with that of aldosterone. The genes encoding CYP11B1 and CYP11B2 lie close to one another on human chromosome band 8q24.3, so that an unequal crossing over at meiosis has been shown to be responsible for the syndrome of glucocorticoid-remediable aldosteronism (now known as familial hyperaldosteronism type I), in which the 3′ end of the CYP11B1 is fused to the 5′ end of CYP11B2. The chimeric gene product is expressed in the fasciculata and responds to adrenocorticotrophic hormone (ACTH) with aldosterone synthesis, producing a syndrome of juvenile-onset hyperaldosteronism and hypertension.
Normal glomerulosa secretion of aldosterone is primarily regulated by angiotensin II in response to posture and acute lowering of circulating volume, to plasma [K + ] in response to elevated potassium, particularly in settings of Na + deficiency, and to ACTH to the extent of entrainment of the circadian fluctuation in plasma aldosterone levels with those of cortisol. Aldosterone secretion is lowered by high levels of atrial natriuretic peptide and by the administration of heparin, somatostatin, and dopamine. As yet, incompletely characterized molecules of adipocyte origin have been shown to stimulate aldosterone secretion in vitro, and roles in the metabolic syndrome have been proposed on this basis.
Angiotensin and plasma [K + ] stimulate aldosterone secretion primarily by increasing the expression and activity of key steroidogenic enzymes as well as the steroidogenic acute regulatory protein (StAR). StAR is required for cholesterol transport into mitochondria and hence is available for steroid synthesis. Regulated steroidogenic enzymes include side-chain cleavage enzyme, 3β-hydroxysteroid dehydrogenase, and, most notably, aldosterone synthase, which mediates the final step in aldosterone synthesis. Common to the mechanism of stimulation by angiotensin II and [K + ] is elevation of intracellular [Ca 2+ ]. Angiotensin activates angiotensin type I receptors in the glomerulosa cell membrane, which in turn activate phospholipase C. Elevated [K + ] increases intracellular [Ca 2+ ] by depolarizing the cell membrane and activating voltage-sensitive Ca 2+ channels. Subsequently, the pathways diverge, with the [K + ] effect but not the angiotensin effect dependent on Ca 2+ /calmodulin kinase. Patients taking angiotensin-converting enzyme inhibitors or angiotensin receptor blockers usually show a degree of suppression of aldosterone secretion, reflected in a modest (0.2 to 0.3 mEq/L) elevation in plasma [K + ]. This is often sufficient to establish a new steady state, with plasma aldosterone levels rising into the normal range, a process best termed breakthrough rather than escape, given the time-honored usage of the latter for escape from progression of the salt and water effect of mineralocorticoid excess in the medium and long term.
Recent data have shed new light on the regulation of aldosterone production by the adrenal glomerulosa in health and disease. Choi and associates found recurrent somatic mutations in the K + channel Kir3.4 (encoded by the gene KCNJ5 ), which were present in more than a third of human aldosterone-producing adenomas studied. These mutations increased Na + conductance through Kir3.4 and resulted in increased Ca 2+ entry and enhanced aldosterone production and glomerulosa cell proliferation. Interestingly, an inherited mutation in KCNJ5 is associated with hypertension associated with marked bilateral adrenal hyperplasia (now known as familial hyperaldosteronism type III [FH-III]). These findings suggest that KCNJ5 may provide tonic inhibition of aldosterone production and glomerulosa cell proliferation. In glomerulosa cells harboring the mutant channel, both proliferation rate and aldosterone synthesis are increased. These initial studies have been continued and extended by a much wider survey by Boulkroun and colleagues ; more recently, less common but similarly somatic mutations in the adrenal cortex (ATP1A1, ATP2A3, CACNA1D) have been associated with hyperaldosteronism caused by adrenal adenomas.
Mechanisms of Mineralocorticoid Receptor Function and Gene Regulation
Mammals cannot survive without MR, except with substantial NaCl supplementation. This member of the nuclear receptor superfamily appears to have both genomic and nongenomic actions; however, the latter do not appear to play a significant role in the control of epithelial ion transport. This section thus focuses exclusively on the function of MR as a hormone-regulated transcription factor.
Mineralocorticoid Receptor Function as a Hormone-Regulated Transcription Factor: General Features and Subcellular Localization
In the presence of agonists, MR binds to specific genomic sites and alters the transcription rate of a subset of genes. Figure 12.3 shows the fundamental paradigm of MR function. All nuclear receptors shuttle in and out of the nucleus; however, in the absence of hormone, some, such as the estrogen and the vitamin D receptors, are predominantly nuclear, whereas others, like GR, are almost exclusively cytoplasmic. In the absence of hormone, MR is distributed relatively evenly between nuclear and cytoplasmic compartments, but in the presence of hormone, it is highly concentrated in the nucleus ( Figure 12.4 ). It is also notable that in addition to this marked change in MR cellular distribution, its subnuclear organization and protein-protein interactions are also changed. Like its close cousin the GR, the unliganded MR (in the absence of hormone) is complexed with a set of chaperone proteins, which include the heat shock proteins hsp90, hsp70, and hsp56, and immunophilins. This chaperone complex is essential for several aspects of MR function, notably high-affinity hormone binding and trafficking to the nucleus. It was thought for many years that after binding hormone, the hsp90-containing chaperone complex is jettisoned. However, it has become clear that this complex remains associated with the receptor and plays an important role in nuclear trafficking. Several members of the immunophilin family, including FKBP52, FKBP51, and CyP40, are present in the chaperone complex and provide a bridge between hsp90 and the cytoplasmic motor protein dynein, which moves the receptor-hsp90 complex retrogradely along microtubules to the nuclear envelope. Here, the receptor is handed off to the nuclear pore protein, importin-α, and translocated into the nucleus, where it functions as a transcription factor, stimulating transcription of certain genes and repressing the transcriptional activity of others. In the regulation of ion transport, stimulation of key target genes is paramount. Transcriptional repression may be essential for effects in nonepithelial cells, including neurons, cardiomyocytes, smooth muscle cells, and macrophages.


Domain Structure of Mineralocorticoid Receptors
The MRs of all vertebrates are highly conserved. There are only minor differences between MRs in rodents and MRs in humans. In general, the steroid and nuclear receptors have been divided into three major domains ( Figure 12.5 ): (1) an N-terminal transcriptional regulatory domain, (2) a central DNA-binding domain (DBD), and (3) a C-terminal ligand/hormone-binding domain (LBD). Each of these broadly defined domains has more than one function, and not all of the functions can be neatly assigned to separate distinct domains; however, much of what MRs do can be understood from this point of view. In the following sections, the domains are described roughly in the historical order in which they were characterized, which also parallels the clarity of functional and structural knowledge about them.

DNA-Binding Domain
The sine qua non of MR function as a transcription factor is its ability to bind specifically to DNA. This protein-DNA interaction is mediated by the receptor’s compact modular DBD (amino acids 603 to 688 of human MR; Figure 12.5 shows a strip diagram and two-dimensional structure), which forms a variety of contacts with a specific 15-nucleotide DNA sequence termed a hormone response element (HRE). Receptor binding to the HRE in the vicinity of regulated genes promotes the recruitment of coactivators and components of the general transcription machinery, such as the TATA-binding protein, which binds to the thymidine- and adenosine-rich DNA sequence found upstream of many genes and is required for correct transcription initiation. These types of HREs have been identified near or in many of the key MR-regulated genes, such as serum- and glucocorticoid-regulated kinase 1 (SGK1), glucocorticoid-induced leucine zipper (GILZ), and amiloride-sensitive sodium channel subunit α (α ENaC). Although in many cases, differential binding to HREs is a key determinant of the specificity of many transcription factors, it should be noted that some steroid receptors (notably MR and GR) have only minor (<10%) differences within this domain and have identical DNA-binding properties. Specificity in these cases is determined through other mechanisms.
The canonical MR HRE is a 15-nucleotide sequence that forms a partial palindrome (inverted repeat), which binds a receptor homodimer. A dimer interface embedded within the DBD is essential for MR to form these requisite homodimers, as well as to form heterodimers with GR. Mutations that disrupt this interface have complex effects on receptor activity in animals and cultured cells, and similar mutations in other receptors (AR in particular) result in disease states. Also, in at least one kindred of the autosomal dominant form of pseudohypoaldosteronism type I, an MR DBD mutation appears to be causative, although the mechanistic basis has not been elucidated.
In addition to supporting DNA binding and dimerization, the DBD also harbors a nuclear localization signal, as well as surfaces that contact distant parts of the receptor and that mediate interactions with other proteins, as has been shown for GR and, in some cases, MR.
Ligand/Hormone-Binding Domain
The MR LBD comprises amino acids 689 to 981 (see Figure 12.5 A ). Like the DBD, the LBD has multiple functions: in addition to binding with high affinity to various MR agonists and antagonists, it also harbors interaction surfaces for coactivators, dimerization, and N-C interactions. MR is distinct from GR in that it binds with equal, high affinity to cortisol, corticosterone (the physiologic glucocorticoid in rats and mice), and aldosterone. Indeed, as discussed later, MR appears to function as a high-affinity glucocorticoid receptor in some tissues, including the brain and the heart.
High-resolution representations of the crystal structures of wild-type and mutant MR have identified the structural features of the LBD and specific amino acid contacts involved in binding to the mineralocorticoid desoxycorticosterone ( Figure 12.6 ). Key features include the following: (1) The LBD of MR, like that of other nuclear receptors, is arranged into 11 α-helices and four small β-strands. (2) The C-terminal α-helix, H12, contains the activation function AF2. (3) Ligand is deeply embedded into a pocket comprising α-helices H3, H4, H5, H7, and H10, and two β-strands; numerous contacts are made between amino acids of the pocket and the hormone. This accounts for the slow off rate and high affinity of aldosterone, corticosterone, and cortisol for MR. The crystal structure of the mutant (S810L) MR, in which progesterone acts as an agonist rather than an antagonist, reveals that H12 is stabilized with AF2 in the active conformation. The crystal structure of wild-type MR LBD also provides insight into the mechanisms underlying some forms of pseudohypoaldosteronism type I. Notably, MR/S810L has an LBD mutation in helix 5, which is predicted to disrupt interaction with the steroid ring structure, whereas Q776R and L979P have been demonstrated to have markedly reduced aldosterone binding. Structural analysis reveals that Q776 is located in helix H3 at the extremity of the hydrophobic ligand-binding pocket and anchors the steroid C3-ketone group.

MR binds cortisol and corticosterone with an affinity similar to that of aldosterone. 11β-HSD2 is an essential determinant of aldosterone specificity, through its effect in metabolizing glucocorticoids to their receptor-inactive keto-congeners in collecting duct principal cells, as discussed later. In tissues that do not coexpress 11β-HSD2, the physiologic ligand for MR is cortisol (corticosterone in rats and mice). The extent to which such “unprotected” MR can be pathophysiologically activated in aldosterone excess states is discussed in the “Primary Aldosteronism” section under “Disease States” and in the “Nonepithelial Actions of Aldosterone” section.
N-Terminal Domain
As its noncommittal name implies, the N-terminal region of MR has diverse functions, which appear to revolve primarily around protein-protein interactions and recruitment of coactivators and corepressors. It has two potent transcriptional regulatory motifs, usually termed AF1a and AF1b. This domain bears some functional and sequence similarity to the homologous region of GR and is capable of stimulating gene transcription when fused to an unrelated DBD. Overall, however, MR and GR differ markedly in the N-terminal domain, and this region of the receptor is a central determinant of specificity. Recent evidence supports the idea that this domain has functional sequences that limit receptor activity through recruitment of corepressors, in addition to transcriptional activation functions. Its role in coactivator and corepressor recruitment is addressed further in the following section.
Mineralocorticoid Receptor Regulation of Transcription Initiation: Coactivators and Corepressors
The major mechanism of MR action is its effects on transcription initiation; however, there may also be effects on transcript elongation. Much has been learned about the generation of an initiation complex and the particular roles that steroid receptors play in this process. Several review articles and book chapters provide in-depth examinations of the biochemistry of the general transcription machinery, transcription initiation, promoter escape, and processive elongation. Most of the coactivators identified so far interact with the C-terminal AF2 domain and include the prototypical GRIP1/TIF2 and SRC, which sequentially recruit a series of different components of the transcriptional machinery and result in the formation of a preinitiation complex (PIC). This PIC includes all of the key components of the transcription machinery, including RNA polymerase II. A detailed picture of MR-dependent PIC formation has not been obtained. However, the general features are likely similar to those for ER and involve the sequential recruitment by the receptor of (1) chromatin-remodeling SWI/SNF and CARM1/PRTM1 proteins, which promote chromatin remodeling and initiation of complex formation; (2) histone acetylase CBP/P300 (cyclic adenosine monophosphate [cAMP]–responsive element–binding protein), which promotes an active chromatin conformation ; and (3) direct or indirect recruitment of the TATA-binding protein and other components of the general transcription machinery.
The aforementioned mechanisms are generic and are used by many transcription factors, including all steroid receptors, through interactions with the C-terminal AF2 domain. The N-terminal region of MR, which harbors the AF1 domain, diverges from the other steroid receptors, and recent evidence has identified coregulators that interact selectively with this receptor domain. ELL (eleven-nineteen lysine-rich leukemia factor) is a coactivator for MR that specifically interacts with AF1b and assists in PIC formation. It was originally identified as an elongation factor, and it may also affect transcript elongation. Other specific coregulators include the synergy inhibitory protein PIAS1, Ubc9, and p68 RNA helicase.
Regulation of Sodium Absorption and Potassium Secretion
General Model of Aldosterone Action
Aldosterone enters the cell passively, binds to MR, triggers changes in gene transcription (as addressed in the “Mechanisms of MR Function and Gene Regulation” section), and potentially has nongenomic effects. Aldosterone effects in the ASDN have been divided into three major phases: latent, early, and late. This designation goes back to the early observations by Ganong and Mulrow that after aldosterone infusion into experimental animals, no effect was observed for at least 15 to 20 minutes. A similar delay was observed in isolated epithelia. The early phase, which is now known to involve primarily MR-dependent regulation of signaling mediators such as SGK1, culminates in increased apical localization—and possibly increased probability of the open state—of ENaC. In the late phase, aldosterone stimulates transcription of a variety of effector genes, including those that encode components of the ion transport machinery, notably the ENaC and Na + -K + –adenosine triphosphatase (Na + -K + -ATPase) subunits. The major direct effect is to increase Na + reabsorption, which is accompanied variably by Cl − reabsorption and/or K + secretion, and ultimately water reabsorption. Aldosterone’s actions in the principal cells of the connecting segment and collecting duct ( Figure 12.7 ) are of primary significance; however, it also has been shown to influence fluid and electrolyte transport in other tubule segments, as well as in other organs. These actions of aldosterone can be surmised from the clinical features of individuals with aldosterone-secreting tumors; they have volume expansion with high blood pressure and are commonly (≈50%) hypokalemic. In general, the effects of aldosterone on Na + absorption and K + secretion work together. However, there are ways that these actions can be separated, as discussed later.

The two basic cell processes that aldosterone regulates—Na + absorption and K + secretion—are depicted in Figure 12.7 . Most aspects of this mechanism are relevant to the various aldosterone target tissues.
Na + -K + -ATPase, located on the basolateral membrane (blood side), establishes the essential electrochemical gradients that drive ion transport (see Chapters 5 and 6 ). The most important, rate-limiting step is that of Na + entry into the cell via the epithelial Na + channel, ENaC. The discovery of the molecular composition of ENaC in 1993 opened the door to understanding how aldosterone functions to regulate this critically important ion channel. Most Na + transporters are encoded by a single gene product. In contrast, ENaC is composed of three similar but distinct subunits, each encoded by a unique gene. All three subunits come together (the stoichiometry is controversial) to form an ion channel with unique biophysical characteristics, the most striking of which is the relatively long time it stays open or closed. The complete loss of any one of these subunits in mice is incompatible with life.
The entry of Na + into the cell via ENaC in the apical membrane is the rate-limiting step in both Na + absorption and K + secretion. Na + enters the cell down a steep electrochemical gradient; intracellular [Na + ] is approximately 10 mmol/L, and the membrane voltage is strongly negative inside the cell. The Na + inside the cell is pumped out across the basolateral membrane by Na + -K + -ATPase, as addressed in detail in Chapter 6 . Most epithelial cells have a greater density of K + channels on the basolateral membrane and thus recycle K + back into the blood. The distal nephron is unique in that it has an unusually high density of K channels on the apical membrane (primarily Kir 1.1 [renal outer medullary potassium (ROMK)] and BK channels). This distribution of K + channels permits a large amount of K + that enters the cell via Na + -K + -ATPase to exit the cell into the lumen and be excreted into the urine. The vast majority of K + that appears in the urine is secreted by the distal nephron.
Much attention has been focused on the early phase of aldosterone action because it appears to be more tractable to dissection and the majority of change in Na + current occurs during this phase. This separation is probably somewhat artificial, however, and there is considerable overlap in events that define the early and late phases. Moreover, many efforts to manipulate mediators of the early phase (through overexpression and knockdown experiments) have been evaluated after prolonged alteration. Nevertheless, there is some heuristic value in considering early and late phases of aldosterone action separately.
In cultured collecting duct cells deprived of corticosteroids and then exposed to high concentrations of aldosterone, an increase in ENaC-mediated Na + transport can be observed in well under an hour, which is consistent with animal studies. Na + transport continues to increase for 2 to 3 hours, then plateaus for a few hours, and then gradually increases over the next several hours. After 12 hours of exposure to saturating aldosterone concentrations, the increase in ENaC activity is near maximal. The molecular basis for this increase in ENaC activity has been intensively investigated, and several key events are now apparent.
For aldosterone to increase ENaC activity, a change in gene transcription must occur. One of the earliest response genes is SGK1 . This discovery has had a major impact on the direction of research on aldosterone action, and this gene is addressed separately—together with its major target, Nedd4-2—later in this chapter. SGK1 is particularly important for the early actions of aldosterone. An important consideration in evaluating our understanding of this molecular pathway is that the genetic disease Liddle’s syndrome provided the first clues that the C-terminal portions of the ENaC subunits were essential in regulating ENaC surface expression (see Chapter 45 ).
Aldosterone and Epithelial Sodium Channel Trafficking
The major action of aldosterone is to increase the number of functional ENaC units on the apical membrane. This process can involve an increase in the number of channel complexes on the surface, or activation of existing complexes, or both. There is evidence to support both, although the bulk of evidence favors the idea that change in the number of ENaC complexes predominates. The redistribution of ENaC to the apical membrane can be detected in less than 2 hours after aldosterone exposure.
It is less well established whether the number of ENaC complexes is increased through increased insertion, decreased removal, or both. Aldosterone probably contributes to both processes. Rapid insertion of ENaC complexes is best understood with regard to the actions of cAMP. The extent to which the molecules involved in cAMP-mediated insertion are also involved in aldosterone action is uncertain, but some common mechanisms probably are used. Trafficking to the apical membrane appears to involve hsp70, SNARE (soluble NEM-sensitive factor attachment protein receptor) proteins, and the aldosterone-induced protein melanophilin. The mitogen-activated protein kinase pathway may also be involved, because interruption of ERK phosphorylation by GILZ increases ENaC surface expression.
Considerably more is known about how ENaC complexes are retrieved from the apical membrane. This understanding is the direct result of dissecting the molecular consequences of Liddle’s syndrome, in which mutations in the C terminus of ENaC lead to increased residence time in the apical membrane. The missing or mutated domains in the β- or γ-subunit of ENaC in this syndrome normally bind to Nedd4-2, a ubiquitin ligase, which ultimately is responsible for initiating endocytosis and degradation. The interaction of Sgk1 and Nedd4-2 in the actions of aldosterone is discussed later. ENaC is internalized via clathrin-coated vesicles and processed into early endosomes, then further processed into recycling endosomes and late endosomes. Degradation is via lysosomes or proteasomes. The processing of ENaC by vesicular trafficking and its regulation by aldosterone has been reviewed.
Phosphatidylinositol-3-kinase (PI3K)–dependent signaling is essential for epithelial Na + transport. It controls SGK1 activity (addressed later) and also appears to have independent effects on ENaC open probability through direct actions of 3-phosphorylated phosphoinositides, particularly phosphatidylinositol (3,4,5) trisphosphate. Ras-dependent signaling may also regulate ENaC and the pump in complex ways that depend on downstream signaling through Raf, MEK and ERK, as well as through PI3K.
The late phase of ENaC activation by aldosterone is less well understood than the early phase. A simple evaluation of the late phase is that aldosterone increases the transcription and protein abundance of the ENaC subunits. This idea comes from the fact that aldosterone increases the mRNA and protein abundance of α-ENaC in the kidney after a lag of several hours. However, whereas aldosterone produces an increase in α-subunit expression in the kidney, it produces an increase of β- and γ-subunits in the colon. Dietary Na + restriction, a physiologically relevant maneuver that increases aldosterone secretion, clearly increases ENaC surface expression in the renal distal nephron. However, there appear to be some important differences between chronic aldosterone administration to an Na + -replete animal and chronic dietary Na + restriction. The role of increased expression of α-ENaC in the long-term actions of aldosterone has been questioned, because overexpression of this subunit does not increase ENaC activity in models of collecting duct and lung epithelia. It appears that increased expression of α-ENaC may be important for the consolidation of the increase, but it is not sufficient to reproduce the steroid-mediated increase in ENaC activity.
Basolateral Membrane Effects of Aldosterone
Over the years, research on aldosterone action has focused with varying degrees of intensity on apical effects, basolateral effects, and effects on metabolism. There is general agreement now that the early effects of aldosterone are on apical events, primarily on ENaC, and that basolateral and metabolic effects come later. In addition, although it is somewhat less settled whether the basolateral effects are direct or result indirectly from the enhanced entry of Na + into cells, the bulk of evidence favors the latter view. Notably, increased Na + entry has been found to control more than 80% of increased Na + -K + -ATPase activity and basolateral membrane density in the rat and rabbit cortical collecting tubule. Furthermore, striking increases in basolateral membrane folding and surface area occur in aldosterone-treated animals, an effect that is markedly attenuated in animals fed a low-Na + diet. This result strongly suggests that apical Na + entry is required for basolateral changes to occur. However, good evidence exists for direct transcriptional stimulation of Na + -K + -ATPase subunit expression, as well as reports supporting some direct effects of aldosterone in increasing basolateral pump activity or at least in constituting the pool of latent pumps, which are then recruited to the basolateral membrane in response to a rise in intracellular [Na + ].
Activation of the Epithelial Sodium Channel by Proteolytic Cleavage
There is now clear evidence that when ENaC is delivered to the apical membrane, it can be activated by proteolytic cleavage. The first hint of this process was the demonstration that rats fed a low-Na diet or given aldosterone over the long term showed the appearance of a proteolytic fragment of the γ-ENaC subunit. Subsequently, investigators have shown that both the α- and the γ- but not the β-ENaC subunits can be cleaved. Furthermore, cleavage at each site apparently initiates a degree of activation of the channel complex. ENaC complexes are activated by cleavage because specific regions of the large extracellular domain (26 residues in the α-ENaC and 46 residues in the γ-ENaC) are excised. These regions contain inhibitory sequences that, when introduced exogenously, can inhibit ENaC function. Removing these regions by proteolytic cleavage releases this inhibition.
Several proteases can cleave either the α- or γ-ENaC subunits. Among them are furin, prostasin, CAP2, kallikrein, elastase, matriptase, plasmin, and trypsin. It is not clear whether activation of ENaC by proteolytic cleavage can be regulated by aldosterone, but the idea certainly has attractive features. Were aldosterone able to regulate expression of one or more rate-limiting proteases, it would be able to regulate both the number of complexes in the apical membrane and the ability of the channel complex to be active. It appears that aldosterone may regulate the expression of prostasin. Aldosterone may also regulate the expression of the protease, nexin-1 (an inhibitor of prostasin), and other proteases.
The discovery of ENaC activation by cleavage helps to explain how aldosterone might increase ENaC activity by increasing both surface expression and the activity of a single ENaC complex. By phosphorylating Nedd4-2 via SGK1 and reducing its ability to bind to the PY domains of the ENaC subunits, aldosterone increases ENaC residence time on the apical membrane. This additional time permits proteolytic activation by one or more endogenous proteases.
Potassium Secretion and Aldosterone
One of the major effects of aldosterone is to increase K + secretion (and thus excretion). This phenomenon has been demonstrated in countless patients with aldosterone-secreting tumors and in hundreds of studies in animals given excess amounts of aldosterone. The general mechanism whereby aldosterone increases K + secretion is depicted in Figure 12.7 . The key feature of this process involves the increased absorption of Na + via ENaC. The dependence of K + secretion on Na + absorption is the basis of the action of the “K-sparing” diuretics, amiloride and triamterene, both of which inhibit ENaC. These drugs have no direct effect on the apical K + channels.
Increasing ENaC activity produces two major secondary effects that, in turn, enhance K + secretion. First, the enhanced Na + conductance of the apical membrane produces depolarization of that membrane and hence a more favorable electrical driving force for K + efflux into the lumen. The second effect relates to the activity of the Na + -K + pump on the basolateral membrane. The more Na + that enters across the apical membrane, the more that must be extruded by the pump. Since the stoichiometry of the pump is constant (3Na + and 2K + ), more K + enters the cell. In isolated, perfused cortical collecting ducts, the amount of secreted K + is highly related to the amount of absorbed Na + when the stimulus for Na + absorption is mineralocorticoid hormone.
Two kinds of K + channels are found in the apical membrane of the ASDN: small conductance (SK, 30 to 40 picosiemens) channels encoded by the ROMK gene, and large conductance (BK, 100 to 200 picosiemens) channels found in many kinds of cells, including the apical membrane of the colon. The majority of the K + channels on the apical membrane of the principal cells appear to be SK, at least as far as can be assessed by patch clamp analysis. The activity of either channel is not increased by aldosterone. .
A feature of K + secretion is that although apical K + channels are abundant in the proximal portion of the ASDN (connecting tubule and cortical collecting duct), they are strikingly less abundant in the medullary collecting duct. Because apical K channels are not regulated by aldosterone, their absence in the medullary collecting duct might uncouple aldosterone-regulated Na + reabsorption from K + secretion in this segment.
Recent advances in understanding genetic forms of hypertension have uncovered an important role for a family of kinases that lack an otherwise conserved lysine residue in the catalytic domain. These kinases, called WNK ( w ith n o lysine; K is the one-letter code for lysine), have potent effects on pathways regulating Na + and K + transport in the distal nephron. An important feature of one member of this family, WNK4, is its ability to modulate the activity of ROMK. The activity of ROMK can be regulated via WNK4 by signaling events activated by aldosterone but modulated by serum [K + ], Na balance via angiotensin II, and SGK1 activity. The importance of this synthesis is that it provides a molecular mechanism for the collecting duct separately to regulate Na + absorption via ENaC and K + secretion via ROMK.
Separation of Sodium Absorption and Potassium Secretion by the Aldosterone-Sensitive Distal Nephron
The preceding sections establish a picture that parsimoniously accounts for the effect of aldosterone to stimulate, pari passu, Na + reabsorption and K + secretion. The simple stimulation of electrogenic Na + reabsorption (via ENaC) is sufficient to stimulate K + secretion, which fits well for organisms faced with a combined low-Na + , high-K + diet, which was maintained most of the time through millions of years of vertebrate evolution. However, organisms do not ingest a fixed amount of Na + and K + , so inexorable linkage between Na + absorption and K + secretion by the ASDN cannot possibly occur all the time. Investigators have proposed several possibilities to explain how these processes can be separated.
Role of Distal Tubule Fluid Delivery
A traditional view for differential Na + and K + handling stems from the differing role that aldosterone plays in potassium secretion depending on the tubular flow (and thus “sodium flow”) rate. Studies from adrenalectomized dogs have demonstrated that the primary regulators of potassium excretion are serum potassium concentration and tubular flow rate. A higher serum potassium concentration yields a higher filtered load of potassium. Hyperkalemia also stimulates natriuresis from upstream segments of the nephron. This latter effect can increase tubular flow rate, which, in turn, diminishes potassium concentration in the lumen and activates flow-stimulated BK channel–mediated potassium secretion in the collecting duct. In the setting of sufficient distal delivery of sodium, potassium loading will not yield a higher steady-state concentration of aldosterone, as the two mechanisms previously mentioned are sufficient to normalize serum potassium. However, under conditions of sodium depletion, proximal Na + reabsorption is increased, which further diminishes distal delivery of sodium and hence tubular flow rate. This diminishes flow-mediated potassium secretion, so that aldosterone secretion is necessary to normalize potassium balance.
Independent Regulation of Sodium and Potassium Transporters
Other possible mechanisms have been suggested, which involve separate regulation of sodium and potassium transport (e.g., ENaC and ROMK) by specific stimuli depending on the state of Na + and K + intake. With a constant Na + intake, one could envision that a high-potassium diet could enhance the activity of ROMK, while a low-potassium diet would reduce its activity. Such an effect would cause more or less of the K + entering the cell via the Na + -K + pump to be recycled across the basolateral membrane. This mechanism, although probably very complex in its execution, is appealing in its simplicity. The actions of WNK4 may be central to the regulation of ROMK.
Electroneutral Versus Electrogenic Sodium Reabsorption
Other appealing possibilities invoke the differing nature of Na + and K + transport along the distal nephron. For example, DCT absorbs Na + and Cl − via the electroneutral Na-Cl cotransporter (NCC), and enhanced activity in this segment would not stimulate K + secretion. Such a situation occurs in pseudohypoaldosteronism type II or familial hyperkalemic hypertension (also referred to as Gordon’s syndrome). Indeed, potassium, independent of aldosterone, is known to regulate the NCC. A high-potassium diet inhibits this transporter and would thus promote aldosterone-mediated sodium reabsorption only through ENaC (rather than both NCC and ENaC), while favoring potassium secretion via ROMK and also potassium and flow-induced K + secretion via BK channels.
Shift to Medullary Collecting Duct
Another possibility involves modulation of Na + absorption by the medullary collecting duct, a segment that has little capacity to secrete K + . The environment of the renal medulla is very different from that of the cortex, and endogenous paracrine factors such as prostaglandins E 2 and transforming growth factor–β, both of which have potent inhibitory effects on Na + transport, are increased in response to a high-NaCl diet.
Regulation of Chloride Transport
A fifth possibility involves the independent regulation of Cl − transport by the collecting duct. Cl − can be absorbed by the paracellular pathway (i.e., between cells) driven by the lumen-negative voltage across the epithelium. This pathway can be influenced by aldosterone. Cl − can also be absorbed through the cells by specific transporters. One example of a Cl − transporter in the collecting duct is pendrin, an anion exchanger present on the apical membrane of intercalated cells. Mice that lack this transporter do not tolerate NaCl restriction as well as normal mice. This transporter seems not to be upregulated by aldosterone, but is dependent on Cl − delivery to the distal nephron and is upregulated by angiotensin II.
Differential Regulation of Intercalated Cell Mineralocorticoid Receptor
A recent study has highlighted a sixth possible mechanism allowing distinct responses to volume depletion versus hyperkalemia. The central feature of this proposed regulation is differential phosphorylation of the MR LBD in intercalated cells, as shown schematically in Figure 12.8 . When phosphorylated at S843 in the LBD, MRs cannot bind aldosterone or cortisol and thus cannot be activated. This phosphorylation, which is stimulated by hyperkalemia, occurs selectively in intercalated cells but not principal cells. Angiotensin II, on the other hand, induces S843 dephosphorylation in intercalated cells, markedly increasing ligand binding and, therefore, activation. Intercalated cells are known predominantly to mediate H + transport; however, recent studies have implicated them in electroneutral NaCl transport via the combined actions of Na + -dependent Cl − -HCO 3 − exchanger (NDCBE) and the apical Cl − -HCO 3 − exchanger, pendrin. Thus, when MR is active in these cells (S843 dephosphorylated), electroneutral NaCl transport occurs, without enhancing the driving force for K + secretion. Since intercalated cells lack 11β-HSD2, under these conditions, it is cortisol that binds to and activates MR. When intercalated cell MR is inactive (S843 phosphorylated), aldosterone acts in principal cells to stimulate ENaC-dependent electrogenic Na + transport, which enhances K + secretion.

Aldosterone-Independent ENAC-Mediated Sodium Reabsorption in the Distal Nephron
The term aldosterone-sensitive distal nephron emphasizes the primacy of this key steroid in the control of ion transport in this region of the nephron. However, ENaC activity and aldosterone sensitivity exhibit axial heterogeneity from the late distal convoluted tubule (DCT2) through the connecting tubule to the cortical collecting duct and, finally, to the medullary collecting duct. In mice on a standard salt diet, total ENaC expression increases with progression from the DCT2 to the connecting tubule, although ENaC apical localization and activity are higher in the DCT2. Only under conditions of low-sodium diet or aldosterone administration does the primacy of the connecting tubule in particular, and to a lesser extent the cortical collecting duct, emerge. The total luminal surface area in the DCT2 and connecting tubule is several-fold higher than in the cortical collecting duct, and together these two segments appear to be sufficient to maintain sodium balance, even in the absence of detectable ENaC along the collecting duct. Mice lacking ENaC selectively in the collecting duct come into balance, even on a low-sodium diet. Congruent with these findings, deletion of α-ENaC from the DCT2, connecting tubule and collecting duct results in severe sodium wasting. Notably, it is the connecting tubule that appears to be most important in the response to aldosterone, while DCT2 has the highest baseline transport in the absence of MR activation. The cortical collecting duct is not as critical as was originally thought for either baseline or aldosterone-stimulated sodium reabsorption, probably due to its smaller surface area compared with the DCT2 and connecting tubule.
As we continue to traverse the nephron, further sodium reabsorption is minimal in the medullary collecting duct, under standard sodium diet conditions, and not significantly stimulated by aldosterone.
Sites of Mineralocorticoid Receptor Expression and Locus of Action Along the Nephron
Aldosterone-Sensitive Distal Nephron
In the kidney, MR is expressed at the highest levels in distal nephron cells extending from the last third of the DCT through the medullary collecting duct, which is frequently referred to as the aldosterone-sensitive distal nephron (ASDN) ( Figure 12.9 ). This pattern of expression was first demonstrated using labeled hormone–binding studies performed before the cloning of MR and has been confirmed since by several methods, including polymerase chain reaction, in situ hybridization, and immunohistochemical analysis. Effects of aldosterone on electrogenic Na + and K + transport in principal cells have been found consistently in these nephron segments, which also express ENaC, and 11β-HSD2, as addressed in detail earlier. Collecting duct intercalated cells also express MR and respond specifically to aldosterone and alter proton secretion. Aldosterone directly increases the activity of the H + -ATPase in the collecting duct, and its absence results in decreased proton secretion. Interestingly, nongenomic stimulation of H + -ATPase activity in type A intercalated cells has been demonstrated in isolated murine collecting ducts. Consistent with these effects, aldosterone deficiency results in distal renal tubular acidosis type 4, and excess aldosterone results in metabolic alkalosis. It should be noted that aldosterone also stimulates H + secretion due to effects on principal cell Na + transport, which alter the electrical gradient. These older studies must also now be interpreted in the context of more recent data, which, as noted earlier, demonstrate that the effect of aldosterone on intercalated cells depends on the genesis of the signal.

Other Sites of Expression
MR has been identified at some level in all parts of the nephron examined, including the glomerulus. Its effects, at least at some of these sites, are likely to be physiologically relevant in states of volume depletion and acid-base disturbances; however, the data are not as robust and consistent as those for the ASDN.
Glomerulus
MR (but not 11β-HSD2) is expressed in glomerular mesangial cells, where it is thought to affect proliferation and production of reactive oxygen species and to have profibrotic effects through SGK1. These effects have been suggested to be important in the progression of renal damage, particularly in diabetic nephropathy, where glucocorticoids mimic the activity of aldosteronism in the context of tissue damage. However, the physiologic role of mesangial cell MR is uncertain.
Proximal Convoluted Tubule
Hierholzer and Stolte showed, through elegant microperfusion studies, that the sodium reabsorptive capacity of the proximal convolution was decreased in adrenalectomized animals and restored by administration of aldosterone. Chronic volume depletion increases sodium reabsorption in the proximal convoluted tubule, which is in part mediated by MR. The mechanisms of action in this nephron segment are controversial. Recent studies indicate an MR-dependent increase in the activity of Na + -H + -exchanger isoform 3, possibly through an increase in trafficking of the transporter to the membrane. This transporter contributes to sodium and bicarbonate reabsorption. MR activation, in turn, may activate the Na + -K + -ATPase in the basolateral membrane of the proximal convoluted tubule to maintain a gradient for sodium reabsorption.
Medullary Thick Ascending Limb
In the medullary thick ascending limb, mineralocorticoids but not glucocorticoids increase sodium and chloride reabsorption. In rodents, adrenalectomy impairs reabsorption of NaCl in the medullary thick ascending limb, and aldosterone restores this process. This reabsorptive defect may contribute to the urinary concentrating and diluting abnormality measured in patients with Addison’s disease and in mice lacking aldosterone synthase. The medullary thick ascending limb also participates in the regulation of acid-base balance by reabsorbing most of the filtered HCO 3 that is not reabsorbed by the proximal tubule. In this context, aldosterone has been shown to stimulate the Na + -H + exchanger in amphibian thick ascending limb, possibly through a rapid nongenomic effect. Recent evidence has also implicated regulation of the Na-K-2Cl cotransporter type 2 in the thick ascending limb—as well as the NCC in the DCT (see later)—by oxidative stress response kinase 1 (OSR1) and STE20/SPS1-related proline/alanine–rich kinase (SPAK) (OSR1/SPAK).
Distal Convoluted Tubule
The DCT is also capable of mineralocorticoid specificity. Hormone studies in rodents demonstrate that aldosterone increases expression of the NCC and its apical membrane abundance without changes in mRNA expression. The recently described family of WNK kinases may play a pivotal role in mediating this effect. Aldosterone acts through MR to increase NCC phosphorylation, which appears to be important for the changes in its expression and apical targeting. Recent evidence supports the idea that aldosterone-induced NCC phosphorylation occurs through a signaling cascade in which SGK1 phosphorylates WNK4, which, in turn, phosphorylates OSR1 and SPAK. OSR1/SPAK then directly phosphorylates NCC at three serine/threonine sites, which results in increased activity. It is interesting to note that SGK1 may also be a target of WNK1.
Nonrenal Aldosterone-Responsive Tight Epithelia
The mineralocorticoid effects of aldosterone have predominantly been studied in the distal nephron, but do influence other—mostly ENaC-expressing—tight epithelia. ENaC is present in visceral epithelial cells of the distal colon, distal lung, salivary glands, sweat glands, and taste buds.
Colon
Under physiologic conditions, approximately 1.3 to 1.8 L of electrolyte-rich fluid is reabsorbed per day from the colonic epithelium, which accounts for about 90% of the salt and water that enter the proximal colon from the terminal ileum. In nonmammalian vertebrates, sodium conservation by the colon plays an even more significant role. This transport is regulated by several transporters and channels, including ENaC. Like the nephron, the proximal colon reabsorbs sodium via an electroneutral, ENaC-independent process. In the distal colon, electrogenic Na + absorption via ENaC channels is the predominant mode of sodium transport. In disease states such as inflammatory bowel disease, ENaC-mediated sodium reabsorption can be reduced, although in diarrheal states, elevated aldosterone levels may attenuate sodium and water loss from the colon. It should be noted that in the colon, as in the distal nephron, MR signaling is aldosterone selective, reflecting the activity of 11β-HSD2. Aldosterone increases electrogenic sodium absorption and potassium secretion and inhibits electroneutral absorption. This is in contrast to glucocorticoids, which, at higher concentrations, activate GR to stimulate electroneutral absorption in the proximal and distal colon. As in the distal nephron, the aldosterone response can be characterized by an early and late response. The early response gene, SGK1, is upregulated by aldosterone via MR. However, in contrast with the kidney, aldosterone and a low-salt diet have been shown to stimulate transcription of β- but not α-ENaC in rat models.
Aldosterone stimulates electrogenic potassium secretion from colonic epithelia. The significance of this secretion is evident in anuric patients. Potassium secretion from the colon is much higher in patients undergoing long-term hemodialysis than in patients not undergoing dialysis. Indeed, administration of fludrocortisone, a mineralocorticoid agonist, to dialysis patients has been shown to reduce hyperkalemia in small clinical trials. Low doses of the common MR antagonist spironolactone do not result in significant hyperkalemia.
Lung
Vectorial transport of salt and water across the distal airway epithelium (ciliated Clara cells, nonciliated cuboidal cells) and alveoli (type I and type II alveoli) primarily determines fluid clearance from the lung. ENaC is the rate-limiting step in sodium transport in the lung and plays a primary role in several physiologic and pathophysiologic conditions determined by fluid clearance. At birth, the lung assumes a resorptive phenotype, and lack of functional ENaC channels leads to neonatal respiratory distress syndrome in mouse knockout models. In children, lack of functional ENaC (e.g., autosomal recessive pseudohypoaldosteronism type I) results in increased rates of recurrent infection due to increased airway liquid. In the mature lung, defective ENaC channels can lead to pulmonary edema and pathologic conditions (e.g., acute respiratory distress syndrome and high-altitude pulmonary edema ). Conversely, hyperabsorption through ENaC is emerging as an important mechanism of decreased mucus clearance in cystic fibrosis.
The molecular apparatus for mineralocorticoid-stimulated liquid reabsorption via ENaC (concomitant MR and 11β-HSD2) is present in late gestational and mature adult lung in humans and rats, and there is some evidence for a significant physiologic role of aldosterone in ENaC-mediated sodium transport, although glucocorticoids acting via GR are likely to play the predominant role in lung. Importantly, glucocorticoids, but not mineralocorticoids, play a critical role in lung maturation in humans, and GR knockout mice, like α-ENaC knockout mice, die of respiratory insufficiency within hours of birth. In contrast, MR knockout mice demonstrate a severe salt-wasting phenotype but no significant lung phenotype.
Exocrine Glands and Sensors
ENaC-mediated sodium reabsorption is also measurable in salivary and sweat glands. The importance of these tissues for sodium and water homeostasis is underscored by rare genetic mutations that confer elevated plasma aldosterone levels and pseudohypoaldosteronism with normal renal tubular function but significant sodium loss from salivary or sweat glands. ENaC channels also play an important role in transduction of sodium salt taste in the anterior papillae of the tongue. The appropriate molecular machinery for mineralocorticoid-responsive sodium reabsorption is expressed in these organs, and these epithelia are model systems for the study of ENaC regulation. As in colonic epithelia, aldosterone stimulates expression of β- and γ-ENaC and sodium transport in glands and taste buds in animal models. Moreover, in humans, changes in dietary sodium are inversely proportional to sodium transport across salivary epithelia. As in the aldosterone-responsive distal nephron and distal colon, sodium uptake is coupled with potassium secretion in salivary epithelia. This effect is evident in humans with hyperaldosteronism. Such patients have a salivary [Na + ]/[K + ] ratio significantly lower than that of subjects without the disorder, although this has not been accepted as a valid means to screen for hyperaldosteronism.
Role of Serum- and Glucocorticoid-Regulated Kinase in Mediating Aldosterone Effects
Induction of SGK1 by Aldosterone
In the early to mid-1960s, primarily from the work of Isidore Edelman and colleagues, it became clear that aldosterone, like cortisol, exerted most, if not all, of its key physiologic effects by altering transcription rates of a specific subset of genes. In particular, hormone-induced changes in gene transcription were shown to be essential for its effects on epithelial Na + transport. Attention focused first on enzymes of intermediary metabolism, particularly citrate synthase, and after the cloning of the transporters involved in Na + translocation (Na + -K + -ATPase and ENaC), these were also found to be regulated by aldosterone at the transcriptional level. However, these effects are manifest several hours after most of the change in Na + transport has already occurred and hence could not explain the early and greatest proportion of effects of aldosterone. Considerable effort by many groups went into unbiased screening for aldosterone-regulated proteins and later aldosterone-regulated mRNAs (reviewed in Verrey ). In 1999, SGK1 was identified as the first early-onset aldosterone-induced gene product, which clearly stimulates ENaC-mediated sodium reabsorption in the distal nephron without pleiotropic effects on other cellular processes. The physiologic relevance of SGK1 is now firmly established, and investigations by numerous laboratories into its mechanism of action have revealed critical general features of the mechanism underlying hormone-regulated ion transport: it is therefore addressed in some detail here. SGK1 mRNA levels are increased within 15 minutes, and protein levels within 30 minutes, in cultured cells upon stimulation by aldosterone and in the collecting duct by aldosterone or a low-salt diet (a physiologic stimulus for aldosterone secretion) in vivo. Notably, SGK1 is increased more abundantly in kidney cortex (the connecting tubule and cortical collecting duct) than in medulla, which corresponds to the potency of ENaC activation in these nephron segments. SGK1 is expressed in other nephron segments, including glomeruli, proximal tubule, and papillae ; however, its rapid induction specifically in the ASDN appears to provide most of the basis for its role in aldosterone-regulated sodium and potassium transport. It is of interest that SGK1 is highly expressed in glomeruli and inner medulla and papillae in the absence of aldosterone; recent data are consistent with the idea that its inner medullary induction is related to its role in osmotic responses.
Molecular Mechanisms of SGK1 Action in the Aldosterone-Sensitive Distal Nephron
SGK1 is a serine/threonine kinase of the AGC protein kinase superfamily, and its kinase activity appears to be essential for Na + transport regulation. Although it has effects on proliferation and apoptosis in kidney cells, these effects appear to be minor, and the control of ENaC and other transporters predominates. SGK1 transcription is induced by a variety of stimuli in addition to aldosterone. As its name implies, these include serum and glucocorticoids, but also follicle-stimulating hormone, transforming growth factor–β, and hypotonic and hypertonic stress. Of these, osmotic regulation is of the clearest physiologic relevance.
SGK1 is interesting as a signaling kinase in that both its expression level and its activity are highly regulated. Regulation of the former occurs primarily through effects on gene transcription, although protein stability is also regulated, whereas regulation of the latter occurs through phosphorylation. Like that of its close relative Akt, SGK1 phosphorylation is stimulated by a variety of growth factors including insulin and insulin-like growth factor-1, which act through PI3K to trigger phosphorylation at two key residues, an activation loop (residue T256) and a hydrophobic motif (S422). Both of these phosphorylation events appear to be PI3K dependent. Specifically, the α-isoform of the p110 subunit of PI3K stimulates PI3K-dependent kinase 1 and mTORC2 (PDK2), respectively. Recent evidence has established that PDK2 is in fact the mammalian target of rapamycin [mTOR] in its complex 2 variant. mTORC2 also uses a co-factor, SIN1, to specify activation of SGK1 rather than related family members such as Akt. In turn, the upstream kinases, PI3K-dependent kinase 1 and mTORC2, phosphorylate and thereby activate SGK1 kinase, which is required for its stimulation of ENaC. Thus, SGK1 serves as a convergence point for different classes of stimuli, which act on the one hand to control its expression (aldosterone) and on the other to control its activity (insulin), which results in the coordinate regulation of ENaC.
In the study of the physiologic and pathophysiologic role of SGK1 in the ASDN, mice lacking SGK1 have provided considerable insight. Unlike MR knockout mice, mice lacking SGK1 survive the neonatal period and appear normal when consuming a diet with normal salt levels, although their aldosterone levels are markedly elevated. When subjected to a low-salt diet, these mice have a profound sodium-wasting phenotype (pseudohypoaldosteronism type I). Notably, this is a significantly milder phenotype than in the MR knockout. This comparison suggests that disruption of SGK1 signaling may be insufficient to eliminate aldosterone-mediated sodium transport due to additional aldosterone-induced and aldosterone-repressed proteins that could compensate for the lack of SGK1. Other early aldosterone-induced genes, including K-ras, GILZ, kidney-specific WNK1, Usp45, melanophilin, and promyelocytic leukemia zinc finger, have also been implicated in the stimulation of ENaC. Their distinct mechanisms of action have not been studied in vivo and are beyond the scope of this chapter.
Alternatively, SGK1 may play a more significant role in states of aldosterone excess or upregulation of hormonal activators of SGK1 (e.g., insulin). Indeed, SGK1 knockout mice are protected from the development of salt-sensitive hypertension, which accompanies the hyperinsulinemia of the metabolic syndrome. Despite its accepted role as a mediator of aldosterone-stimulated sodium reabsorption, the mechanisms by which SGK1 stimulates ENaC are not fully characterized. Several mechanistic studies have demonstrated that SGK1 is rapidly induced but also rapidly degraded. The N terminus of the kinase, which distinguishes SGK1 from other kinase family members (e.g., Akt), is important for stimulation of sodium transport but is also the target for rapid degradation of the kinase via the ubiquitin-proteasome system. In addition, several naturally occurring variants of SGK1 possess distinct N termini that modify the ability to stimulate ENaC and to be degraded. The pathophysiologic implications of the N terminus for sodium transport are unclear, but they may involve a negative feedback loop to limit sodium reabsorption in states of hypertension.
The molecular mechanisms of ENaC stimulation by SGK1 can be divided into three known categories ( Figure 12.10 ): (1) posttranslational effects on the E3 ubiquitin ligase Nedd4-2, (2) posttranslational Nedd4-2–independent effects, and (3) transcription of gene products such as α-ENaC.
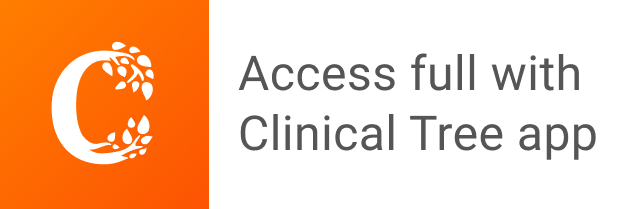