1.2
Wound Healing in the Urinary Tract
Motaz ElMahdy Hassan1 and Mohamed Ismail2
1 NHS Grampian, Aberdeen Royal Infirmary, Aberdeen, UK
2 Portsmouth Hospitals, NHS Trust, Portsmouth, UK
Abstract
Wound healing is a dynamic process that demonstrates the body’s ability to respond to change in its protective integrity and maintain homeostasis by swiftly responding to this change. Wounds, either surgically or trauma induced, are a form of cellular injury that leads to a tissue response. This response is a complex process which involves the removal of necrotic tissue and induction of repair. When tissue injury occurs, the damaged blood vessels haemorrhage into the defect, platelets aggregate, and a thrombus forms. This process allows the interaction with the complement system, and inflammatory cells are attracted to the site of injury by chemotactic factors. Platelets play an essential role in this response as they release two important factors. These factors are platelet‐derived growth factor (PDGF) and transforming growth factor beta (TGF‐β); they are powerful chemotactic factors for inflammatory cells such as macrophages, which then migrate into the wound to phagocytose necrotic tissue and fibrin. PDGF induces the cells to change from the resting phase in G0–G1. Epidermal growth factor (EGF) and insulin‐like growth factor (IGF) act to induce cell progression from G1 phase to DNA synthesis. Capillary proliferation is stimulated with angiogenic growth factors such as vascular endothelial growth factor (VEGF). The defect is repaired by capillary hyperplasia, myofibroblasts, and epithelial cells.
Nutrients and hormones play a vital role in the wound‐healing process, as insulin, thyroid hormones, glucose, amino acids, and vitamin C. The deficiency in nutrients or vitamins or the presence of infection or poor local circulation may lead to delay in wound healing.
Keywords: wound healing; surgical incisions; inflammatory response; effect of urine; suture materials
1.2.1 Introduction
Throughout history, wound healing has been a subject of interest in all the civilizations. Since the time of ancient Egyptians, 1600 BCE, the Edwin Smith surgical papyrus described the different types of wounds. The Ebers Papyrus, 1550 BCE, described the use of different materials that can be applied to the wounds (e.g. honey, grease, and lint to absorb wound discharges). Warriors and gladiators of the Roman Empire suffered a large number of injuries, and Galen, who was appointed as a physician to the gladiators, described that maintenance of moisture in the wound promoted healing.
For many centuries, wound healing and pathology has evolved, but the risk of infection was always a major concern. At the beginning of the nineteenth century, Semmelweis, the Hungarian obstetrician (1818–1865) found that sepsis after childbirth was much lower if the medical students attending the birth washed their hands with soap and hypochlorite. Louis Pasteur (1822–1895) proved that germs are introduced to the wounds from the environment. In 1865, Joseph Lister, who was a professor of surgery in Glasgow, read Pasteur’s work and started using carbolic acid (phenol) on wounds. This has vastly reduced the rate of infection, and he then used phenol for hand washing and sterilising instruments and sprayed carbolic acid in the theatre to limit infection. This reduced the rate of infection from 50% to 15.
Gradually, the type of dressings being used evolved to encompass a wide array of customised dressings that allow variable degrees of permeability, absorbency, and antiseptic properties. Currently, wound management involves the application and manipulation of growth factors, cytokines, and bioengineered tissue [1].
1.2.2 Wound‐Healing Process
The cell is a dynamic entity that maintains homeostasis despite continuous changes in the environment. When the changes are severe, cellular injury will occur. There are various mechanism of cellular injury: physical, chemical, and biological. In surgery, these three mechanisms may occur simultaneously or sequentially. A surgical incision is a form of physical injury or trauma to the tissue that may result in another form of tissue insult, such as hypoxia and predisposition to infection as a result of the breach of protective barriers. The response to injury also depends on various factors, such as, the nutritional status of the patient, blood supply to the injured area, and immunity. Previous radiation or chemotherapy may preclude an adequate response of the tissue to heal.
The response to cellular injury whether pathological as in trauma or physiological as in surgery is the same, and that is the process of inflammation. The inflammatory response is a sequential reaction to cellular injury. The mechanism of inflammation is basically the same regardless of the insulting agent. The response depends on the extent and the severity of injury and on the patient’s individual response. The inflammatory response can be divided into a vascular response, cellular response, formation of exudate, and healing [2].
1.2.3 Vascular Response
After cellular injury, arterioles in the area briefly undergo transient vasoconstriction. Histamine and other chemicals are released by the injured cells leading to vasodilation. This vasodilation results in hyperaemia, which raises filtration pressure. Vasodilation and chemical mediators cause endothelial cell retraction, which increases capillary permeability that facilitates the movement of fluid from capillaries into tissue spaces, inflammatory exudate.
Exudate is composed of serous fluid that contains plasma proteins, primarily albumin, exerting an oncotic pressure that draws more fluid leading to tissue oedema. The products of the injured cells activates the plasma protein, fibrinogen, to form fibrin. Fibrin strengthens the blood clot formed by platelets. The function of the clot is to trap bacteria and prevent their spread and to serve as a framework for the healing process.
1.2.4 Cellular Response
As more fluid is lost, the blood viscosity increases, and the blood flowing through the capillaries of the injured tissue slow down. Neutrophils and monocytes move to the inner surface of the capillaries by the process of margination and then by diapedesis, which is movement by an amoeboid fashion through the capillary wall to the site of injury. Chemotaxis is the directional migration of white blood cells (WBCs) along a concentration gradient of chemotactic factors, which are substances that attract leukocytes to the site of inflammation. Chemotaxis is the mechanism for ensuring accumulation of neutrophils and monocytes at the focus of injury.
Neutrophils arrive first at the site of injury, usually within 6–12 hours; they phagocytise damaged cells, foreign material, and bacteria. To maintain the supply for the inflammatory process, the bone marrow releases more neutrophils into the circulation, and these result in an elevated WBC count. Occasionally, the demand for neutrophils increases to the extent that the bone marrow releases immature forms of neutrophils, called ‘bands’ or ‘segmented neutrophils’, into circulation. Increased numbers of band neutrophils in the circulation is called a ‘shift to the left’, which is commonly found in patients with acute bacterial infections. Neutrophils have a short life span of 24–48 hours. Dead neutrophils and cellular and bacterial debris accumulate and form pus.
Monocytes are also phagocytic cells that migrate from circulating blood. They are attached to the site by chemotactic factors such as mononuclear attractant protein‐1 (MCP‐1), fibrinopeptides, and macrophage inflammatory proteins and usually arrive at the site within three to seven days after the onset of inflammation. Monocytes transform into macrophages after arrival at the tissue spaces and phagocytose the inflammatory debris. The role of the macrophages is to clear the debris in the injured tissue before healing can occur. Macrophages have a longer life span, and as they multiply, they remain in the injured tissue for weeks; they are instrumental in the healing process. Macrophages accumulate and fuse to form multinucleated giant cells that can engulf large particles that are too large for single macrophages. Giant cells are encapsulated by collagen and form a granuloma. This process has been classically described when tuberculosis bacillus infects the lung. As the bacillus is encapsulated, a chronic state of inflammation ensues. As the granuloma forms, the process will continue, and this cavity will contain necrotic tissue.
Each cellular component has a different role in the inflammatory process. Basophils and eosinophils have a more selective role. During an allergic reaction, eosinophils, which constitute up to 5% of the WBCs, are released in large quantities and they target organisms that are too large to be engulfed. Their mode of killing involves secreting toxic substances (e.g. reactive O2 compounds), major basic protein, which is toxic to parasites, and several enzymes. Eosinophils also release inflammatory mediators (e.g. prostaglandins, leukotrienes, platelet‐activating factor, and many cytokines).
Basophils share several characteristics with mast cells. When these cells encounter specific antigens, they undergo cellular degranulation and release preformed inflammatory mediators, such as platelet‐activating factor and histamine. They also synthesise new mediators such as leukotrienes, prostaglandins, and thromboxanes. Connective tissue mast cells contain chymase, tryptase, and heparin. Through the release of these mediators, mast cells play an important role in generating a protective acute inflammatory response. The main role of lymphocytes that arrive later at the injury site is humeral and cell‐mediated immunity.
The complement system plays a major role in the mediation of the inflammatory response. It contributes to increasing the vascular permeability, and enhancing phagocytosis, chemotaxis, and cellular lysis. When the complement system is activated, it works in a sequential order, which is C I, C4, C2, C3, C5, C6, C7, C8, and C9. These numbers reflect the order of their discovery. The activation of the complement system is through the fixation of the component CI to the antigen–antibody complex, which is the primary pathway. The complement is fixed by immunoglobulins IgG and IgM. When each complex is activated, it acts on the next component, hence, creating a cascade effect. In the alternative pathway; C3 is activated without prior antigen–antibody fixation [3].
1.2.5 Urinary Tract Healing
The healing process in the urinary tract after surgical intervention is slightly unique from other tissues because of the presence of urine. Due to the various surgical approaches in urological surgery, the technique involved, the location of the procedure, and the organ operated on, all contribute to the outcome of this process.
The common notion of dividing the urinary tract during surgery, which is applied in open surgery, involves division and suture of tissue. The tissue edges are bonded with fibrin, which will stimulate the growth of capillaries to form granulation tissue which will be gradually replaced by fibrous tissue, which matures to form a scar in the course of few weeks to months as a result of the remodelling process. The peculiarities of each procedure, technique, and organ involved affect the healing process. These unusual features will be discussed in the next section.
1.2.6 Different Methods of Making Surgical Incisions
In urology, there are a myriad procedures that are performed through endoscopic and minimally invasive approaches. Endoscopic surgery has replaced many conventional procedures that required open surgery and the use of the scalpel. The use of endoscopes that gradually became smaller, more robust, and provide multichannel apparatus that allows the surgeon to perform many procedures like biopsy, resection, removal of foreign body, and stone fragmentation. To provide a safe surgical environment, good visibility is essential, and hence, haemostasis and techniques for haemostasis have also evolved. Such devices include diathermy, laser technology [1], and the ‘ultrasonic scalpel’ [2]. To achieve ideal haemostasis, the application of thermal devices to the resected tissue will lead to a degree of tissue damage. This creates nonviable tissue that leads to more wound induration, and as the tissue has lost part of its blood supply, the sloughed tissue left behind acts as a foreign body and increases the risk of infection [3, 4].
A skin incision heals through a conventional process of granulation tissue formation which is gradually covered with an advancing film of epithelial cells that conglomerate from the wound edges by mitotic activity to close the defect [5]. In the urothelium, the process is different because this type of tissue has abundant mitoses in every layer.
The notion that all tissue defects healed the same way was defied as an unexpected finding that was reported by Brauer [6] but was largely ignored until confirmed nearly 30 years later by Johnson and McMinn [7], who found that healing in the biliary tree, the salivary ducts, and the urinary tract followed a different pattern to that of skin or intestinal mucosa. After endoscopic resection of bladder pathology, the defect is filled with the usual granulation tissue and gradually over this layer, a film of urothelium rapidly spreads because of the abundant mitotic activity in the cells that grow from the edge of the wound. This is in contrast to the skin and bowel pattern of healing because the mitotic activity in these tissues are found some distance away from the wound edge (Figure 1.2.1).

Figure 1.2.1 (a–d) A nest of cells may become buried under the healing urothelium.
This inwardly growing thin film of urothelium becomes thicker and eventually takes on the features of normal urothelial tissue. The agility and rapidity of urothelial growth has two important implications: (i) any cavity (such as a urinoma) which is in communication with the urinary tract may be relined with urothelium, and (ii) the regeneration of the urothelium is so rapid and effective that it can cover over and bury little islands of the original damaged urothelium.
1.2.7 Von Brunn’s Nests and Metaplasia
More than 100 years ago, Von Brunn [8] described how these buried islands of urothelium would form cysts or ‘nests’. More than a decade later, Giani [9], while studying the healing of transitional epithelium in dogs, observed every stage between burying of fragments of epithelium, the development of von Brunn’s nests, and the formation of full‐blown cystitis cystica and was able to create similar nests by implanting the vesical epithelium.
Similar epithelial growths caused by healing may occur in the skin where they give rise to innocent implantation dermoids [10]. In the urinary tract, they are peculiarly dangerous. The little nests become progressively thicker until they actually form columnar epithelium (Figure 1.2.1). In other mammals, patches of columnar mucosa are considered normal, but in humans, they are potentially malignant and are followed by the development of adenocarcinoma [11–14].
1.2.8 Squamous Metaplasia
Conditions that lead to recurrent inflammation and healing as a result of chronic irritation or infection can lead to the formation of squamous metaplasia (e.g. because of a calculus, bilharziasis, or a long‐term indwelling catheter). The transitional urothelium changes into stratified squamous epithelium, which forms keratin and comes to resemble the skin, and in the presence of urine, changes into a characteristic white plaque – leucoplakia – which is a precursor for neoplasia. It must be distinguished from the innocent ‘vaginal metaplasia’, which is a normal feature of the trigone. The risk of malignant transformation probably varies from one site to another.
1.2.9 Heterotopic Ossification
The urothelium presents an interesting entity because a graft of urothelium placed in contact with almost any type of connective tissue may induce the formation of heterotopic bone, which is most likely to develop in the vicinity of a foreign body such as a nonabsorbable suture but may be found whenever urothelium grows in an unusual site or is used as a graft (e.g. around the track of a chronic urinary fistula or around a urinoma or pseudocyst).
1.2.10 Regeneration of Smooth Muscle in the Urinary Tract
For quite some time, it has been considered that smooth muscle could regenerate across a gap in the bladder or the ureter. This was the basis for the intubated ureterotomy of Davis [15], in which a stricture in the ureter was incised and a splint was left in for six weeks and which then became surrounded by fibrous tissue, lined with a film of urothelium, and finally enclosed in smooth muscle. The same principle was considered and lay behind the idea that the bladder could regenerate after cystectomy, especially if a mould of plastic was left for it to grow over [16]. Today, it is believed that muscle does not truly regenerate but spreads in from the cut margin of the urethra [17].
1.2.11 Particular Effects of Urine
1.2.11.1 The Presence of Urine Modifies the Normal Process of Healing in the Urinary Tract
- Prolongation of the healing process. The remodelling phase continued to the tenth day for the dermis and until the twelfth day for the urethra. It is suggested that the possible reason for the prolonged urethral wound healing is because of urine extravasation through the urethra into the surrounding tissues.
- Necrosis: Urine may interfere with the healing process and may lead to tissue necrosis. An example of this manifestation is the loss of skin and subcutaneous tissue that can follow extravasation of urine after injury to the perineal urethra when the skin of the penis, scrotum, and lower abdomen may be affected and tissue damage may take place and lead to necrosis and slough formation. Also this is evident in cases of chronic urinary incontinence, when skin excoriation is noticed. The process by which urine affects the skin or tissue is not entirely clear, but it could be multifactorial as the duration of exposure, the pre‐existing skin condition, the nutritional status of the individual, and factors in the composition of urine itself (e.g. hyperosmolarity, alkaline, or acidic pH) and secondary infection. The subcutaneous injection of almost any fluid that is markedly hypertonic, acid, or alkaline is well known to give rise to pain and inflammation, and in practice even if infection is not present at first, because the tissue is damaged by inflammation, infection may inevitably follow.
The extravasation of urine, for example, after cystectomy may lead to necrosis of the momentum and delay the healing process at the anastomotic sites. It may also lead to tissue breakdown and formation of fistulas (e.g. enterocutaneous fistulas).
- Contracture: The effect of urine by causing contracture formation on scar tissue is an important issue. All scars contract to some extent, and contracture in scars which cross joints is a common problem that may affect joint mobility. In the urinary tract, as the healing process continues through tissue remodelling, the sites of anastomosis could be affected by stricture formation. Urine exacerbates the tendency for scars to contract. When urine is diverted from the site of an injury to the urethra, the formation of a stricture may be prevented. Taking this notion into consideration, surgical technique can have an impact on this process so as to avoid the effect of scar tissue and stricture formation; however, considerable ingenuity in planning the anastomotic technique in the urinary tract is essential. The anastomosis can be designed in the form of a long elliptical shape so that even after healing and scar‐tissue formation, the lumen would still be adequate for maintaining drainage (Figure 1.2.2).

Figure 1.2.2 To avoid stenosis, all anastomoses in the urinary tract are designed as an ellipse so that there will still be an adequate lumen even after contracture has taken place.
1.2.12 Suture Materials, Splints, Meshes, and Films
1.2.12.1 Suture Materials
There are different types of suture material and are classified into absorbable or non‐absorbable and monofilament or braided (multifilament) (Table 1.2.1). Commonly used absorbable sutures are synthetic, whereas the non‐absorbable can be natural or synthetic.
Table 1.2.1 Suture types, material, and absorption time.
Material | Absorption time (days, strength retention) | |
Absorbable multifilament | ||
Vicryl | Polyglycolic acid polymer | 56–70 (75% at 2 weeks, 50% at 3 weeks, and 25% at 4 weeks) |
Vicryl rapide | Polyglycolic acid polymer | 30–40 (75% at 2 weeks, 50% at 3 weeks, and 25% at 4 weeks) |
Absorbable monofilament | ||
Monocryl | Poliglecaprone | 90–120 (60% to 70% at 1 week 30% to –40% at 2 weeks) |
PDS | Polydioxanone | 18–240 (3.0 : 80% at 2 weeks, 70% at 4 weeks, 60% at 6 weeks; 4.0 : 60% at 2 weeks, 40% at 4 weeks, 35% at 6 weeks) |
Synthetic nonabsorbable multifilament | ||
Ethibond | Polyester | |
Mersilene | Polyester/Dacron | |
Nurolon | Nylon | |
Synthetic nonabsorbable monofilament | ||
Ethilon | Nylon | |
Prolene | Polypropylene | |
Pronova | Polyvinylidene fluoride | |
Natural nonabsorbable multifilament | Perma‐hand (silk) | |
Natural nonabsorbable monofilament | Stainless steel (metallic) |
The absorbable sutures are broken down by phagocytes and are hydrolysed with no remaining material and vary depending on the length of time it takes the body to absorb the material: 30–40 days, 50–70 days, or > 100 days (Table 1.2.1). Nonabsorbable sutures will remain for a long time in the wound, and although they will eventually break up, residual foreign matter will remain in the tissue (e.g. Ethibond and Prolene). This remaining material may harbour microorganisms which may hinder phagocytosis or the action of antimicrobials and lead to wound infections or chronic infection, or they may get calcified.
There is a risk of sinus formation when nonabsorbable sutures are used during infection; hence, they are avoided in infection‐related procedures such as a nephrectomy of pyonephrosis.
When nonabsorbable sutures are chosen during surgery, one must bear in mind that monofilament sutures have the advantage over braided or twisted ones because they have a smaller surface area and no interstices which may harbour bacteria. This may reduce the risk of infection, although it cannot be eliminated entirely.
In the urinary tract, if a nonabsorbable suture is exposed to urine, it is almost inevitable that stones will form. There is also risk of stone formation even if absorbable sutures are used; however, it is significantly less than with nonabsorbable sutures [18, 19]. Even the most inert of metals (e.g. haemostatic clips) can cause stones to form [20]. Sutures placed right outside the urinary tract may, in the course of time, work their way like a cheese wire through living tissue until they come to lie within the urinary tract.
The tendency to form calculi does not seem to be related to the amount of histological reaction generated in the tissues. Even the most histologically inert materials (e.g. polyurethane, silicone, and hydrogels used for manufacturing double‐J stents) may be affected by encrustation and stone formation with deleterious consequences.
1.2.12.2 Synthetic Absorbable Suture Materials
The synthetic absorbable suture material made of polyglycolic acid (PGA) or polyglactin (a copolymer of glycolide and lactide) provokes less inflammatory response. The breakdown of these sutures is a result of chemical hydrolysis rather than biological phagocytosis. These sutures are relatively strong for their size and maintain their tensile strength long after the healing process and the tissue has reached its maximum tensile strength.
When absorbable sutures are used in the urinary tract, they have two possible disadvantages: (i) The suture may take a considerable period of time to hydrolyse and as it is in contact with urine, there is a chance that a stone may form; and (ii) urine may accelerate the hydrolysis of the suture material, especially in the presence of a Proteus infection [21, 22].
1.2.12.3 Meshes
Meshes are frequently used in urology to treat stress urinary incontinence and pelvic floor prolapse. It can be classified into synthetic or biological meshes. Synthetic meshes can be absorbable or non‐absorbable. Absorbable mesh is made of polyglactin (Vicryl) or polyglycolic (Dixon). Nonabsorbable mesh is made of polypropylene and mostly used in urological procedures because of their durability and strength.
The most important characteristic of synthetic mesh is pore size [23]. Synthetic meshes are classified according to their pore size into macroporous (>75 μm) and microporous (<10 μm) (Table 1.2.2). Macroporous mesh allows immune cells to travel through mesh structure and reduce risk of infection. It also incorporates well into the host tissue by promoting higher ratio of type I/III collagen [24].
Table 1.2.2 Mesh types, characteristics, and material.
Mesh type | Characteristics | Material |
Type I | Macroporous prostheses (pores >75 μm) | Prolene |
Type II | Microporous prostheses (pores <10 μm) | Gore‐Tex |
Type III | Macroporous prostheses with microporous components | Teflon |
Type IV | Submicronic pore size | Silastic |
A mesh originally used to support bladder neck is placed outside the urinary tract; however, it may migrate through the tissues into the urinary bladder causing chronic pain, urinary tract infection, and overactive bladder syndrome.
Biological meshes are primarily made of organic material of human, bovine, or porcine in origin such as dermis or pericardium. Biological mesh is treated to acellular extracellular matrix composed mainly of collagen with additional elastin and laminin. Growth factors within the mesh attract fibroblast. The three‐dimensional architecture and porosity of the mesh allow structural cells to enter the mesh and adhere to it. The mesh eventually degrades and is replaced with a collagen scaffold of the host tissue in a process called ‘remodelling’ [25].
1.2.12.4 Injectable Agents
Injectable agents are used to treat stress urinary incontinence and vesicoureteric reflux. Polytetrafluoroethylene (PTFE, or Teflon) was first introduced in 1973 [26]. More recently, various agents have been used as a bulking agent. Injectable bulking agents can be classified into biological or synthetic. The most commonly used biological agents are collagen. Synthetic agents that are currently in use include hyaluronic acid dextranomer (Deflux) and polyacrylamide hydrogel (Bulkamid). The ideal injectable agent should be easy to prepare and inject, biocompatible, non‐imunogenic, non‐carcinogenic, and does not contract with time [27]. Glutaraldehyde cross‐linked (GAX) collagen bulking agent has been demonstrated to be safe and efficacious in the short and intermediate term [28]. After injection, it attracts host immune cells and fibroblasts and encourages neovascularisation. Ultimately, the remodelling process results in the replacement of the injected collagen with the patient’s own type I and III collagen [29].
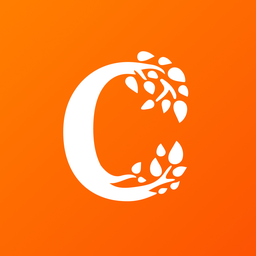
Full access? Get Clinical Tree
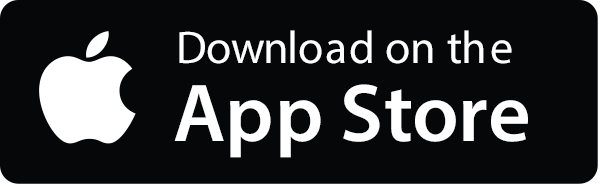
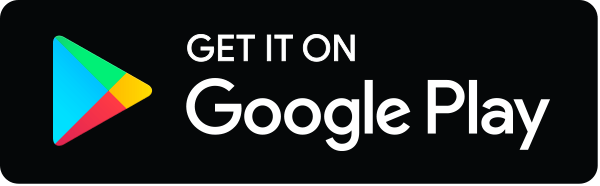