75 Domenico Veneziano1 & David M. Hananel 2 1 Department of Urology and Kidney Transplant, Grande Ospedale Metropolitano “Bianchi, Melacrino, Morelli”, Reggio Calabria, Italy 2 Center for Research in Education & Simulation Technologies, University of Washington, School of Medicine, Seattle, WA, USA “See one, do one, teach one” has been the mantra of surgical training for decades. Unfortunately, when we ask where are we going to see, do, and teach, the answer is often still “the patient.” In its early days, laparoscopy was considered too challenging and surgeons asked “why work through a keyhole when you can just walk through the door?” [1]. Indeed, operating through a keyhole adds even more complexity to surgery: fulcrum effect, decreased haptic feedback, and loss of depth perception, to name a few [2]. Despite these challenges, the early 1990s saw rapid acceptance of laparoscopy driven by patient demands: reading and hearing about faster recovery, less pain, and less scarring. The field was caught by surprise and device companies started to provide training courses led by the early adopters. A weekend course was often considered enough by many surgeons to try this new technology at their home institutions. There was no standardized curriculum established and training was performed mainly on porcine models. The desire to overcome some of the challenges in laparoscopy and an interest in remote surgery to perform operations in underserved or dangerous venues led to research in robotically assisted surgery. These efforts were funded by Defense Advanced Research Projects Agency (DARPA; Arlington, VA, USA) and the National Aeronautics and Space Administration (NASA; Washington DC, USA) [3–5]. This new technology provided the surgeon with stereoscopic vision to reinstate depth perception, hardware compensation of the fulcrum effect via console design, and tremor control and scaled motion to improve the accuracy of instrument movement well beyond what a normal set of hands was capable of. Yet robot‐assisted surgery came with its own learning curve in addition to the basics, such as console management, robotic instrument knowledge, docking of the machine, and emergency undocking in case of life‐threatening complications. At the same time, complete knowledge of surgical anatomy, procedural skills, and basic surgical principles still had to be properly mastered before dealing with the new technology. Moreover, modern surgery with its complex scenarios has raised the importance of so‐called “nontechnical skills” [6] (situational awareness, communication, teamwork, leadership, stress management) in order to safely perform a surgical procedure, in spite of technological advancements. Whenever we approach mastering something new, scaffolding [7, 8] facilitates acquisition of the required skills. This principle is equally valid whenever we are learning to ride a bicycle or to perform a new surgical intervention. Despite the famous phrase “to err is human,” limiting errors and facilitating the correct technique becomes critical in the surgical field, as patients cannot continue to be the training platform. Simulation has the goal of approaching the same situation multiple times, in order to understand it, apply different strategies to solve it, assess the pros and cons, select a preferred approach, and improve on it in a safe environment. It is widely used in several nonmedical fields to test new products and procedures, improve performance, predict future outcomes, and certify the acquisition of skills. In surgery, shortened hospital stays, shortened residency hours, and the rate at which new procedures and new technologies are introduced translate into a reduced number of operative cases for learners to participate in for any given procedure [9–11]. Moreover, in situ skills assessment is challenging and the results of residency training are barely measured or considered in view of becoming a full surgeon. Although the use of simulation could potentially fill these gaps, its application is not yet as standardized or widespread as one would imagine. Indeed, it is used to varying degrees from country to country and even from institution to institution, leading to significant differences in technical and nontechnical skills between young practitioners in the same field. The American College of Surgeons’ Accredited Educational Institutes (ACS‐AEI) program was started in 2005 with the aim of providing guidelines and standards for simulation centers inside and outside the United States. The results turned out to be outstanding, and there are currently 94 accredited centers worldwide [12]. Despite the significant inroads, many sim‐centers still prefer not to adopt a standardized training model, continuing to create their own model instead. In Europe simulation is not typically considered a critical part of the urology residency curriculum. In the last decade some countries have adopted standardized training protocols to provide learners with a unified model to follow, preparing them for medical practice. This happened in the Netherlands with the 40 hours program and in the United Kingdom with the “Guidelines for training” [13] written under the auspices of the British Association of Urological Surgeons (BAUS). In Europe, simulation centers are still not common and few universities offer residents a chance to use pelvic trainers or simulators in general. Recognition of the need for deliberate, repeated simulation training and the lack of proper tools has led hands‐on training (HoT) sessions to become more and more popular all over Europe. HoTs provide tutoring on dedicated models during congresses and educational events. These sessions use standardized training kits with predefined exercises and learning objectives. The European Urology Residents Education Program (EUREP) [14], flagship training event of the European Association of Urology (EAU), introduced the HoT format into its schedule in 2006 and today attracts hundreds of final‐year residents from all over Europe, exposing learners from many countries to a unified training protocol [15]. The Urological Society of Australia and New Zealand (USANZ) streamlined the training regimen with the nSET Program, which includes training activities through the six residency years up to independent clinical practice, including the achievement of primary operator skills for laparoscopic surgery. Technical skills training is still not common practice in Africa, South America, and Asia, where structured technical skills teaching is limited to few training centers and dedicated courses. Robotic surgery training is not yet formally considered as a part of urological education worldwide and few residents have a chance to perform robot‐assisted surgery as first operators before the end of their learning path. Although ancient texts, such as the Sushruta Samhita from India, laid down in Sanskrit around 500 CE (original date unknown, but thought to go back to 1000 BCE), mention the use of various models to practice surgical skills for speed and accuracy, we will focus on recent history to follow our current model for medical education. Surgical residency as we know it today in the United States began in the early 1900s at Johns Hopkins University in Baltimore, MD, under the guidance of William Halstead. During those formative years, we have evidence that dog labs were used to teach both procedural as well as team‐based skills. We also tend to point at flight simulators to make a case for the use of simulation in medicine. Surprisingly, that path began later, in 1929, with the Link Trainer (Figure 75.1) [16], which was instrumental in training young pilots how to land on aircraft carriers. Figure 75.1 The Link Trainer. These early trainers can be compared to the basic skills trainers we see today, such as FLS, BLUS, and E‐BLUS (see below) [17–19]. The growth of the Link Trainer into today’s full flight simulators and regimented training programs could not have happened without a significant investment by the US Department of Defense (DoD) to develop the science and technology that underlies them. We can recognize multiple phases in the progression of simulation in healthcare. The initial driver was the promise of objective assessment. In theory, by using virtual reality (VR) for training and assessment, we could measure everything that was represented in the mathematical models. Thus we could look at variables such as time and distance, but also collisions, penetrations, and path lengths, and even the use of energy, radiation, and blood loss. The believers were so excited about the possibilities that the mantra was “build it and they will come.” For the first VR simulators to be developed a number of technologies had to evolve. Many of them were supported by the DoD, such as graphics processors, head mounted displays, and haptic interfaces. As these technologies came into existence, by many accounts Dr Richard Satava, a laparoscopic surgeon who at the time was at DARPA, began funding projects in the late 1990s to see if “flight simulators for surgery” were within reach [20]. Some of these early efforts resulted in a sinus surgery platform [21, 22] and a vascular anastomosis trainer, among others [23, 24]. The second wave came only a few years after that, with the tagline “validate it and they will come.” Early validation studies provided much‐needed proof of the scientific and educational merits of surgical simulation. The key study which set the standard that many followed was that by Seymour et al. [25]. By that time a number of companies in the United States and abroad had launched commercial products into the market. That opened up the door for the next phase in development: an integrated curriculum, where simulation was one component of the whole. Validation studies gave the new technology legitimacy, while the integrated curriculum concept paved the way for today’s comprehensive view: backwards design, predefined learning objectives, assessment tools, part task trainers, human patient simulators, and full procedural VR simulators. In the last few years the DoD has refocused on medical simulation with significant investment in infrastructure: funding the development of open‐standards‐based building blocks to support the big picture and exponentially accelerate development. To formalize even more the birth of this new field of medical education and to push its development and widespread, ACS‐AEI accredited in 2014 a research fellowship program in education and simulation technologies, to be delivered by selected centers. When a new surgical intervention has to be learned, there are many topics that need to be addressed. Given a learner’s previous experience, some of those may be skipped, some may just need refreshing, and others may require in‐depth study and practice. Figure 75.2 provides a picture of the many elements that go into learning a surgical procedure. The concept is based on two principles: first and foremost a focus on patient management; second, bringing all contributors to safely performing a new procedure under one umbrella. This allows the learners to assess what they already are comfortable with, review where they have gaps, and finally layer in the new and difficult components specific to the procedure. The complexity of the procedural training template in Figure 75.2 demonstrates how difficult it is to move from basic clinical knowledge in medicine combined with basic technical skills to complete procedures and finally take on the responsibility of independent patient management. The true value of having a standardized template is to allow academic surgeons from all over the world to collaborate and contribute to this vast endeavor in developing training materials for all procedures [9, 26, 27]. Figure 75.2 Procedural training template. Source: CREST, University of Washington, USA. Reproduced with permission of CREST. To acquire the technical skills portion of a new procedure, we can often use some of the existing simulators. One of the first simulators to be validated with sufficient rigor in order to be used for a high‐stakes exam was Fundamentals of Laparoscopic Surgery (FLS). It was first proposed at McGill University by Dr Gerry Fried [17], then developed under the auspices of SAGES, and validated in collaboration with the ACS [28]. FLS tasks were thought to reproduce the basic movements used in laparoscopy and were so well planned to be easily adapted to basic skills teaching in other laparoscopic specialties, such as gynecology and urology. FLS was developed to target basic maneuvers and not full procedures. In the United States FLS has been used as a starting point for the creation of the Basic Laparoscopic Urological Skills (BLUS) curriculum. Despite a study [18] showing validity, the BLUS exam is not mandated and is used only by choice at select residency programs. The EAU started to build upon the FLS experience in 2011, when the European Basic Laparoscopic Urological Skills (E‐BLUS) [19] exam was given for the first time. E‐BLUS is today the only technical skills certification to be mandated by an international urological society. It is made up of four tasks: peg transfer, circle cut, needle guidance, and intracorporeal knot tying (Figure 75.3). Depending on faculty’s preference, basic training protocols often include the use of a camera navigation trainer [29] for a 30° laparoscope, although this exercise is not included in the examination. Initially intended as a stand‐alone certification program, E‐BLUS is rapidly evolving into the first step of a comprehensive pathway, guiding the novice from basic skills to full procedures and mastery. Figure 75.3 The E‐BLUS tasks. Modular hands‐on training, as depicted in Figure 75.4, suggests approaching technical skills training in three distinct steps: basic (simple skills), intermediate (complex tasks), and advanced (full procedures) [30, 31]. This general concept, in this case applied to laparoscopy, prescribes a logical progression for novices, a learning path to prepare them for mentored cases with patients. Figure 75.4 Modular hands‐on training. Source: infographics by D. Veneziano MD. Basic skills target fundamental building blocks: simple maneuvers like camera handling, grasping, traction/countertraction, cutting, and single‐knot tying, which form the basis of any surgical technique. These are common to different laparoscopic specialties and that’s why FLS, originally designed for general surgeons, was so easily adopted by urologists. Given the proven validity of trainers like FLS and E‐BLUS, a novice should never learn basic skills on a patient. Intermediate training is much more specialty‐specific and focuses on the “complex tasks:” the most challenging steps of full procedures. Indeed, mastering basic skills, as well as practicing complex tasks on bench models, prepares the trainee to approach full procedural, or advanced training. At this stage the trainee has to link all the concepts learned along the way in order to perform the whole procedure (making errors, and learning how to recover) in a safe, simulated environment, before working on a real patient. While progressing from basic skills to full procedures, focus shifts to different aspects of surgical education, from technical skills to decision making and patient management, necessitating very different types of simulators. While laparoscopic basic skills are already well documented and standardized, complex tasks for intermediate training are not yet fully defined and incorporated into validated curricula. Moreover, these are much more challenging to simulate. A first comprehensive curricular implementation exists as a joint effort between the ACS and the Association of Program Directors in Surgery (APDS) as the ACS/APDS Surgery Resident Skills Curriculum Phases I, II, and III, going from basic steps and tasks to procedures and team‐based training [32–35]. Laparoscopic complex tasks according to the American Urological Association (AUA) and the EAU are summarized in Table 75.1. In either case, both American and European Urological societies agree that mastering complex tasks is critical before continuing on to perform complete procedures. Table 75.1 Complex tasks according to AUA and EAU.
Training and Credentialing Laparoscopic and Robotic Surgery
Surgical training today: a complex scenario
History of surgical training: a growing field
From FLS to the concept of modular hands‐on training
AUA Laparoscopic, Robotic and New Surgical Technology (LRNST)
ESU/European School of Urology and section of Uro‐Technology (ESUT) training research group
Pyeloplasty
Horizontal anastomosis (as per pyeloplasty)
Y‐V plasty
Vertical anastomosis (as per vesicourethral)
Vesicourethral anastomosis
Hilar dissection
Control of aortic and inferior vena cava injury
Major vessel injury repair
Tumor enucleation and suture of renal parenchyma
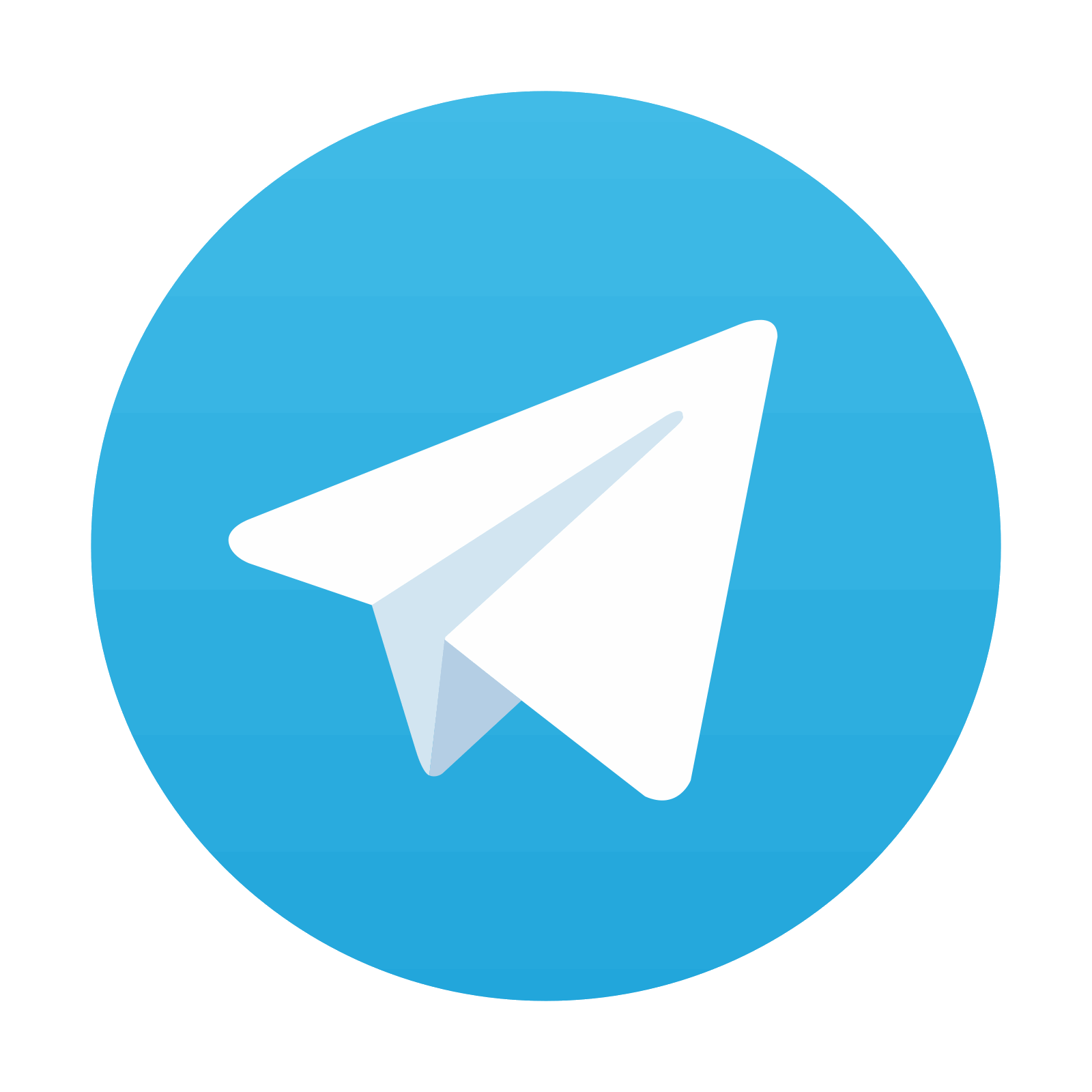
Stay updated, free articles. Join our Telegram channel
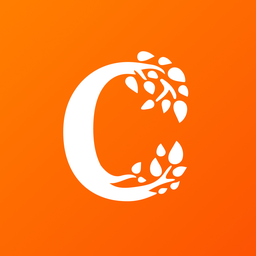
Full access? Get Clinical Tree
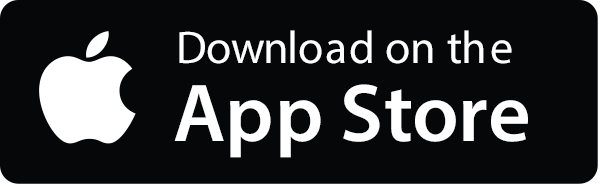
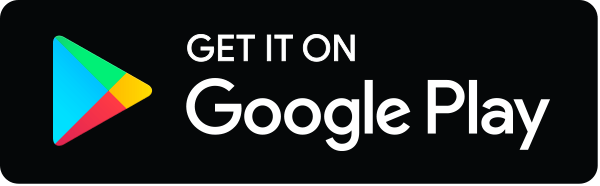