Outline
Introduction 38
Genetic Modification In Vivo 38
Transgenic Expression 38
Manipulation of the Mouse Embryo 38
Pitfalls in Transgenic Mice and the Development of the Gene Trap ROSA26 Locus 39
Pitfalls to the ROSA26 Locus 39
Coalescence of Ubiquitous Transgenic Gene Expression Under the CAGGS Promoter 39
Diphtheria Toxin Systems for Cellular Ablation In Vivo 40
Recombineering and the Advent of Genetic Mutation by Knockin and Knockout Approaches 42
Genome Modification by Site-specific Recombinases 42
Inducible Systems and Pitfalls 42
Fluorescent Proteins, Their Development and Use in Mice 43
Pitfalls to In Vivo Genetic Manipulation 44
Mouse Strain 44
Mosaicism 45
Cellular Lineage of Gene Activation 45
Development of the Nephron: How Genetic Tools have Enabled Understanding of Nephron Formation 45
Nephron Formation During Kidney Organogenesis 45
A Multipotent Self-renewing Nephron Progenitor Population 46
Nephron and Interstitium Compartments During Kidney Organogenesis 46
Formation of Other Cell Types in the Kidney 47
Adult Kidney Regeneration: How Genetic Tools have Enabled Understanding of Nephron Regeneration 47
Kidney Repair and Regeneration: A Lack of Model Systems 47
Kidney Repair and Regeneration: The Ischemia–Reperfusion Model 47
Bone Marrow Chimerism in Mouse Kidney Leukocyte Lineage Tracing: The Origin of Regenerating Cells 49
Lineage or Fate Tracing in the Mouse Kidney: Origin of Regenerating Epithelial Cells 52
Cellular Ablation in the Mouse Kidney: Role of Myeloid Cells in Organ Repair and Regeneration 53
Myeloid Cells in Organ Repair and Regeneration 53
Podocyte Regeneration 57
Gene Mutation in the Study of Mouse Kidney Repair and Regeneration 60
Wnt Signaling Pathway Activation 60
Overlap Between Polycystins, Cyst Formation and Epithelial Repair 60
Sphingosine-1 Phosphate Receptor Deficiency in Renal Repair 61
Lessons from Generation of the Kidney: Can We Use Recent Insights and Developmental Tools to Regenerate the Kidney? 62
Genetic models to study kidney disease in mice are now commonplace. Recently, such mouse models have been used to study kidney disease and regeneration, and have provided powerful insight into disease mechanisms and therapeutic options. However, despite the power of such studies they are complex and there are many hidden pitfalls. This chapter will discuss the underlying principles at play in mouse models, show the power of the technology and highlight key advances in regenerative medicine that have come from such technologies.
Introduction
Since the development of the first transgenic mice there has been an explosion of knowledge and experience in the use of genetic manipulation to test gene function in vivo in mice. These technologies have proved to be tractable and powerful tools with significant advances over in vitro studies that were the mainstay of basic sciences. Genetic manipulation in mice has proven to have greater relevance to human disease and greater reproducibility, and has enabled functional studies at a level of sophistication not previously possible. Alongside this explosion of genetic tools in mice, has been the development of an array of mouse models of human conditions including kidney disease and regeneration. Regenerative nephrology, a new field in nephrology, has emerged from these technological advances. This chapter will explain some of the underlying technologies that are in common usage, highlight key areas of technological advance using these methods, and show key areas of pitfalls that may not be readily apparent to a reader.
Genetic Modification In Vivo
Transgenic Expression
Transgenesis is the introduction of foreign DNA from another organism. Since the advent of molecular biological techniques in the 1970s we have been able to use bacteria and subsequently mammalian cells to synthesize proteins. To achieve this the coding sequence for the gene of interest is placed alongside a promoter sequence that is active in the host bacteria or cell in a small circular DNA commonly found in bacteria, called a plasmid. This plasmid is not part of bacterial genomic DNA, but functions in bacteria as a stable source of DNA and is replicated and inherited like genomic DNA during cellular replication. Plasmids can easily be transferred into bacterial cytoplasm. Plasmids can also be persuaded to enter mammalian cell cytoplasm transiently, particularly in transformed cell lines that proliferate well in culture. Bacterial plasmids have been genetically modified to contain key elements of mammalian promoters/enhancers that express at high levels in many types of cells and also contain the 3′ untranslated region (UTR) of genes such as globulin. By placing the coding region or open reading frame of a gene of interest between the promoter/enhancer region and the 3′ UTR of a typical mammalian gene in such a plasmid, and introducing copies of that plasmid into the cytoplasm of mammalian cells it became common practice to transiently generate the protein encoded in that plasmid by the cell that accepts it. Although plasmids do not remain in mammalian cell cytoplasm for more than a few days, linearized plasmid occasionally integrates into the genomic DNA, resulting in stable production of protein by the cell line. Since the establishment of this key advance in molecular biology, many modifications have been made to the development of stable gene expression in mammalian cells. For example, whereas the coding mRNA lacks introns, mammalian genomic DNA contains many introns. Transgenic stable expression of genes in mammalian cells using plasmids may result in progressive silencing of the transgene or removal of the sequence after weeks or months. However designed, introduction of an intronic sequence into the plasmid results in enhanced stable gene expression . Manipulation of the standardized 3′ UTR, downstream to the open reading frame, and also the promoter/enhancer regions upstream has resulted in high-level stable expression of many genes in cell lines .
Manipulation of the Mouse Embryo
The first transgenic animal was created using mice. With the advent of molecular biological techniques, Ralph Brinster injected DNA in the pronucleus of forming zygotes and observed transgene expression in maturing zygotes . In 1982, several groups reported the first germ line transmitting transgenic mice by DNA injection into the pronucleus of the zygotes, one-cell stage fertilized embryos . The team coupled the coding sequence for the enzyme thymidine kinase or the sequence for rat growth hormone, under regulation of the metallothionein promoter.
Around the same time, Martin Evans (UCL) and Matthew Kaufman (Harvard), and independently also Gail Martin, established embryonic stem (ES) cells from mouse blastocysts and grew them in culture in 1981 ( Fig. 3.1 ). ES cells can be cultured indefinitely in vitro when cultured in the presence of feeder cells that provide crucial growth factors, including leukemia inhibitory factor (LIF). These cultured ES cells are also susceptible to the introduction of DNA-containing transgenes by transfection techniques such as electroporation, in which cells are transiently damaged by electric shock treatment that punctures holes in the cytoplasm allowing diffusion of extracellular DNA into the cell, before the plasma membrane repairs itself. Incorporation of linearized plasmid into the genomic DNA of ES cells results in transgenic stem cells, which can be placed in a mouse blastocyst and returned to a pseudopregnant mouse uterus where implantation and embryonic and fetal development proceed . It is possible to generate completely ES cell-derived embryos using tetraploid complementation or eight-cell stage embryo injection .

Pitfalls in Transgenic Mice and the Development of the Gene Trap ROSA26 Locus
By the simplified methods described the first transgenic mice evolved. All mice were initially developed predominantly on 129/Sv inbred background since mouse ES cells are traditionally isolated from the 129/Sv strains. This strain restriction has important implications for the interpretation of phenotype (see below) . Incorporation of transgenes into mice is associated with problems. The first is the integration site. The transgene incorporates randomly into the genomic DNA. There may be multiple copies (> 50) often in head-to-tail tandem. The local genomic environment of the transgene may have a profound impact on the fidelity of expression. Therefore, despite the use of promoter and enhancer the pattern of gene expression may not truly reflect the expression of gene under the endogenous promoter. The insertion may disrupt or modify endogenous gene expression. Nevertheless, despite these pitfalls, transgenic mice rapidly became established as a tool to study in vivo gene overexpression or de novo expression of foreign or mutated genes, or aberrant expression of genes in cells that would not normally express the gene. In part to circumvent the problems of mosaicism, but also to standardize the insertion of the transgene into the genomic DNA and to standardize ubiquitous expression under the same promoter enhancers, the gene trap system was developed, also known as gene trap ROSA26. Soriano and co-workers were studying random integration of a retrovirus driving the open reading frame for the gene βgeo (known as gene trap) into the mouse genome in ES cells. This virus, reverse orientation splice acceptor (ROSA), integrated at many sites but one clone, numbered 26, resulted in expression of βgeo (βgal, neomycin resistance fusion protein) in all tissues in the mice that resulted from the virally infected ES cells ( Fig. 3.2 ). The ROSA26 site was thought to represent a housekeeping gene but although the endogenous promoter at the ROSA26 locus generates transcripts, no proteins are synthesized. The ROSA26 locus has been extremely carefully characterized for ubiquitous activity in the mouse, and following sequencing of the locus a targeting vector that contains 5′ and 3′ regions of the ROSA26 locus was generated that enables insertion of any gene of interest through homologous recombination at this site . Insertion of an open reading frame of a gene of interest at this site leads to ubiquitous expression in all cells in all tissues and has become the standard site to express any gene of interest. This system of overexpression under endogenous regulators of gene expression has proven extremely tractable and heralded further advances in technologies that target gene expression .

Pitfalls to the ROSA26 Locus
First, expression levels of the gene product in adult mice are significantly lower than in embryonic or fetal mice , and therefore may not be useful in adult tissues if levels of gene expression above a certain threshold are required. Second, the ROSA26 locus may not be amenable to Cre-mediated recombination at high levels in adult mice. It may be that the ROSA26 locus becomes increasingly inaccessible in certain types of cells following development, possibly owing to chromosomal structure modifications .
Coalescence of Ubiquitous Transgenic Gene Expression Under the CAGGS Promoter
The chicken β-actin promoter coupled to the cytomegalovirus (CMV) early intermediate minimal enhancer followed by an intron (CAGGS promoter) can yield high-level transgene expression in the mouse . Single-copy incorporation of this transgenic construct into genomic DNA can lead to widespread stable expression of the gene of interest at high levels in many cell types . Despite the use of this system for universal overexpression the transgenic approach remains imperfect. In contrast to ubiquitous expression of genes under the ROSA promoter/enhancers, transgenic expression under short promoter DNA often is not ubiquitous and uniform within tissues and between transgenic lines. This mosaicism of patchy expression levels depending on random insertion of the transgene remains a potential problem for such mouse models using this system .
Recent novel strategies combining the strengths of the ROSA26 locus ubiquitous expression and CAGGS promoter/intron system for high-level expression by inserting CAGGS-driven genes at the ROSA26 locus promise high-level ubiquitous transgenic expression in vivo in a “plug and play” fashion that will enable high-throughput screening of gene function in any given tissue or cell type .
Diphtheria Toxin Systems for Cellular Ablation In Vivo
Diphtheria toxin (DT) is a protein produced by Corynaebacteria diphtheriae . It binds to the heparin-binding epithelial growth factor receptor (EGFR) in human cells enabling its endocytosis and entry to the cytoplasm of subunit A (DTA), where it binds to and inactivates the ribosomal protein eEF-2, disabling the translational machinery which triggers apoptotic cell death rapidly. In humans, whooping cough, a disease of the upper respiratory tract caused by C. diphtheriae , frequently results in lethality owing to release of toxin in the upper respiratory tract and circulatory delivery to the myocardium and other vital organs. One milligram of purified DT is lethal to humans. Rodents, including mice, however, have a form of EGFR that does not bind DT and are therefore completely resistant to the toxic effects of extracellular DT ( Fig. 3.3 ). Transgenic expression of the catalytically active subunit DTA intracellularly has been used by molecular biologists in cellular ablation . Initially this technique was used to delete stem cells that had not incorporated a transgene faithfully, but transgenic expression of DTA under a cell-specific promoter leads to ablation of any cell that transcribes the transgene. Similarly, transgenic expression of the human heparin-binding EGFR, which is the diphtheria toxin receptor (DTR), in mouse cells, renders those cells susceptible, like human cells, to extracellular exposure of DT . Transgenic expression of DTR selectively in one of a restricted number of cell types can yield a mouse in which injection of DT into the circulation results in uptake of the DTA subunit only into cells expressing the transgene and rapid loss or ablation of the specific cell-type . The use of these systems was first reported by Evans in 1989, and Breitman in 1990 . However, one of the first successful uses of this model system in mice was reported by Lang and Bishop in 1993 . In these studies expression of DTA was transgenically driven by a promoter created by the fusion of a portion of a viral promoter and part of the granulocyte–macrophage colony-stimulating factor (GM-CSF). This resulted in restricted expression of the transgene only in macrophages in the peritoneal cavity and the eye, and also inflammatory macrophages and these populations of macrophages were absent. The resultant surviving mice carried a very distinctive phenotype, in the eye. The hyaloid microvasculature of the developing eye, which normally regresses following delivery of newborns, failed to regress in the absence of macrophages in the eye ( Fig. 3.4 ). This novel method was the first genetic system to study macrophage function in vivo by cellular ablation and showed that a major function of macrophages is in tissue remodeling.


Additional ablation systems are available and have been used successfully, including the expression of viral thymidine kinase (Tk) in cells that are then susceptible to the toxic effects of the drug gancyclovir . Unlike DT, Tk ablates only dividing cells.
Recombineering and the Advent of Genetic Mutation by Knockin and Knockout Approaches
With the identification of the ROSA locus, which affords universal cellular expression of a gene of choice placed at that locus, it was obvious that inserting a gene of interest under any other endogenous promoter and regulatory sequences would be possible. All that is required is the sequence of the gene, including exons and introns, and the sequences of the promoter upstream and downstream for approximately 2000 bp. However, to modify genomic DNA at will, several key hurdles had to be overcome: first, the capacity to sequence large regions of DNA around the gene of interest; second, the capacity to synthesize a copy of this region of genomic DNA with strategic alterations; and finally, the capacity to “persuade” genomic DNA to be replaced by the constructed DNA sequence ( Fig. 3.5 ). Initially, sequencing of genomic DNA was acquired by tedious sequencing upstream and downstream of the start site of the gene of interest. A length of DNA was then copied from the genomic DNA of the region upstream and downstream of the coding region. One of the exons of the gene was selected and deleted from the copied piece of DNA, usually resulting in a frame shift or premature stop codon in the coding region. This synthesized piece of DNA, which corresponds to the gene except for the absence of one of the exons, when placed in a dividing cell undergoes homologous recombination. The first mouse in which a gene was disrupted in the genomic sequence by this method was reported in 1989 independently by Capecchi, Evans, Smithies and their respective colleagues . Genomic DNA undergoes homologous recombination during meiosis, but bacteria and eukaryotes have endogenous systems to repair dsDNA and ssDNA breaks. By relying on endogenous homologous recombination systems in ES cells and a combination of negative and positive selection ( Fig. 3.5 ), spontaneous recombination at the homologous sites will result in insertion of the engineered construct at the specified site in the genomic DNA in a minority of cells that then become resistant to a positive selection.

Genome Modification by Site-specific Recombinases
Site-specific recombinases are widely used to genetically manipulate the mouse genome. These recombinases include Cre, FLP and ΦC31, which recognize specific loxP, FRT and att site sequences, respectively. These recombinases, initially described in bacteriophage viruses and yeast, do not require cofactors to catalyze DNA recombination in eukaryotic cells, which simplifies transgenic designs . The site-specific recombination allows removal, insertion and inversion of specific DNA sequences in the genome. These tools have been applied to genetic recombination techniques with high levels of success, enabling tissue-specific gene activation or gene mutation (conditional gene deletion) .
Inducible Systems and Pitfalls
For temporal gene expression, tetracycline-regulated gene expression system is most commonly used in the mouse , which was developed by Hermann Bujard in 1992 . In the Tet-Off system, in the absence of doxycycline, tetracycline transcriptional activator (tTA) binds to tetracycline operator (tetO) DNA elements and activates transcription of transgene downstream of CMV minimal promoters adjacent to tetO. After doxycycline administration, doxycycline-bound tTA can no longer bind to tetO, which leads to repression of transgene expression. In contrast, in the Tet-On system, in the absence of doxycycline, the reverse tetracycline transcriptional activator (rtTA) cannot bind DNA. After doxycycline administration, doxycycline-bound rtTA binds to tetO, which activates transgenes downstream of adjacent CMV minimal promoters. The critical consideration for the tetracycline-regulated gene expression system is to place tetO-CMVmin transgenes in neutral loci in the genome so that there is no leaky transgene expression when their transcription is not activated by the tetracycline system and there is a high level of transgene expression when activated by the tetracycline system. It is also noted that the tetracycline system may activate neighboring genes adjacent to tetO elements . GAL4-UAS systems have also been successfully used in the mouse , although this system has been most widely used in Drosophila fly studies. These bigenic systems, where the promoter and the transgene are separated, have proven useful especially when transgene expression causes lethality and infertility, which prevents maintenance of transgenic lines.
Recombinases fused with a subunit of the estrogen receptor (ER) have become widely used to temporally regulate recombination in the mouse. The nuclear localization subunit of the cytoplasmic ER requires estrogen binding to translocate the receptor into the nucleus where the DNA binding subunit can regulate transcription. The capacity for compartmentalizing proteins to cytosol or nucleus is very attractive for temporal recombination since recombinases need to be in the nucleus to function, so a recombinase fused to the ER (nuclear localization subunit) will remain in the cytosol and be functionally inactive in the absence of estrogens. Unfortunately, endogenous estrogens and other factors have rendered such approaches “leaky” until recently. A mutated form, ER T2 , is widely used owing to its complete dependence on the estrogen-mimetic drug tamoxifen for nuclear translocation. In the absence of tamoxifen, ER T2 retains fusion proteins in the cytoplasm. Upon tamoxifen administration, tamoxifen binds to ER T2 . The tamoxifen-bound ER T2 allows translocation of fusion proteins into the nucleus, where recombinases can recombine target sites in DNA.
Fluorescent Proteins, Their Development and Use in Mice
Green fluorescent protein (GFP), an endogenous protein of the jellyfish Aequorea victoria , exhibits green fluorescence in response to blue light. Its calcium-dependent fluorescent properties were described by Osamu Shimomura . It was originally cloned in 1992 by Douglas Prasher. However, it was first exogenously expressed by three teams in bacteria and worms and found to fluoresce well, outside of jellyfish ( Fig. 3.6 ). This remarkable breakthrough in fluorescent protein technology was awarded the Nobel Prize (2008) and has heralded unprecedented advances in biomedical sciences . Following the determination of its crystal structure, the original GFP protein has undergone numerous modifications (beyond the scope of this text) by directed mutagenesis to yield eight different fluorophores: enhanced GFP, blue fluorescent protein (BFP), cyan fluorescent protein (CFP), citrine and yellow fluorescent protein (YFP). Red fluorescent protein (RFP) was cloned from the Red Sea coral Discosoma sp. and is also known therefore as DsRed . It fluoresces red with green light. Although it was successfully cloned as a partner to the GFP variants, it was tetrameric, very slow to mature and highly toxic to cells. Through directed mutagenesis requiring 33 substitutions, a monomeric form, mRFP1, was initially described, and this was improved to yield three monomeric proteins that could be linked to other proteins, called RedStar and mCherry and mOrange . tdTomato, a dimer, is brighter but less amenable to linking to other proteins. mCherry has perhaps gained most traction as a complementary fluorophore to use in vivo in mice . Fluorescent proteins with a variety of colors have been generated and successfully used in the mouse . These fluorescent proteins also function without cofactors and function at 37°C in mammalian cells, either fused to other proteins via a linker or as unlinked proteins. Monomeric fluorescent proteins, including eGFP and mCherry, are therefore useful to tag proteins of interest. Modified forms are directed to different cellular organelles, plasma membrane or nucleus. Alternatively, these fluorophores have been used in vivo to label recombination events or cell signaling activity or for lineage tracing ( Fig. 3.7 ).


Pitfalls to In Vivo Genetic Manipulation
Mouse Strain
Thanks to fanciers in Japan and England in the eighteenth and nineteenth centuries, followed by fanciers and biologists, primarily located in Massachusetts, interested in Mendelian inheritance in the later nineteenth and early twentieth century (Lanthrop and Castle), we now have at our disposal many strains of inbred mice. These mice strains have been bred in excess of 150 times in brother × sister matings and are essentially homozygous at all loci. Moreover, each inbred strain is essentially isogenic (genetically identical). Mice are broadly derived from four species/subspecies: Mus musculus molossinus (Japan), Mus musculus domesticus , Mus musculus castaneus and Mus spretus . The period before World War I also led to the initiation of inbreeding in rats by Dr Helen King in about 1909 and in mice by Dr C. C. Little in 1909.
The latter project led to the development of the DBA strain of mice, now widely distributed as the two major substrains, DBA/1 and DBA/2, which were separated in 1929–1930. DBA mice are selected for coat color (d dilute, b brown and a agouti). Soon after World War I, inbreeding in mice was started on a much larger scale by Dr L. C. Strong, leading in particular to the development of strains C3H and CBA, and by Little, leading to the C57 family of strains (C57BL, C57BR and C57L). Many of the most popular strains of mice were developed during the next decade, and some are closely related. Evidence from the uniformity of mitochondrion DNA suggests that most of the common inbred mouse strains were probably derived from a single breeding female about 150–200 years ago . The mice were bred for specific purposes: C57BL/6 had increased preference for alcohol and narcotics; FVB mice had large pronuclei and were useful for direct gene transfer into fertilized eggs; and 129 ES cells were selected because of high levels of success in germline transmission. Other mice spontaneously developed tumors.
Phenotypic differences between inbred strains need to be taken into account when designing and interpreting experiments. Strains may vary in characteristics that may not be relevant directly to the phenotype studied but which may nevertheless influence experimental results. The genealogy of mouse strains is both informative and important in experimental design. The genealogy tree is available from ftp://www.informatics.jax.org/pub/datasets/misc/genealogy/genealogy.pdf . For example, with respect to the kidney, different strains of mice have differing susceptibility to kidney diseases, from glomerulonephritis, through diabetic nephropathy to ischemia–reperfusion injury (IRI). All studies comparing wild-type mice with mutants must be on the same genetic background. If the mice are on mixed backgrounds, additional controls should be performed for strain combination differences. The extent of this genetic variation was exhibited most clearly in studies of mutation of the protein serum amyloid P/pentraxin-2. Mutant mice developed by targeted mutation of 129 ES cells grown in C57BL6 embryos developed a spontaneous autoimmune phenotype. However, the strain-matched controls also had a mild autoimmune phenotype. This may be because the segment of the chromosome where pentraxin-2 resides is in the Sle1 susceptibility region of chromosome 1. Selection for 129 Sle1 in C57BL/6 mice predisposes to autoimmunity .
Mosaicism
Mosaicism is the presence of two heterogeneous genetic types within an individual. Therefore, tissue-specific recombination is mosaicism by definition. The term is often used to describe lower recombination within target tissues where Cre recombinase is expressed. The degree of recombination largely depends on levels of Cre expression and recombination efficiency at target sites. To achieve recombination, a certain level of Cre protein accumulation is required. Also, as described above, different tissues have different accessibility of recombinases to target sites in the genomic DNA, and this may be context, disease or temporally dependent. In addition, owing to the limited time activation, inducible recombinases give lower recombination efficiency. To overcome this type of mosaicism, multiple doses of tamoxifen or tetracycline are required to obtain more complete recombination in target tissues.
Cellular Lineage of Gene Activation
Although the expression of collagen1α1 gene is highly restricted in the adult mouse, the endogenous gene is activated in the blastocyst. This is a serious consideration in designing gene-targeted mice using this locus for analysis. Although expression of a gene such as GFP under the collagen promoter identifies cells and denotes function, activation of a gene permanently or inactivation of a gene permanently under the regulated expression of collagen1α1 would yield no specificity since it is activated in many cells early in embryonic development. With the explosion of in vivo reports of gene function and cell lineage using Cre recombinase to mutate or activate a gene in the genomic DNA, this problem of cell specificity may be rife and extremely opaque to both the investigators and readers. Although Cre may be active only in one cell type in the adult, developmentally it may be active in other cell types and results in genomic recombination (activation or inactivation of the gene or interest) in cell types other than those known to the investigator, and therefore the readers. One such example is the use of the Tie2 promoter to drive Cre. Tie-2 is an endothelial receptor for angiopoietins. Like many other endothelial receptors it is also expressed by leukocytes. In mice, all myeloid cells in the bone marrow transiently express Tie-2 and a subpopulation of myeloid leukocytes continues to express Tie-2. The result is that Cre driven by Tie-2 will recombine genomic DNA in both endothelial cells and myeloid leukocytes .
Development of the Nephron: How Genetic Tools have Enabled Understanding of Nephron Formation
Nephron Formation During Kidney Organogenesis
The nephron is the basic function unit of the kidney. A kidney contains about 100,000 nephrons in humans and 13,000 in mice. After formation of germ layers during gastrulation, the nephric duct is formed along the intermediate mesoderm of embryos around day 8 in the mouse ( Fig. 3.8 ). At the onset of kidney organogenesis around day 10 in the mouse, the ureteric bud emerges at the posterior end of the nephric duct. Formation of the ureteric bud is induced by signaling from the adjacent metanephric mesenchyme. This tissue interaction largely depends on glial cell line-derived neurotrophic factor (GDNF) and its receptor Ret in the nephric duct. Ectopic expression of GDNF is sufficient to induce ectopic ureteric bud formation at the anterior region of the nephric duct.

Through the reciprocal interactions between the ureteric bud and metanephric mesenchyme, a portion of the metanephric mesenchyme condenses to form the cap mesenchyme. The nephrogenic interstitium (cortical stroma) also arises at this stage. GDNF-Ret signaling continues to be required during kidney organogenesis. The cap mesenchyme and ureteric tip express GDNF and Ret, respectively. The GDNF-mediated tissue interaction between the cap mesenchyme and ureteric tip is essential for ureteric branching, which forms the collecting duct system of the kidney ( see Fig. 3.8 ).
As the ureteric tip branches, a nephron precursor called the pretubular aggregate forms at the newly formed ureteric tip. The pretubular aggregate undergoes mesenchyme-to-epithelium transition (MET) to form an epithelial sphere, the renal vesicle. The renal vesicle undergoes morphological changes to differentiate into the comma-shaped body, S-shaped body and eventually the nephron epithelia. The induction of the nephron also depends on tissue interactions. The ureteric tip-derived Wnt9b induces formation of the pretubular aggregate in mesenchymal tissue. In the mesenchymal tissue, β-catenin is required, suggesting that Wnt9b from the ureteric tip activates the canonical Wnt signaling mediated by β-catenin in the mesenchyme.
A Multipotent Self-renewing Nephron Progenitor Population
Recently, genetic studies have identified nephron progenitor cells. Cited1 and Six2 are exclusively expressed in the cap mesenchyme of the developing kidney. Using Cited1-CreER T2 and Six2-eGFPCreER T2 , it was shown that the cap mesenchyme is a multipotent self-renewing nephron progenitor population ( Fig. 3.8 ) . Fate map analysis using the Cre-loxP system showed that the cap mesenchyme contributes to all cells in the nephron epithelia. Using a genetic label retention assay, evidence has been provided that the cap mesenchyme is maintained by self-renewal. Lastly, clonal analysis indicated that a single cap mesenchyme cell can differentiate into different cell types of the nephron epithelia.
Thus, the presence of the cap mesenchyme allows repetitive formation of the complement of numerous nephrons in the kidney. The Six2+ population of progenitor cells in the mammalian kidney disappears by 5–7 days postpartum, and after this time no new nephrons are formed. Further, Six2-expressing cells have not been identified in adult kidney in health or following injury .
Nephron and Interstitium Compartments During Kidney Organogenesis
In kidney disease, nephrons are lost and the fibrotic interstitium expands. Therefore, identification of molecular mechanisms for specification of the nephron and interstitium, respectively, during kidney organogenesis is crucial to understand and cure kidney disease in adults. A forkhead family transcription factor, Foxd1 (also known as Bf-2), is expressed in the nephrogenic interstitium (stroma) which overlies the cap mesenchyme in the developing kidney ( Fig. 3.8 ). By generating Foxd1-Cre mice, it was found that the cortical and medullary interstitium are derived from the nephrogenic interstitium (A. Kobayashi, unpublished data) and these cells mature into pericytes, vascular smooth muscle and mesangial cells of the adult kidney, but not resident macrophages or endothelium . These Six2-Cre and Foxd1-Cre alleles are useful tools to genetically manipulate the nephron and surrounding interstitial tissues in the kidney. Fate mapping of these nephron and interstitial tissues revealed cellular regulation during kidney disease and repair (see below). In addition, these Six2-Cre and Foxd1-Cre alleles allow inactivation or activation of certain genes in a specific compartment of the kidney. This will clarify signaling pathways for tissue interactions in the kidney. For example, collecting duct-derived Wnt7b signaling was shown to be mediated by β-catenin in the interstitium during kidney organogenesis .
Formation of Other Cell Types in the Kidney
Developmental processes for several tissues in the kidney remain poorly described. These include the vasculature and neurons in the kidney. The vasculature is an important component of the kidney, and increasing studies point to its central role in both nephrogenesis and nephron regeneration after injury . However, little is known about how the vasculature develops during kidney organogenesis. One possibility is that it derives from angioblasts originating from blood islands or the aorta–gonad–mesonephros (AGM) which migrate into the metanephric mesenchyme at about the same time as the ureteric bud invades this condensing mesenchyme. Fate mapping studies of AGM-derived endothelium or blood island-derived endothelium are underway and hopefully will answer this question soon. The other possibility is that a third condensate of peripheral metanephric mesenchyme lying peripheral to Six2+ cap mesenchyme and Foxd1+ interstitial mesenchyme has a distinct vascular lineage. Expression of the transcription factor Osr1 occurs in intermediate mesoderm cells that become Six2+ nephron progenitors and Foxd1+ interstitial progenitors. Osr1+ cells of the condensing metanephric mesenchyme may also become kidney angioblasts . Further studies in this area are required and may prove beneficial for understanding both developmental kidney diseases and regenerative medicine of the adult kidney.
Adult Kidney Regeneration: How Genetic Tools have Enabled Understanding of Nephron Regeneration
Kidney Repair and Regeneration: A Lack of Model Systems
Unlike many other organs, units of the kidney, nephrons in mammals, are not regenerated. If a nephron becomes sclerotic or absolescent it is not replaced. This fact sets the kidney apart from organs such as liver in which lobules, or functioning units of liver, can be completely regenerated, and muscle, where whole myo-units can be regenerated. Nevertheless, if the nephron and its proximal blood supply remain intact the distal portions of the nephron from proximal tubule onward can be successfully regenerated. Despite widespread loss of epithelial cells in the kidney near complete regeneration can be achieved ( Fig. 3.9 ). Aquatic, non-mammalian vertebrate kidney, which includes many orders of fish, is different. Young kidneys in elasmobranchs and teleosts (fish) can regenerate entire nephrons from precursor cells in a manner highly reminiscent of developmental nephrogenesis in the mammal . Perhaps, since there is no mammalian nephrogenesis following injury, many of the model systems used in mice are not true regenerative models. Following mammalian kidney injury there is progressive fibrosis and chronic kidney disease which either stabilizes with hypertrophy of surviving nephrons or progresses toward near complete nephron loss.

Kidney Repair and Regeneration: The Ischemia–Reperfusion Model
This model system, initially developed in rats but now widely adopted in mice, can be singled out as a repairing/regenerative model of the kidney. The model requires clamping of both renal arteries for a defined period while the kidney is maintained at body temperature. Following release of the clamps the organ is immediately returned to the body cavity to maintain temperature. One feature of this model is that there is minimal injury to the glomerulus, therefore all the proximal structures of the nephron and the origin of the nephron blood supply remain following injury. It is perhaps for this reason that repair and regeneration are effective. The model is very temperature sensitive, and sensitive to the strain of the inbred mice used for experimentation. For this reason reproducibility from laboratory to laboratory has been lacking.
The IRI model has yielded significant information about the cellular components of repair by straightforward cell biological studies. Ischemic injury (and many toxic injuries) primarily injures proximal tubule epithelium of the outer medulla ( Fig. 3.10 ). Injury is widely held to be maximal here because of a combination of high aerobic metabolic demand and a sluggish subcortical blood supply which characterizes the nephron. These epithelial cells undergo predominantly necrotic death. Other segments of the nephron are more likely to experience milder injury with apoptotic death . In addition to the obvious epithelial injury there is an underappreciated injury and loss of the peritubular capillary network ( Figs 3.9, 3.10 ). From 2 days to 15 days the lost and damaged portions of the nephron regenerate, as do the peritubular capillaries. However, although there is complete functional recovery from severe (> 90%) functional loss, there is never complete capillary recovery or nephron recovery . Moreover, the regeneration of the peritubular capillary plexus remains incomplete . In severe examples of this model or a unilateral version of this model, following functional recovery a syndrome of progressive loss of tubular function or even progressive nephron dropout ensues .

Although there are other toxic injury models, including sepsis triggered by cecal puncture in aged mice, and toxins which trigger death of epithelial cells of the proximal nephron, including gentamicin, carbon tetrachloride or cisplatin, these generally produce either very mild disease or progressive disease. There are reports, however, of these models successfully being used to study regenerative capacity . Understanding why the IRI model predominantly repairs whereas other models characterized by epithelial injury do not may yield key information about mechanisms that promote repair compared with those that result in chronic kidney disease. Recent reports indicate that the obstructive model of kidney disease which results in progressive parenchymal disease is reversible and this reversal results in nephron regeneration . The prospect of such a model to study both progression and reversal in a single disease model is tantalizing, but those findings have yet to be reproduced in the literature.
Bone Marrow Chimerism in Mouse Kidney Leukocyte Lineage Tracing: The Origin of Regenerating Cells
To understand the contributions of cells derived from the bone marrow to kidney regeneration, bone marrow transplantation has been employed. It is straightforward to replace bone marrow stem cells completely in rodents by lethal irradiation followed by intravenous infusion of bone marrow stem cells from a donor. Bone marrow transplantation has been used for more than 30 years to trace cells derived from bone marrow stem cells . If the donor stem cells are different from the recipient then cells deriving from bone marrow stem cells can be detected permanently. Permanent genetic or molecular markers of bone marrow stem cells include male stem cells harboring a Y chromosome in female mice, transgenic expression of a marker such as RFP or GFP under a universal promoter, or expression of a marker at the endogenous ROSA26 locus. Other markers include the CD45.1 compared with CD45.2 variant which can be detected readily using specific antibodies. However, CD45 is expressed by leukocytes but may not be expressed by derivatives of hematopoietic stem cells (HSCs) that differentiate into non-hematogenous cells. Three publications supporting the hypothesis that following ischemic injury the proximal tubule cells are regenerated by incorporation of cells derived from bone marrow that differentiate into epithelial cells were published between 2002 and 2003 . Two of the studies used LacZ expressed at the ROSA26 locus as the marker of bone marrow cells and one of the studies used the Y chromosome as the fate marker. These studies were published around the time of a range of studies using fate markers of bone marrow HSCs that reported organ regeneration by differentiation of HSCs into myocytes (in muscle), cardiomyocytes (in heart) and a number of other cells in other organs. However, many of the studies were flawed. In subsequent follow-up studies it was not possible to reproduce the findings. In fact, detection of LacZ in the adult kidney is fraught with problems. The bacterial LacZ gene generates β-gal, an enzyme whose activity can be used to generate a blue stain using X-gal solution. LacZ expression can be detected by this blue colorimetric assay. The kidney proximal tubule (the compartment under investigation in these studies) has endogenous mammalian β-galactosidase . Its enzyme activity can also trigger blue stain using X-gal solution. Mammalian, endogenous β-gal activity is maximal at low pH owing to its intracellular compartment, and is minimal at pH > 7.4 ( Fig. 3.11 ). By contrast, bacterial β-gal activity is high at neutral or alkaline pH. Therefore, careful attention to tissue pH during staining is a prerequisite for detecting the LacZ fate marker but not endogenous β-gal activity. The early studies showing blue-stained tubules in the kidney of bone marrow chimeras carrying LacZ in HSC-derived cells were not reproduced, probably as a result of this artifact.
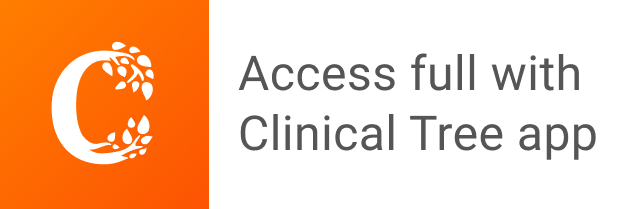