Abstract
Interstitial cells of Cajal (ICC) are part of the control system that orchestrates and regulates a diverse set of motor patterns in all parts of the gut to facilitate absorption, transit, neural innervation, stretch sensation, and bacterial homeostasis. ICC are unique cells that either have intrinsic pacemaker activity, or can perform stimulus-induced pacemaking; they act as networks and this network is connected to inhibitory and excitatory intrinsic and extrinsic nerves that influence the ICC network. Ultimately, it is the smooth muscle cells that generate contractile activity. The smooth muscle cells respond to stimuli to contract; all stimuli affect the electrical activity of the smooth muscle cells that regulates calcium influx, the trigger for contraction. The primary stimulus is depolarization, which is received from ICC in a rhythmic fashion and from other stimuli such as distention or neurotransmitters interacting with the numerous receptors on its surface. Many patterns of contractions are determined by the rhythmic depolarization coming from a single ICC network, or are determined by more complex changes in electrical activity as a consequence of interaction of pacemaker activities coming from different ICC networks. The amplitude of contractions is determined by the absolute membrane potential, how far it reaches above threshold for calcium channel activation.
Keywords
ICC, Interstitial cells of Cajal, Pacemaking, Innervation, Stretch, Distention, Calcium, Peristalsis, Segmentation, Colon, Intestine, Stomach, Esophagus, Motility, Constipation, Inflammation, Chloride channels, Nitrergic, Cholinergic, Vagus
Acknowledgments
My research has been consistently funded by grants from the Canadian Institutes of Health Research and the Natural Sciences and Engineering Research Council of Canada. This review is dedicated to all students, post docs, technicians, research assistants, and research scientists past and present in my laboratory with whom it has been and continues to be such a great pleasure to discover ICC structure and function.
13.1
The Influence of ICC Pacemaker Cells on the Electrical Activity of Smooth Muscle
13.1.1
The Early Discussions on Gut Pacemaker Activity
In the early 20th century, Walter Alvarez realized that electrical activity was underlying the movements of the gut organs and started to record “action currents.” He soon understood that there was a disconnect between the electrical activity and the contractile activity since often the electrical activity would continue even though the contractions waned. He also discovered that the frequency of contractions in the intestine was higher in the proximal compared to the distal end and so the concept of pacemaking in the gut and a key component of the pacemaker network, its frequency gradient, was born. Hence, rhythmic smooth muscle depolarizations (slow waves) were underlying the rhythmic contractions of the intestine. Nevertheless, Alvarez struggled with the physiology of peristalsis and he and Alexander Keith discussed a potential role for specialized pacemaker cells, which Keith had postulated based on anatomical observations and comparisons with the sinus node. In the end, Alvarez rejected Keith’s ideas based on the fact that he could cut the intestine into sections and every section would show peristalsis. Alvarez assumed that according to Keith’s ideas there would be a single-dominant pacemaker (a gut sinus node) that would govern the rest of the intestine, even though Keith suggested that each section of the intestine would have its own pacemaker. This discussion continues today, with theories assuming that gut pacemaking travels through the intestine similar to a cardiac action potential and theories assuming that a network of millions of continuously active pacemaker cells orchestrates pacemaking.
13.1.2
Electrical Activity Generates Gut Smooth Muscle Contraction in a Two-Step Process
Gut smooth muscle contracts on demand; hence, a trigger needs to initiate the contractions. In the heart, this trigger is the autonomous pacemaker action potential from the sinus node; in the gut, the trigger is a two-step process. Gut smooth muscle cells contract when calcium enters the cell cytoplasm, originating from the cell surroundings, potentially fortified by calcium from the sarcoplasmic reticulum. Calcium from the extracellular fluid enters the cell through calcium- and/or voltage-activated calcium channels. For the smooth muscle cells to reach the threshold for opening of the calcium channels, significant depolarization is needed. For gut smooth muscle that is dominated by pacemaker activity (slow waves) such as in the stomach and small intestine: the smooth muscle cells are constantly subjected to rhythmic depolarizations from the interstitial cells of Cajal (ICC) pacemaker cells, but this might not be enough to trigger contraction. Hence, another stimulus such as muscle stretch or neural activity is needed to further depolarize the cell and so bring the slow-wave plateau above the threshold for the generation of smooth muscle action potentials that bring calcium into the cells. The subsequent mechanisms to generate contraction are excellently reviewed by Bitar et al.
13.1.3
The Development of the ICC Pacemaker Hypothesis
In 1889, Cajal published on an interstitial cell type that came to be associated with his name although Cajal thought them to be interstitial neurons. In the following 90 years, the ICC remained a topic of investigation by anatomists, and suggestions on a potential role in gut motility control often surfaced. The first doctoral thesis on ICC was written by Jan Boeke at the University of Utrecht in 1949 who summarized his interpretation as follows: “Interstitial cells of Cajal are the neurohumoral region of the sympathetic nerve endings, the synaptic field, where humoral energy is produced necessary for the transmission of the nervous stimulus.” Nelemans and Nauta concluded in 1949 that “Since most organs containing interstitial cells show rhythmicity…. it seems to us most probable that we have to find the origin of this rhythmicity in the interstitial network” and their studies indicated communication between ICC and the extrinsic autonomic nervous system “The sympathetic and parasympathetic fibers likely make synaptic contact with the interstitial cells.”
In the late 1970s, physiologists started to deduce from their experiments that the slow-wave activity may not be a general property of smooth muscle cells. Edwin Daniel started to talk about hybrid cells that may interact with smooth muscle cells and nerves. Tadao Tomita surmized that the slow waves might originate from cells in between the circular and longitudinal muscle layer. Nelson Durdle scraped the submucosa off the circular muscle of the dog colon and observed the slow waves to disappear, suggesting that circular smooth muscle cells may not have intrinsic slow-wave activity.
An important moment in the history of ICC physiology was the 9th International Symposium on Gastrointestinal Motility held in 1983 in Aix-en-Provence where Lars Thuneberg showed data on methylene blue mediated destruction of ICC-MP (see Section 13.2.1 ) in the intestine and the loss of slow waves as a consequence, providing the first indirect physiological evidence of a pacemaker function of ICC. Thuneberg also showed videos of contracting ICC in culture. For many physiologists present, it was the first time that ICC came on their radar screen, and for some it was the start of incorporating ICC into their physiological research from then on. Thuneberg published his ideas about ICC as pacemaker cells, focusing on the mouse small intestine, in his seminal thesis “Interstitial cells of Cajal: intestinal pacemaker cells?” A similar hypothesis based on electron microscopic observations on the human esophagus was put forward by Faussone Pellegrini who published her original ideas in Italian in 1976, later translated into English.
Dissection experiments provided evidence for a role of ICC in pacemaking in the colon. The original observations from Durdle et al. that scraping the submucosa from the circular muscle layer caused loss of slow waves in the dog colon were confirmed by Smith et al. in 1987. The junction of smooth muscle and submucosa was shown to contain ICC. Conklin and coworkers showed that, also in the cat colon, slow waves depended on an intact interface with the submucosa. Many studies followed to characterize the ICC-SMP as the pacemaker cells of the canine colon. From the ICC-SMP (see Section 13.2.4 ), slow waves propagate actively into the circular muscle layer where they rhythmically depolarize the network of muscle cells. The slow-wave plateau remains at a relatively constant level of depolarization; from the submucosa to the myenteric plexus, the slow-wave amplitude decreases, this is not due to electrotonic decay but to a gradient in membrane potential. When ICC-SMP are removed from the circular muscle layer, the smooth muscle cells at the ICC-SMP border depolarize by about 18 mV. Hence, ICC-SMP not only provide rhythmic depolarization but they also hyperpolarize adjacent smooth muscle cells.
13.1.4
The Definitive Experiments on ICC Pacemaking in the Mouse Small Intestine
Thuneberg’s hypotheses were based on the ICC-MP in the mouse small intestine, and physiologists turned again to this model to provide definitive proof of ICC as pacemaker cells. Maeda et al. described how Kit antibodies eliminated Kit-positive cells from the intestine and affected the rhythmicity of the intestinal contractions. This was followed by studies on mutant mice that have a malfunctioning kit receptor, which causes inability to develop ICC-MP after birth ; it was shown that these mice lost the ability to generate slow-wave activity and slow-wave-driven peristalsis in the proximal small intestine. The small intestine still functions, the musculature generates normal action potentials because the loss of ICC results in marked depolarization of smooth muscle cells allowing action potential generation at the resting membrane potential instead of at the plateau of slow waves. In 1998, the definitive piece of evidence was reported : ICC isolated from the myenteric plexus area of the mouse small intestine, held in short term culture, produced spontaneous rhythmic inward currents with a reversal potential of + 10 mV or + 17 mV whereas smooth muscle cells did not. It was thought that the channel involved was a nonselective cation channel.
13.1.5
Role of ICC in Setting Smooth Muscle Cell Membrane Potential
The circular muscle layer of the stomach and small intestine in dogs, cats, mice, and humans have a resting membrane potential gradient with the myenteric plexus region being up to 23 mV hyperpolarized compared to the submucosal border. The advantage may be that the musculature can generate weak and strong contractions dependent on the region depolarized enough to generate action potentials. An important contributor to the gradient is carbon monoxide, which is generated by ICC-MP. Carbon monoxide is generated by heme oxygenase 2 (HO2) and in HO2-KO mice and ICC-MP depleted tissue, the gradient is abolished.
In the dog and mouse colon, a membrane potential gradient exists but the most hyperpolarized region is adjacent to the submucosa. In the mouse colon, the gradient was shown to depend on carbon monoxide generated by HO2, but the enzyme was found in submucosal neurons and not in ICC-SMP. Hydrogen sulfide also plays a role in chronic hyperpolarization of smooth muscle by blocking nitric oxide synthesis and as such contributes to the maintenance of the membrane potential gradient. The hydrogen sulfide generating enzymes are found in enteric neurons.
Acknowledgments
My research has been consistently funded by grants from the Canadian Institutes of Health Research and the Natural Sciences and Engineering Research Council of Canada. This review is dedicated to all students, post docs, technicians, research assistants, and research scientists past and present in my laboratory with whom it has been and continues to be such a great pleasure to discover ICC structure and function.
13.1
The Influence of ICC Pacemaker Cells on the Electrical Activity of Smooth Muscle
13.1.1
The Early Discussions on Gut Pacemaker Activity
In the early 20th century, Walter Alvarez realized that electrical activity was underlying the movements of the gut organs and started to record “action currents.” He soon understood that there was a disconnect between the electrical activity and the contractile activity since often the electrical activity would continue even though the contractions waned. He also discovered that the frequency of contractions in the intestine was higher in the proximal compared to the distal end and so the concept of pacemaking in the gut and a key component of the pacemaker network, its frequency gradient, was born. Hence, rhythmic smooth muscle depolarizations (slow waves) were underlying the rhythmic contractions of the intestine. Nevertheless, Alvarez struggled with the physiology of peristalsis and he and Alexander Keith discussed a potential role for specialized pacemaker cells, which Keith had postulated based on anatomical observations and comparisons with the sinus node. In the end, Alvarez rejected Keith’s ideas based on the fact that he could cut the intestine into sections and every section would show peristalsis. Alvarez assumed that according to Keith’s ideas there would be a single-dominant pacemaker (a gut sinus node) that would govern the rest of the intestine, even though Keith suggested that each section of the intestine would have its own pacemaker. This discussion continues today, with theories assuming that gut pacemaking travels through the intestine similar to a cardiac action potential and theories assuming that a network of millions of continuously active pacemaker cells orchestrates pacemaking.
13.1.2
Electrical Activity Generates Gut Smooth Muscle Contraction in a Two-Step Process
Gut smooth muscle contracts on demand; hence, a trigger needs to initiate the contractions. In the heart, this trigger is the autonomous pacemaker action potential from the sinus node; in the gut, the trigger is a two-step process. Gut smooth muscle cells contract when calcium enters the cell cytoplasm, originating from the cell surroundings, potentially fortified by calcium from the sarcoplasmic reticulum. Calcium from the extracellular fluid enters the cell through calcium- and/or voltage-activated calcium channels. For the smooth muscle cells to reach the threshold for opening of the calcium channels, significant depolarization is needed. For gut smooth muscle that is dominated by pacemaker activity (slow waves) such as in the stomach and small intestine: the smooth muscle cells are constantly subjected to rhythmic depolarizations from the interstitial cells of Cajal (ICC) pacemaker cells, but this might not be enough to trigger contraction. Hence, another stimulus such as muscle stretch or neural activity is needed to further depolarize the cell and so bring the slow-wave plateau above the threshold for the generation of smooth muscle action potentials that bring calcium into the cells. The subsequent mechanisms to generate contraction are excellently reviewed by Bitar et al.
13.1.3
The Development of the ICC Pacemaker Hypothesis
In 1889, Cajal published on an interstitial cell type that came to be associated with his name although Cajal thought them to be interstitial neurons. In the following 90 years, the ICC remained a topic of investigation by anatomists, and suggestions on a potential role in gut motility control often surfaced. The first doctoral thesis on ICC was written by Jan Boeke at the University of Utrecht in 1949 who summarized his interpretation as follows: “Interstitial cells of Cajal are the neurohumoral region of the sympathetic nerve endings, the synaptic field, where humoral energy is produced necessary for the transmission of the nervous stimulus.” Nelemans and Nauta concluded in 1949 that “Since most organs containing interstitial cells show rhythmicity…. it seems to us most probable that we have to find the origin of this rhythmicity in the interstitial network” and their studies indicated communication between ICC and the extrinsic autonomic nervous system “The sympathetic and parasympathetic fibers likely make synaptic contact with the interstitial cells.”
In the late 1970s, physiologists started to deduce from their experiments that the slow-wave activity may not be a general property of smooth muscle cells. Edwin Daniel started to talk about hybrid cells that may interact with smooth muscle cells and nerves. Tadao Tomita surmized that the slow waves might originate from cells in between the circular and longitudinal muscle layer. Nelson Durdle scraped the submucosa off the circular muscle of the dog colon and observed the slow waves to disappear, suggesting that circular smooth muscle cells may not have intrinsic slow-wave activity.
An important moment in the history of ICC physiology was the 9th International Symposium on Gastrointestinal Motility held in 1983 in Aix-en-Provence where Lars Thuneberg showed data on methylene blue mediated destruction of ICC-MP (see Section 13.2.1 ) in the intestine and the loss of slow waves as a consequence, providing the first indirect physiological evidence of a pacemaker function of ICC. Thuneberg also showed videos of contracting ICC in culture. For many physiologists present, it was the first time that ICC came on their radar screen, and for some it was the start of incorporating ICC into their physiological research from then on. Thuneberg published his ideas about ICC as pacemaker cells, focusing on the mouse small intestine, in his seminal thesis “Interstitial cells of Cajal: intestinal pacemaker cells?” A similar hypothesis based on electron microscopic observations on the human esophagus was put forward by Faussone Pellegrini who published her original ideas in Italian in 1976, later translated into English.
Dissection experiments provided evidence for a role of ICC in pacemaking in the colon. The original observations from Durdle et al. that scraping the submucosa from the circular muscle layer caused loss of slow waves in the dog colon were confirmed by Smith et al. in 1987. The junction of smooth muscle and submucosa was shown to contain ICC. Conklin and coworkers showed that, also in the cat colon, slow waves depended on an intact interface with the submucosa. Many studies followed to characterize the ICC-SMP as the pacemaker cells of the canine colon. From the ICC-SMP (see Section 13.2.4 ), slow waves propagate actively into the circular muscle layer where they rhythmically depolarize the network of muscle cells. The slow-wave plateau remains at a relatively constant level of depolarization; from the submucosa to the myenteric plexus, the slow-wave amplitude decreases, this is not due to electrotonic decay but to a gradient in membrane potential. When ICC-SMP are removed from the circular muscle layer, the smooth muscle cells at the ICC-SMP border depolarize by about 18 mV. Hence, ICC-SMP not only provide rhythmic depolarization but they also hyperpolarize adjacent smooth muscle cells.
13.1.4
The Definitive Experiments on ICC Pacemaking in the Mouse Small Intestine
Thuneberg’s hypotheses were based on the ICC-MP in the mouse small intestine, and physiologists turned again to this model to provide definitive proof of ICC as pacemaker cells. Maeda et al. described how Kit antibodies eliminated Kit-positive cells from the intestine and affected the rhythmicity of the intestinal contractions. This was followed by studies on mutant mice that have a malfunctioning kit receptor, which causes inability to develop ICC-MP after birth ; it was shown that these mice lost the ability to generate slow-wave activity and slow-wave-driven peristalsis in the proximal small intestine. The small intestine still functions, the musculature generates normal action potentials because the loss of ICC results in marked depolarization of smooth muscle cells allowing action potential generation at the resting membrane potential instead of at the plateau of slow waves. In 1998, the definitive piece of evidence was reported : ICC isolated from the myenteric plexus area of the mouse small intestine, held in short term culture, produced spontaneous rhythmic inward currents with a reversal potential of + 10 mV or + 17 mV whereas smooth muscle cells did not. It was thought that the channel involved was a nonselective cation channel.
13.1.5
Role of ICC in Setting Smooth Muscle Cell Membrane Potential
The circular muscle layer of the stomach and small intestine in dogs, cats, mice, and humans have a resting membrane potential gradient with the myenteric plexus region being up to 23 mV hyperpolarized compared to the submucosal border. The advantage may be that the musculature can generate weak and strong contractions dependent on the region depolarized enough to generate action potentials. An important contributor to the gradient is carbon monoxide, which is generated by ICC-MP. Carbon monoxide is generated by heme oxygenase 2 (HO2) and in HO2-KO mice and ICC-MP depleted tissue, the gradient is abolished.
In the dog and mouse colon, a membrane potential gradient exists but the most hyperpolarized region is adjacent to the submucosa. In the mouse colon, the gradient was shown to depend on carbon monoxide generated by HO2, but the enzyme was found in submucosal neurons and not in ICC-SMP. Hydrogen sulfide also plays a role in chronic hyperpolarization of smooth muscle by blocking nitric oxide synthesis and as such contributes to the maintenance of the membrane potential gradient. The hydrogen sulfide generating enzymes are found in enteric neurons.
13.2
The Different Types of ICC
Structure and function are inextricably intertwined and to get insight into the essential components of ICC structure it is an absolute delight to read the ICC Atlas written and illustrated by Terumasa Komuro covering all ICC subtypes, focused on the guinea pig. In general, ICC form two-dimensional networks of cells throughout the gut, localized in association with the myenteric plexus, the nerve plexus located at the inner border of the circular muscle layer, and at the serosal surface. Three-dimensional networks are present within the muscle layers. They are hence ideally suited to transmit pacemaking activity throughout the musculature and be an active conduit between nerves and smooth muscle.
13.2.1
ICC-MP (ICC Associated With the Myenteric Plexus)
ICC-MP provide primary pacemaking to the stomach and small intestine and secondary pacemaker activity to the colon ( Fig. 13.1 ). They are found around the myenteric ganglia and in between the ganglia, they form a continuous network of pacemaker cells around the circumference and along the length of the organ. ICC, in general, have features of smooth muscle cells and fibroblasts and their electron microscopic identification and differentiation needs expert evaluation; their identity is in part dependent on their connection to associated cells. Principle pacemaker ICC appear to have a stellate-shaped cell body with a large nucleus and relatively sparse cytoplasm and processes extending in all direction, connected to other ICC-MP by gap junctions. Pacemaker ICC are rich in mitochondria, likely related to the need for ongoing pacemaker activity and rich in caveolae related to calcium handling. ICC have intermediate filaments but no thick filaments and are not contractile. Thuneberg showed dramatic contractile activity in isolated ICC after a few days in culture, but recent studies using calcium imaging do not provide evidence for contractile activity in situ. An example of ICC density: there are about 750 cells per mm 2 in ICC-MP in the mouse small intestine or a total of 2.5 million. ICC-MP are Kit positive, the Kit protein is essential for ICC development, and recovery after injury, and has as natural ligand, steel factor. Hence, mutations in both the Kit protein and steel factor (the Sld/Sld mice) lead to absence of ICC-MP and exposure to Kit antibodies will destroy the ICC. Enteric nerves regulate ICC-MP activity.

13.2.2
ICC-DMP (ICC Associated With the Deep Muscular Plexus of the Small Intestine)
ICC-DMP are positioned at the outer edge of the circular muscle but separated from the submucosa by a thin layer of circular muscle cells, hence the identification of an inner and outer circular muscle layer with the ICC-DMP in between. The ICC-DMP are associated with a plexus of nerve varicosities along the axons, but no neuronal cell bodies, hence no ganglia. ICC-DMP are usually bi-polar in shape and run along the neighboring circular muscle cells. They are exceptionally well connected to each other and to the outer layer of smooth muscle cells via gap junctions. ICC-DMP have close contacts with nerve varicosities, containing accumulations of synaptic vesicles, via synapse-like junctions. Hence ICC-DMP are intercalated between nerves and smooth muscle cells and can act as an accessory route for neuromuscular transmission, as originally suggested by Cajal. ICC-DMP are Kit positive but they are completely normal in W/Wv or Sld/Sld-mutant mice, hence in contrast to ICC-MP, ICC-DMP maturation is not dependent on the Kit protein. Immunohistochemistry using Kit or Ano1 antibodies shows ICC-DMP processes to be aligned with the long axis of circular muscle cells. In addition to neurotransmission, ICC-DMP are thought to play a role in secondary pacemaking, that is, stimulus-induced pacemaking, where a neural stimulus or distention may evoke rhythmic depolarizations that will interact with the primary pacemaker ICC-MP to generate specific motor patterns such as segmentation. The intimate association of the ICC-DMP with enteric nerves facilitates neurotransmission and neural stimulus-induced pacemaking.
13.2.3
ICC-IM (Intramuscular ICC)
Intramuscular ICC are found throughout the gastrointestinal tract and form a three-dimensional network within the longitudinal and circular muscle layers. Only in the small intestine of small animals are they scarce or absent. They are sometimes subdivided into ICC-CM and ICC-LM. ICC-IM are connected to ICC-MP and as such facilitate the passage of pacemaker activity to the circular muscle layer ( Fig. 13.3 ). ICC-IM are usually bipolar with their long axes along the long axes of smooth muscle cells. They connect with neighboring smooth muscle cells via many large gap junctions and often show close contacts with nerve terminals via synapse-like junctions.
ICC-IM in the esophagus and stomach are connected to vagal afferents; intramuscular arrays (IMAs), vagal stretch receptors, are all intimately connected to ICC-IM. Mutant mice with diminished ICC-IM also show a marked reduction in IMAs suggesting mutual dependence. Destruction of the nodose gangion results in loss of IMAs in the esophagus as well as loss of ICC-IM. Vagal stimulation of ICC-IM in the stomach may induced pacemaker activity in these cells which then becomes dominant over the primary pacemaker activity of the ICC-MP. ICC-IM are therefore monitoring smooth muscle activity and modifying it through their interactions with enteric and extrinsic nerves.
In larger animal and humans, the circular muscle is divided into lamellae with septa in between that extend from the submucosa to the myenteric plexus area. ICC line the septal borders and therefore run in a different direction compared to regular ICC-IM. The septal ICC can be regarded as part of the ICC-IM network although they are sometimes categorized as a separate subtype.
13.2.4
ICC-SMP (ICC Associated With the Submuscular Plexus of the Colon and Antrum)
Colonic ICC-SMP are observed at the interface between the submucosa and the circular muscle layer. They form a network connected by gap junctions and this network is loosely attached to the smooth muscle cells, that is, some sections are gap junction-coupled to smooth muscle cells and other sections are found disconnected and wander into the submucosa. The ICC-SMP not only connect to each other but also connect to ICC-IM in the septa, so that a continuing network of pacemaker cells is present throughout the colon ( Fig. 13.1 ).
A specialized pacemaker function has been proposed for ICC-SMP in the colon, they are primarily responsible for generating the slow waves, and ICC-MP acting as secondary pacemaker cells providing stimulus-dependent pacemaking. ICC-SMP are connected to enteric nerves and have neurotransmitter receptors such as NK1. Consistent with ICC-MP being stimulus-dependent pacemakers in the colon, ICC-SMP-associated contraction patterns are much less influenced by neural blockade compared to activity orchestrated by ICC-MP, and electrical nerve stimulation in vitro also affects ICC-MP-related activity much more compared to the ICC-SMP-related activity. Inhibitory innervation has little effect on ICC-SMP generated slow waves.
In the rat antrum, ICC-SMP were of very similar structure as the ICC-MP. They may functionally be connected to ICC-IM. ICC-SMP have been described in the human antrum, they have a close association with nerve networks but a specific function has not been elucidated yet.
The nonganglionated submuscular plexus is distinct from the ganglionated submucosal plexus; ICC are also present in association with the submucosal plexus and may be involved in orchestration of contractions by the muscularis mucosa.
13.2.5
ICC-SS (ICC of the Subserosa of the Colon)
ICC-SS were described in 1982 by Thuneberg in the mouse. Komuro studied their structure extensively in the guinea pig colon and found them to be distributed within a thin layer of connective tissue lining the mesothelium, appearing to constitute part of the serosa, but commonly referred to as being part of the subserosa. Several branches extend from ICC-SS cell bodies, diverging into secondary and tertiary processes to form an extensive two-dimensional network. In the guinea pig colon, they are connected by gap junctions to each other and by peg and socket junctions and close appositions to smooth muscle cells. Komuro observed that the ICC-SS connect to ICC-IM in the longitudinal muscle and suggested them to be stretch receptors that might trigger contractions of the longitudinal muscle. Connections with ICC-IM in the longitudinal muscle were also seen using 3D imaging. In the guinea pig colon, few nerve fibers were present in this connective tissue layer and a relationship with ICC was difficult to ascertain. ICC-SS may also help in stretch-induced nutrient absorption in the microcirculation and fluid drainage into lymph vessels similar to the fibroblast network underlying epithelial cells in the mucosa. A study by Vanderwinden on the mouse colon showed that ICC-SS were rare at birth but fully developed into a two-dimensional network 7 days after birth, well connected by gap junctions, aligned with smooth muscle cells and in contact with them through peg and socket junctions, and in close apposition to nerve fibers.
Administration of the Kit antibody imatinib for 4 days beginning 8 days after birth markedly reduced ICC-SS together with ICC-MP and ICC-IM ; ICC recovered within 4 days after withdrawal of the drug. Imatinib did not have this effect 24 days after birth suggesting a specific role of Kit in neonatal development.
Rumessen studied ICC-SS by electron microscopy in the human colon and found them to have myoid features, also rich in caveolae and secretory organelles. They did not find gap junctions and although small nerve bundles appeared abundant in the subserosal layer, close contacts with ICC appeared inconspicuous. ICC-SS appeared connected by peg and socket contacts; connections with smooth muscle cells were close appositions as well as peg and socket structures.
13.3
The Structural Basis of Intercellular Coupling of ICC Within Their Networks and Communication With the Smooth Muscle Layers
ICC function as networks and the nature and degree of intercellular coupling is one of its most essential features, yet it is poorly investigated. One difficulty is that selective and specific blockers of gap junctions are lacking. It is clear, however, that changes in ICC coupling can dramatically influence motor patterns. In addition to gap junctions, other modes of communication are present, which are largely unexplored such as peg and socket junctions and close apposition membranes facilitating field coupling.
13.3.1
Electron Microscopy
Gap junctions are universal proteins that connect cells to allow transfer of ions such as K + , Na + , Ca 2 + , and Cl − but also signaling molecules such as cyclic AMP, cyclic GMP, inositol 1,4,5-triphosphate, and ATP, hence allowing for electrical and metabolic communication. Electron microcopy is the perfect tool for establishing intercellular communication through gap junction plaques, which means that a series of connexons have to be present to reveal a 7 line structure; in other words, electron microscopy cannot see individual pairs of connexons. Hence, electron microscopy does not give evidence on the nature or extent of coupling when these gap junction plaques are not present; cells may be richly coupled by connexons assembled in small clusters and electron microscopy will not pick it up. In addition, electron microscopy only gives the presence of gap junction plaques, not their conductance. In the mouse small intestine, the ICC-DMP network has dense presence of gap junctions but the ICC-MP network has a much sparser gap junction distribution, yet the functional significance of this dramatic difference in gap junction coupling is still obscure. Also of interest is that the circular muscle layer has a very high density of gap junction plaques and immunohistochemistry for connexin 43, but the longitudinal muscle layer appears to be devoid of gap junction plaques and immuno-positivity, despite excellent electrical communication within the longitudinal muscle layer. The relationship between gap junctions and electrical communication was attempted to be elucidated by studies from Daniel and his students into the effect of the dramatic increase in gap junctions in the myometrium, just before birth. No physiologically significant increase in electrical coupling could be found. The interpretation is that the electrical coupling is already optimal before the dramatic increase and it suggests that the increase is not to enhance electrical coupling but to enhance metabolic coupling, likely via oxytocin-induced signaling.
13.3.2
Immunohistochemistry
Immunohistochemistry using Kit and Ano1 antibodies has given us tremendous insight into the network structure of the ICC, in particular, when shown in three dimensions as in Komuro’s atlas. It has also given us insight into receptors on ICC and the connexin nature of gap junctions. Connexin 43 (Cx43) is the most widely distributed connexin in the canine gut, both seen in ICC and smooth muscle cells. There was good agreement with presence of gap junction plaques observed using electron microscopy and density of Cx43. Cx45 and Cx40 were found in ICC but far less than Cx43. A similar Cx distribution was found in the rat and guinea pig, except that ICC-DMP were thought to connect through Cx45. In the human gut, the strongest Cx43 staining is in the circular muscle of the small intestine. Immunohistochemistry will not detect single connexins hence density of Cx distribution cannot be equated with functional coupling. In fact, electronmicroscopy appears to be more sensitive in detecting gap junctions than immunohistochemistry. Furthermore, many different connexin proteins have not yet been explored for a role in ICC coupling.
13.3.3
Dye Coupling, Passage of Small Molecules Through Gap Junctions
Dye coupling is a very useful technique, extensively explored by Menachem Hanani, and has revealed strong communication where electron microscopy saw no gap junction plaques. Gap junctions could not be demonstrated in longitudinal muscle cells of the guinea pig small intestine by electron microscopy, but these cells were equally well dye coupled compared to the gap junction-rich circular muscle cells. Dye coupling also demonstrated coupling between smooth muscle cells and ICC in the dog colon where no ultrastructurally defined gap junctions were observed and showed that communication across circular muscle septa occurred through ICC. Dye coupling is also a good technique to reveal metabolic regulation of gap junction communications such as effects of intracellular calcium or pH.
13.3.4
Electrotonic Coupling
Some features of intercellular coupling can be assessed by the characteristics of the propagation of an electrical pulse. In the dog colon, a hyperpolarizing pulse from the circular muscle penetrated into the longitudinal muscle, hyperpolarized it and inhibited action potentials; but dye coupling in this tissue showed that there was no direct communication between the two muscle layers, the communication occurred through ICC-MP.
13.3.5
ICC in the Small Intestine
ICC-MP in the small intestine of laboratory animals are coupled by gap junctions. In the human small intestine, the ICC-MP were described as a network of overlapping cell processes connected by adherence type junctions. However, dye coupling showed that these ICC were coupled to up to 35 other ICC by dye transfer, which was inhibited by octanol, suggesting passage through pairs of connexons. Gap junction inhibitors have been shown to disturb calcium wave propagation between ICC in the mouse ileum. Dye coupling among ICC-MP in the ileum of the guinea pig and human was found to be highly sensitive to small changes in the extracellular pH; mildly acidic pH reduces coupling and mildly basic pH increases it.
Thuneberg never observed gap junction plaques between ICC-MP of the mouse small intestine and smooth muscle cells of either muscle layer, and also dye injection studies failed to show dye passage from ICC-MP into muscle in the small intestine of mice, guinea pigs and humans. Yet, physiological evidence shows that ICC-MP and smooth muscle cells are electrically connected. In the canine colon, the circular and longitudinal muscle layers show synchronized electrical activity, consistent with electrical coupling between these layers, and dye coupling study showed that this coupling is not direct, but mediated by ICC-MP. The results suggest that ICC-MP are weakly connected by connexon-like structures to each of the muscle layers, providing the coupling between the two muscle layers. It was long considered unlikely that the relatively few ICC could electrically dominate a vast area of smooth muscle cells, and the lack of coupling structures was seen as necessary for this isolation. Hence, lack of gap junctions between ICC-MP and smooth muscle cells appears logical; however, in other parts of the gut, for example, in the antrum, there is direct evidence for communication via gap junctions between ICC-MP and muscle cells. Hence, we are still missing fundamental knowledge about structure-function relationships of intercellular communication. We do not know how gap junction conductance or gap junction permeability is physiologically regulated in relation to various functions of the ICC network. We also need more information about the relative role gap junctions play in electrical versus chemical transmission.
ICC-DMP are abundantly coupled by gap junction plaques, and dye coupling is easily demonstrated. ICC-DMP are connected by gap junctions to the outer circular muscle but not the inner circular muscle. Dye coupling studies showed similar features in the guinea pig ileum, sensitive to pH. In the human small intestine, ICC-DMP were found to extend into the outer circular muscle layer and closely associated with nerve terminals. In the rat small intestine, Cx43 was observed in ICC-DMP and smooth muscle cells but Cx45 was observed only in between ICC-DMP adding another layer of intrigue on the functions and regulation of intercellular communication.
13.3.6
ICC in the Stomach
ICC-MP are electrically coupled to both muscle layers in the guinea pig antrum, abundant connexin 43 was found within the ICC-MP network and within the circular muscle layer, but not close to ICC-MP. Dye coupling indicated a dense network of ICC-MP. Interestingly, heptanol did not block dye transfer between ICC-MP similar to observations in the intestine, questioning the ability of heptanol to influence gap junction conduction under these conditions. In the rat stomach, gap junction density was high within ICC-MP.
ICC-IM are bipolar and found throughout the stomach in animal models and humans but ICC-MP are found only in the corpus and antrum. ICC-IM are gap junction coupled to smooth muscle cells and there is extensive electrical communication between ICC-IM and ICC-MP. ICC-IM extend into the septa, likely facilitating communication throughout the circular muscle layer.
13.3.7
ICC in the Colon
ICC-SMP are the dominant pacemaker cells of colonic smooth muscle. In the canine colon, they form a network and neurobiotin spread shows extensive communication with circular smooth muscle cells. In the mouse, no gap junction plaques were seen, but peg and socket junctions and close apposition connections were abundant.
ICC-MP in the human colon are connected by peg and socket junction, whereas gap junction plaques are rarely seen. In general, at the ultrastructural level, gap junction plaque density is usually much lower in humans compared to animal models suggesting that in humans, small aggregates or single connexons are determining communication in addition to close appositions. In the mouse, a dense network of ICC-MP is connected to ICC-IM close to the myenteric plexus, but no ICC-IM are present in the more inner layers.
A rich network of ICC-SS is present in the human and guinea pig colon that communicates with the longitudinal muscle layer, but its function has not been elucidated yet.
13.4
The Electrophysiological Basis of ICC Pacemaking
All ICC assessed thus far can produce rhythmic transient depolarizations but not all ICC generate spontaneous slow-wave activity. This reflects the notion that some types of ICC may generate stimulus-dependent pacemaking. Hence, only when needed do these ICC generate rhythmic depolarizations, which then may interact with omnipresent pacemaking activity so that compound electrical activity develops for specific on-demand motor patterns.
13.4.1
The Making of the Slow Wave
Jonathan Lee and Lars Thomsen recorded rhythmic inward currents in my laboratory from chemically isolated ICC, the final proof that ICC were pacemaker cells, as also shown by Koh et al. These rhythmic currents were first thought to be generated by nonspecific cation channels but are now believed to be primarily generated by chloride channels, probably by more than one type of chloride channel. These channels are activated by calcium that is rhythmically released from the sarcoplasmic reticulum, hence the rhythmic release and reuptake of calcium is the pacemaker clock. Classical pacemaking activity in the mouse small intestine is not blocked by calcium channel blockers but it is inhibited by blocking calcium influx into the sarcoplasmic reticulum or blocking the IP 3 receptor in the calcium store. This has also been shown to occur in the stomach. In the mouse intestine, calcium imaging has convincingly shown that ICC within a network all oscillate and in the field of view of a microscope they appear to oscillate in unison and their rhythmicity coincides with the rhythmicity of depolarization. It is likely that several channels are activated by calcium to generate pacemaker depolarization; three have been the focus of much research, a high conductance channel that carries chloride and sodium, the Ano1 chloride channel and a sodium channel.
In the mouse small intestine ICC, maxi channels are proposed to be an important component of pacemaking; the channel has single channel openings of 30 or 60 pS and carries chloride and cations, in particular, sodium. In many studies, they were identified as a high-conductance chloride channel that was spontaneously and rhythmically active at the same frequency as the rhythmic slow waves defining ICC pacemaker activity. Main conductance levels occurred between 122 and 144 pS and between 185 and 216 pS. Periodicity in the channel opening coincided with periodicity in membrane potential change, hence, at the single channel level, chloride channels were seen to be associated with the generation of rhythmic changes in membrane potential leading to the conclusion that ICC harbor high-conductance chloride channels that participate in the generation of pacemaker activity ( Fig. 13.2 ). The maxi chloride channel was seen to be activated by intracellular calcium. In the whole-cell configuration, the maxi-chloride channel activity was enhanced by volume increase and was constitutively active and contributed to the resting membrane potential. The studies mentioned thus far were carried out using chemically isolated ICC, that is, small pieces of tissue were gently exposed to digestive enzymes to allow several ICC to be identified at the edge of the small explants such that they could be patch clamped ( Fig. 13.2 ). The uncertainty that the maxi-chloride channel was expressed or changed by culture conditions was taken away when successful patch clamping was carried out in situ . A dominant channel thus identified was a chloride channel that showed an average single channel conductance of 61 pS, but with dominant openings of 126 pS. These in situ experiments provide strong evidence for the chloride channel being one of the pacemaker channels generating the rhythmic inward currents. First, in situ, the presence of the channel is prominent. Second, channel activity appeared in a burst-type fashion in the cell-attached configuration with a high open probability at the resting membrane potential. Clearly, intracellular activity regulates the channel activity where periods of quiescence alternate with high activity of the channel, all at a constant membrane potential. The in situ technique was further refined to be able to distinguish ICC-MP using differential interference contrast optics. It became clear that the maxi-chloride channel is also very permeable to Na and that the single channel conductance may be 30 pS. In fact, when the ionic composition favored cation channel passage, a 29.8 pS channel was identified that showed bursting activity in response to exposure to substance P, both using isolated cells and in situ. Substance P promotes IP3-sensitive calcium release from the sarcoplasmic reticulum in ICC and promotes inward currents in the whole-cell configuration via NK1 receptors. Both excitatory motor neurones and primary afferent sensory neurones in the plexuses of the gut are known to release substance P at the level of the myenteric plexus, and ICC closely appose intrinsic afferent neurons. Indeed, neurobiotin injection in AH neurons together with ICC-MP immunohistochemistry proved the presence of multiple contacts between AH neuron varicosities and the cell bodies and processes of ICC-MP. Generating action potential activity in AH neurons led to increase in the frequency and amplitude of calcium transients underlying pacemaker activity in ICC-MP. Chloride channels in ICC are also activated by M3 muscarinic receptors increasing the amplitude and frequency of spontaneous transient inward currents.


A different calcium-sensitive chloride channel was shown to be critical for ICC pacemaking, the Ano1 channel. The ano1 protein is found in all ICC and is used to identify them using immunohistochemistry. Recordings from freshly isolated ICC demonstrated a chloride channel that was activated by depolarization and sensitive to intracellular Ca 2 + , extracellular Ca 2 + , and blockers of T-type Ca 2 + channels. The currents reversed at the Cl − equilibrium potential and the single channels had a conductance of 7.8 pS, which was consistent with the conductance produced by expression of Ano1 in HEK293 cells. Genetic deletion of Ano1 in mice results in loss of slow waves in smooth muscle of the small intestine but not in loss of ICC-MP. Spontaneous Ca 2 + transients are present in ICC-MP in both Ano1 wild type and knockout mice. However, Ca 2 + transients within the ICC-MP network in Ano1 knockout mice are uncoordinated, while they are rhythmic and coordinated in Ano1 wild-type mice. Conditional knockouts indicated that high loss of Ano1 maintains Ca transient synchronicity and slow-wave activity indicating a large functional reserve.
A role for a sodium channel, the SCN5A encoded Nav1.5 channel, in ICC pacemaking has been proposed for ICC isolated from human intestinal musculature where the sodium channel blocker QX-314 slowed the rate of rise and reduced the frequency of slow-wave activity. Interestingly, 50% of patients with a SCN5A mutation, leading to a cardiac long QT syndrome, reported abdominal pain compared to only 13% of controls. The sodium channel is stretch sensitive hence may be activated by the constant mechanical forces to which the gut wall is subjected.
Many other channels likely contribute to rhythmic depolarization of ICC. Part of the upstroke is associated with influx of calcium through a non-L-type calcium channel, likely a mechano-sensitive voltage-activated T-type calcium channel. These channels may be of critical importance in the generation of stimulus-dependent pacemaking activity as shown in the rat and mouse colon. ICC-MP in the mouse intestine may use L-type calcium activity in cell to cell communication and synchronization of slow waves, in the anal sphincter they may be responsible for rhythmic contractile activity delivering tone. Murine ICC also express Ca 2 + -inhibited, nonselective cation channels with a single-channel conductance of 13 pS, that are periodically activated at the same frequency as pacemaker currents. CCK, via CCK1 receptors, is thought to contribute to pacemaker activity via activation of the TRPC5 channel. Little is still known about the role of different ion channel compositions in the functioning of the different ICC subtypes.
K channels are critical for setting the membrane potential and repolarization. A dominant K channel in mouse colon ICC is the KV7.5 revealed using cell-attached patch clamp studies on isolated ICC. Single channel activity revealed voltage-sensitive K + channels, which were blocked by the KV7 blocker XE991, which also evoked inward maxi channel activity. Muscarinic acetylcholine receptor stimulation with carbachol inhibited K + channel activity. The single channel conductance was 3.4 pS. Single-cell RT-PCR revealed Ano1-positive ICC that were positive for KV7.5. Double immunohistochemical staining of colons for Kit and KV7.5 in situ revealed that intramuscular ICC (ICC-IM), but not ICC associated with the myenteric plexus (ICC-MP), were positive for KV7.5. It also revealed dense cholinergic innervation of ICC-IM. ICC-IM and ICC-MP networks were found to be connected ( Fig. 13.3 ). This study let to the proposal that the pacemaker network in the colon consists of both ICC-MP and ICC-IM and that one way of exciting this network is via cholinergic KV7.5 channel inhibition in ICC-IM.

The ICC-DMP are spontaneously quiescent. Upon stimulation by exogenous substance P, ICC-DMP first exhibit high-frequency calcium transients; this activity is not synchronized within the ICC-DMP network and does not lead to electrical activity. Upon further sustained stimulation by substance P, the high-frequency calcium transients become superimposed on strong rhythmic low-frequency calcium transients, which are synchronized within the ICC-DMP network; this is associated with rhythmic transient depolarizations. Hence, the ICC-DMP network exhibits stimulus-induced synchronized rhythmicity, leading to the hypothesis that ICC-DMP are stimulus-dependent pacemaker cells.
13.4.2
Calcium Waves
The generation of the slow wave in ICC, that is, the activation of ion channels that generate the start of the slow-wave upstroke is initiated by the rhythmic release of calcium from the sarcoplasmic reticulum. Rhythmic release is then terminated by reuptake of calcium in the sarcoplasmic reticulum via calcium pumps. A wave of a transient increase in intracellular calcium is associated with the propagating slow wave, or more precisely, the calcium transients are synchronized within the network with an apparent propagation velocity of ~ 2 mm/s, due to a calcium phase wave. Glycyrrhetinic acid, inhibiting gap junction conductance, reduced the coherence of propagation, causing single cells to pace independently. Thus, virtually all ICC-MPs are spontaneously active, but normal activity is organized into propagating wave fronts. Elegant work from the Van Helden laboratory has given us much insight into the role of calcium in pacemaker activity. Although calcium signals are synchronized within ICC networks, the synchronization is due to electrochemical coupling. Oscillatory store calcium release generates inward current through calcium-activated cell membrane ion channels with consequent depolarization; the conducted depolarization to neighboring cells then leads to activation of the release of calcium from their stores. Van Helden reasoned with modeling that diffusion of calcium or IP3 through gap junctions of the ICC network is not fast enough to be solely responsible for pacemaker synchronization. The stores then are strongly linked though cell depolarization-enhanced IP 3 R-mediated Ca 2 + release.
13.5
ICC Pacemaking in General With a Focus on the Small Intestine
In this section, many general aspects of ICC pacemaking will be discussed as well as the particulars of ICC pacemaking in the small intestine. ICC-MP in the small intestine generate slow-wave activity that actively propagates into the musculature. Hence, the small intestine musculature (both the circular and the longitudinal muscle layers) is continuously subjected to an oscillating membrane potential. In order to elucidate the consequences for motor pattern development, we need to first establish an understanding of the characteristics of peristalsis, the frequency gradient, and propagation. The acceptance of the importance of slow waves was hindered by the fact that research on the role of the enteric nervous system in motility was conducted primarily in the guinea pig and it so happens that in the guinea pig small intestine the slow wave may not be recorded under unstimulated conditions, which caused uncertainty about its importance. However, when the guinea pig small intestine is distended to evoke peristaltic contractions, the presence of slow waves is obvious and easy to demonstrate.
13.5.1
Peristalsis
Peristalsis can be defined as a motor pattern of the gut organ musculature that can propel content into the anal (antegrade peristalsis) or oral (retrograde peristalsis) direction. The term “peristalsis” can evoke distinctly different images hence it needs clarification in any discussion. The classic image is that of a bolus or pellet that slowly moves distally along the intestine. Such a bolus exerts a distinct local distention on the intestinal wall and may propagate according to the “law of the intestine,” that is the distention created by the bolus stimulates inhibitory neurons to relax the musculature in front of the bolus and stimulates excitatory neurons at the oral end of the bolus and so propels the bolus in anal direction. This is what can be called the classic peristaltic reflex . It was a major discovery and strengthened by another major discovery, the fact that enteric nerves can project both anally and orally. Immediately after the discovery of this reflex by Bayliss and Starling, it was difficult for many investigators to measure the inhibition in front of the bolus, hence this reflex, although proven to exist, did not appear to be the only mechanism that can propel intestinal content. Furthermore, under many conditions that require propulsion, there is no bolus, only slushy content or harder content of various lengths. So the term, peristaltic reflex is best reserved for bolus-induced peristalsis, and the term “peristalsis” can be used for all the other mechanisms that can propel content. Extrinsic nerves can orchestrate peristalsis: the vagus nerve excites sections of the esophageal musculature in sequence so that orderly peristalsis occurs. The migrating motor complex is a peristaltic movement that moves chyme out of the intestine at night and is orchestrated by the enteric nervous system. The rhythmicity and propagating characteristics of the peristaltic movements of the stomach are orchestrated by ICC-MP and ICC-IM. Peristaltic activity can also be evoked by enteric nerves that evoke stimulus-dependent pacemaking in a network of ICC such that the rhythmicity and propagation characteristics are dependent on ICC but its occurrence and the force of contraction dependent on enteric neural activity, illustrating the often intertwining actions of the ENS and ICC.
13.5.2
The Frequency Gradient
A key feature of ICC pacemaker-driven peristalsis is the frequency gradient of the slow waves within ICC networks. In the small intestine and stomach, there is a fixed proximal to distal frequency gradient, that is, the most proximal pacemakers have a higher frequency compared to the distal pacemakers. In the human stomach it goes from ~ 3 to ~ 2 cpm and in the human intestine from ~ 12 to ~ 6 cpm. In the colon, the frequency gradient is labile, and often in opposite direction compared to the small intestine. The frequency gradient determines the direction of propagation of the slow wave and associated contraction. The frequency gradient, however, is not static, it can easily be disrupted by local excitations leading to local increases in frequency, high-frequency islands, from which slow waves can propagate in different directions, leading to “chaotic” or segmental contractile activity.
13.5.3
Propagation
The terms “propagation” and “propagation velocity” are used to indicate that slow waves and contractions are seen to move in anal or oral direction. However, the nature of propagation of an action potential along a nerve axon, of the migrating motor complex along the small intestine, and the propagation of the slow wave in the small intestine or stomach have entirely different mechanisms underlying them.
13.5.4
The Nature of Slow-Wave Propagation and Slow-Wave-Driven Peristalsis
The slow-wave-driven peristalsis was illustrated in the proximal intestine of the mouse when contrast fluid was gavaged into the stomach and, upon entering the small intestine, produced strong slow wave directed peristalsis that kept the proximal intestine empty to be able to receive content from the stomach. This activity was absent in W/Wv mice that did not have ICC-MP. Slow-wave propagation is optimally measured using an array of electrodes. Lammers and coworkers used an array of 240 electrodes, creating high-resolution maps to accurately determine direction and velocity of propagation. Without stimulation, one witnesses a front of depolarization occurring near simultaneously around the circumference that propagates primarily in the anal direction. When a high-frequency island is in focus, then slow-waves or calcium waves propagate with a similar velocity in all directions. Several studies have recorded the arrival times of a slow wave at two electrodes located parallel to the circumference of the intestine and observed that the time difference between the two sites is minimal, which indicates nearly identical activation times but is sometimes interpreted as fast conduction velocity. The work from Lammers and others, using many very closely spaced extracellular electrodes, giving very high resolution, has given us tremendous insight into slow-wave propagation within networks. Although every technique has limitations, it is important to note that the notion that extracellularly recorded slow waves necessarily represent contraction artifacts is unfounded.
The ICC network is continuous in an organ and slow waves can propagate over the whole length of the organ. Whether or not this often happens in vivo is a different matter. After a meal, distension, or fatty acids may cause the emergence of local high frequency islands from which slow waves will propagate in all directions and which will then collide against the normal aborally propagating slow wave, thereby interrupting its normal propagation. Such induced pacemaker sites are not “ectopic,” as in “abnormal,” since they are an expression of natural properties of the pacemaker network. The notion that slow waves can propagate all along the intestine has been questioned because it appears to interfere with the main focus of the intestine, mixing and absorption. This argument assumes that a peristaltic contraction governed by the slow wave and fully accompanying the slow wave to the end of the intestine would be the dominant contraction pattern after food intake, which is not the case except for the first part of the duodenum. As shown in the mouse intestine, stomach content is rapidly moved away from the pyloric region to allow sufficient content from the stomach to enter the small intestine. After that, contractions at the slow-wave frequency rarely propagate over long distances. Measurements of slow waves propagating from one end to the other in the small intestine were carried out in vitro where stimuli can be minimized and kept relatively constant. However, in vivo, distention caused by changing and moving content, spontaneous and induced neural activity, all cause action on the ICC networks since the ICC are very sensitive to stretch and nerve action. This means that the network along the intestine is not under uniform stimulation and the noisy activation or inhibition thus created will cause areas (islands) of higher and lower pacemaker frequencies, causing oral and anal propagation of slow-wave activity which cause back and forth movements of content.
Once in the musculature, a slow wave creates an area of depolarization, limited in time and space. When the depolarization is strong enough to pass threshold for activation of voltage-sensitive calcium channels, action potentials occur within this field of depolarization; hence action potentials follow the slow wave in time. Within this propagating field of depolarization, action potentials vary in number and intensity, which is associated with the degree of force of contraction. A slow wave propagates into both the circular and longitudinal muscle layer and initiates action potentials in both. The propagation characteristics of action potentials are very different from those of slow waves. Action potentials in a patch (the depolarized part of the slow wave) can propagate in any direction but its propagation is anisotropic, that is, it is much faster in along the length axis of smooth muscle cells compared to their width axis. An action potential will terminate abruptly when it drops down the cliff of depolarization generated by the slow wave. The contractions associated with this type of action potential generation are slow-wave-driven segmental contractions, or very brief peristaltic contractions (resulting in mixing primarily) or long (resulting in propulsion of content when the force of contraction is strong enough to occlude the lumen).
The ICC are networks of cells that are coupled by connexons, often forming gap junctions. They do not form a true syncytium, in that the network does not function as a single cell; the network behavior is sometimes referred to as a functional syncytium since the cells within the network are in constant communication. There are two dominant theories as to how pacemaker activity, the slow wave propagates through this network. On the one hand, there is the “one pacemaker model” or the “cable model” that suggests that there is one dominant pacemaker, that this transient depolarization actively propagates, creating a premature slow wave in neighboring ICC (since the intrinsic pacemaker frequency of this neighboring cell will be slightly slower) and so travels along the network basically encountering passive cells (cells not yet in the process of generating a slow wave) that are ignited by the traveling slow wave, much like an action potential is traveling along an axon. The other theory of slow-wave propagation refers to a “coupled oscillator model.” Although in some discussions these models are presented as diametrically opposed; in fact, they are in most respects similar, they both rely on the basic biophysical features of the ICC and smooth muscle cells, the features of gap junction coupling, etc. Much of our knowledge gained from cable theories are, or will eventually be, incorporated in coupled oscillator theories. The key component of the coupled oscillator theory is that all ICC are seen as active pacemaker cells and that propagation comes about through synchronization of all slow waves. This means that there is no active propagation; just synchronization of activities, and the apparent propagation is caused by phase lags between oscillators created primarily by a gradient in intrinsic frequencies present along the length of the organ. That all ICC are active is consistent with the observation that after cutting the smooth muscle layer of the intestine in very small sections (a few mm 2 ), all sections will always generate electrical slow-wave activity as long as ICC-MP are present, and when ICC are chemically isolated, they show rhythmic pacemaker activity. Within the network, the activity in each ICC is influenced by the oscillations of its neighboring cells, as coupled oscillators. The key features are synchronization and entrainment. Synchronization is the tendency of two coupled oscillators to minimize their phase difference. When the phase difference between two oscillators reaches and stays at zero they are completely synchronized. Synchronization was first observed by Huygens in the 17th century between pendulums and explains how networks of oscillators can generate waves. The wave is a coordinated phase difference across the oscillator network, a “phase wave.” With a gradient in frequency across the network, the higher frequency oscillators will always be ahead of the phase of the lower frequency oscillators and so the phase wave will appear to propagate from the high to the low-frequency part of the network.
The frequency gradient is a consequence of a gradient in factors related to calcium homeostasis in ICC, the rhythm of calcium flow out of the sarcoplasmic reticulum that underlies frequency regulation in individual ICC. Entrainment is a consequence of chemical and electrical communication between individual ICC that minimizes frequency differences. It is important to note the difference between the oscillator’s natural frequency, the frequency at which it oscillates in isolation, and the frequency it oscillates at within a network being influenced by neighboring oscillators. Entrainment is when an oscillator with a higher natural frequency, “pulls up” the oscillation frequency of an oscillator with a lower natural frequency, toward its own frequency. How much depends on the strength of coupling and difference in natural frequency between the oscillators. In a chain of oscillators with a natural frequency gradient, entrainment can pull a length of the chain to the same frequency, which then forms a frequency plateau.
Both in the intestine and in intestinal models of coupled oscillators, a series of plateaus is observed, with intervening steps where the frequency difference becomes too great for entrainment. At this step, one plateau changes into another plateau with a reduced frequency, because one or more slow waves terminate, a phenomenon called a dislocation ( Fig. 13.4 ). The characteristics of the plateaus and dislocations are dependent on the coupling strength between ICC since the gap junction inhibitor carbenoxolone increased the number of plateaus and dislocations and decreased wave velocity. Although carbenoxolone did influence cell to cell coupling, it did not abolish it, suggesting that current gap junction blockers are not capable of fully blocking electrical communication or that communication other than through gap junctions is present.

Carbenoxolone not only increased the number of plateaus but is also created a “chaotic” pattern of propagation of the circular muscle contractions that were governed by the slow wave. Hence, inhibition of gap junction conductance can change a peristaltic motor activity into a segmentation motor activity that favors mixing and absorption. A model of weakly coupled oscillators can explain this. When spatial noise was added to the natural interval gradient, as gap junction conductance decreased, the number of plateaus increased as before, but in addition, the phase waves frequently changed direction of apparent propagation, mimicking the effect of carbenoxolone. Hence, key features of the motor patterns that are governed by pacemaker activity are likely a direct consequence of biological noise, specifically spatial noise in gap junction coupling and pacemaker frequency. The potentially important role of dynamic network properties as a regulating feature of motor pattern development was further strengthened by the development of a two-dimensional network of coupled oscillators and direct experimental comparison with electrical features of the cat and mouse intestine. The occurrence of dislocations, frequency plateaus, and the slowing of the propagation velocity distally was shown to be natural consequences of spatial and frequency noise in a network of coupled oscillators with a strong frequency gradient as that occurs in the cat intestine.
13.5.5
The Minute Rhythm Pattern of Peristalsis
The slow-wave activity in the small intestine is omnipresent; hence, the musculature is constantly exposed to rhythmic changes in membrane potential. This influences all motor patterns, but the classic slow-wave-driven peristalsis, is not the only motor pattern of the small intestine, and not the most powerful pattern that produces peristalsis. Under many conditions, this slow-wave-driven peristaltic activity appears to be of low amplitude and in such conditions it is often referred to as “ripples.” Strong peristaltic activity can occur with a “minute rhythm.” When this occurs, a rhythmic depolarization progresses through the intestine at a propagating velocity that is much slower than that of the slow wave. Such a rhythmic depolarization will crank up the depolarization level of the slow waves which occur at the same time which will appear superimposed on this slow depolarization, and more action potentials will be generated within the depolarized “patches” of these slow waves such that the minute rhythm will generate forceful propulsive contractions, bands of depolarization that propagate according to the propagation characteristics of the slow depolarization. Within the band of depolarization, slow-wave-driven peristalsis occurs, just as within the migrating motor complex under fasting conditions. The minute rhythm of peristalsis in powerfully shown in a high-resolution manometry recording of the human small intestine.
The minute rhythm has been observed in many studies and it considered neurogenic. Hence, the rhythmic depolarization that is experienced by the musculature either comes from pacemaker neuronal circuitry or from neurally induced rhythmic activity in ICC, possibly the ICC-DMP that are extensively innervated by excitatory neurons. The propagation velocity of the minute rhythm in different studies is highly variable and can be relatively slow at ~ 1 cm/s or almost instantaneous, suggesting that more than one mechanism may underlie the minute rhythm.
13.5.6
Slow-Wave-Driven Segmentation
Cannon described the intestinal motor pattern that shows local transient contractions that are not propagating. Alvarez showed them to be contracting as the slow-wave frequency. When the segmentation motor pattern occurs, it was shown that a second electrical oscillatory activity becomes active, a rhythmic wave of depolarization at a much lower frequency. It was hypothesized that this activity comes from ICC-DMP and calcium waves at this lower frequency were demonstrated. It was shown that interaction of the slow wave and this second rhythmic depolarization through phase amplitude coupling creates a waxing and waning electrical activity in smooth muscle that creates the segmentation motor pattern. This phenomenon could be perfectly reproduced in a mathematical model of coupled oscillators. The stimulus of the ICC-DMP-induced oscillatory activity can be distention or neural activity and with neurally induced ICC activity, the distinction between myogenic and neurogenic motor patterns falls apart, the control systems of ICC and nerves are inextricably linked.
In the small intestine, absorption of nutrients is essential and hence, in the presence of content, motor patterns should serve mixing and absorption. The mechanism by which the classic “Cannon-type” segmentation is achieved has been described above. But other mechanisms are likely in play to achieve hours of absorption-promoting activity. In studies on the empty isolated unstimulated intestine, slow waves travel anally. When Seerden et al distended different parts of the small intestine of the mouse, the slow-wave pattern changed so that slow waves were “initiated” in random places and they then propagated in both directions. There was perfect synchronization between slow waves and circular muscle contractions and distention, hence motor patterns change caused by local distention that creates high slow-wave frequency islands from which slow waves and hence contractions propagate in both directions. Local actions of chronotropic agonists, excitatory neurotransmitters, prostaglandins, will cause an increase in the local pacemaker frequency with marked effects on motor activity. In the stomach, it was shown that prostaglandins or EP3 agonists act on the prostaglandin EP3 receptor on ICC-IM to increase slow-wave activity and to change direction of propagating contractions. This also happens with vagal nerve stimulation. Local excitation by acetylcholine acting on M3 receptors on ICC, increasing IP3, increases the frequency of pacemaker current and slow waves in isolated ICC. In the small intestine, stimulation of enteric sensory nerves affects ICC-MP pacemaker activity, indicating that local activation will disturb ongoing anal propagation and enhance absorption. Hence, the intestinal pacemaker activity in ICC is very sensitive to stimuli that can increase local slow-wave frequency, which when it happens at many points along the intestine, will give erratic back and forth movements that promote mixing and absorption. In the stomach, this is much less likely to occur, probably because of a stable frequency gradient and strong intercellular coupling although propagation directions other than aboral are not infrequently encountered in healthy pigs in vivo and other animals as already observed by Alvarez.
In addition to specific motor patterns promoting absorption, peristaltic activity most often serves this function as well. The prime example is the stomach where slow-wave-driven peristaltic contractions pounce content against the pylorus with all or the majority of content being pushed back and hence, in the stomach, the peristaltic contractions are the major force behind mixing, grinding, and absorption. Without opening of the pylorus, there is little net transit.
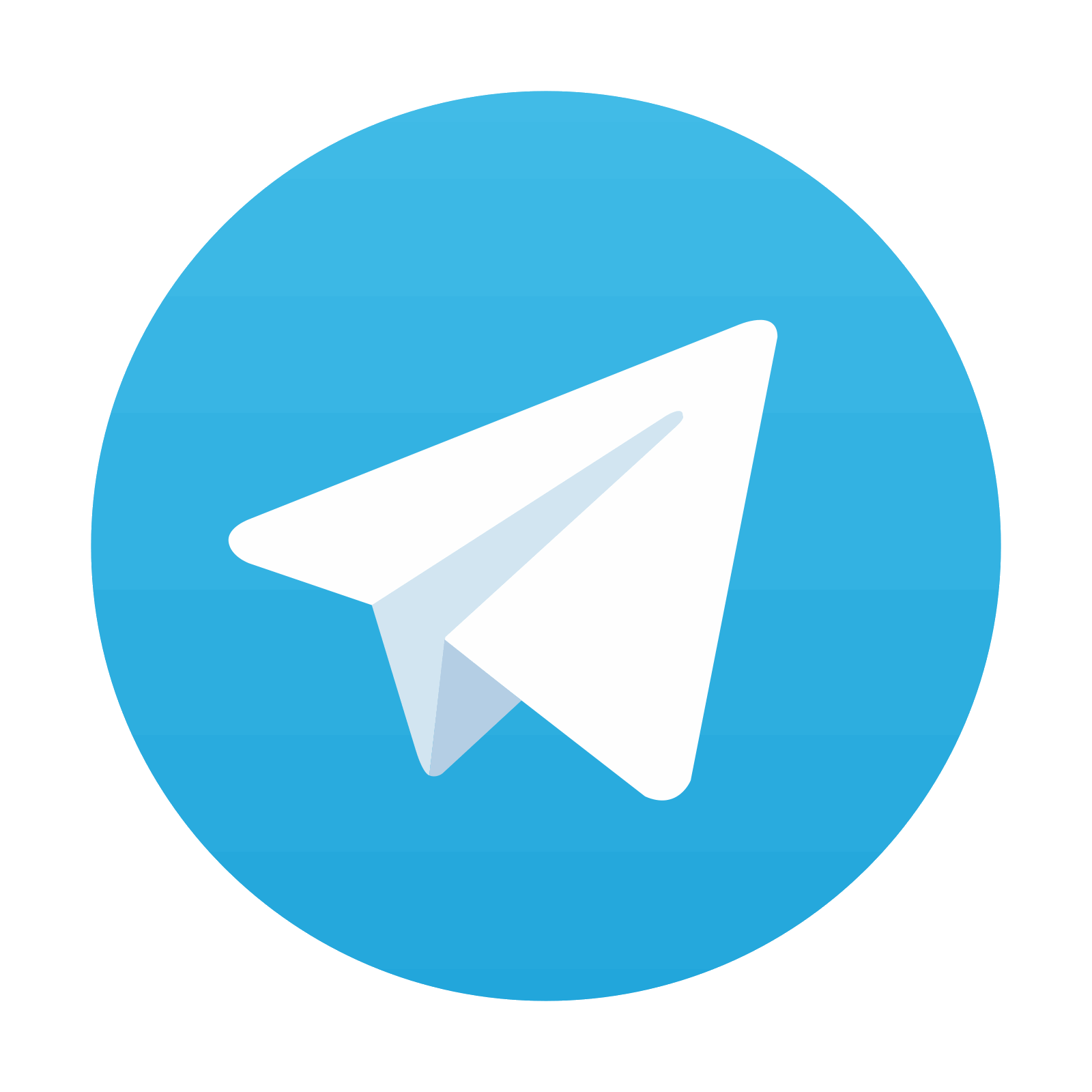
Stay updated, free articles. Join our Telegram channel
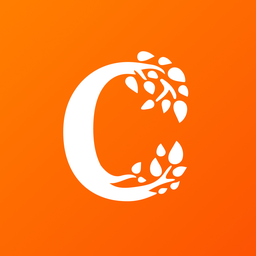
Full access? Get Clinical Tree
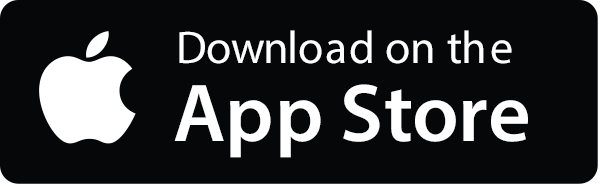
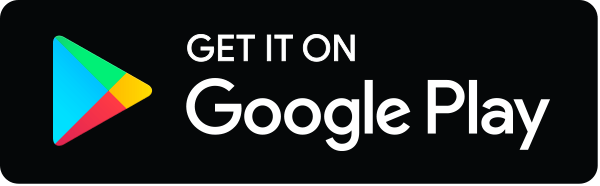