Abstract
Visceral information reaches the brain through a diverse array of neural, humoral, and immune pathways, conveying an abundance of ascending visceral information that helps to maintain homeostasis within the gastrointestinal (GI) tract (Johnson and Gebhart). While the vast majority of these physiological processes are subconscious, those that do enter consciousness include sensations such as hunger, satiety, and the urge to defecate. Contrastingly, at the extreme of this GI sensory spectrum is visceral pain, engendered by a complex interaction between the intraluminal milieu and a plethora of host factors that, in turn, comprise the multifaceted neurobehavioral system. The GI sensory spectrum invokes activity in numerous subcortical and cortical structures via projections from vagal and spinal afferents, which cumulatively results in an individual’s unique sensory experience (Willis et al. and Derbyshire). This chapter will focus on the processing of GI sensation within the brain in health that results in conscious perception with particular attention being paid to the neuroanatomical representations that have been derived from recent functional neuroimaging studies.
Keywords
Gastrointestinal sensation, Afferent signaling, Brain
16.1
Introduction
Visceral information reaches the brain through a diverse array of neural, humoral, and immune pathways, conveying an abundance of ascending visceral information that helps to maintain homeostasis within the gastrointestinal (GI) tract. While the vast majority of these physiological processes are subconscious, those that do enter the consciousness include sensations such as hunger, satiety, and the urge to defecate. Contrastingly, at the extreme of this GI sensory spectrum is visceral pain, engendered by a complex interaction between the intraluminal milieu and a plethora of host factors that, in turn, comprise the multifaceted neurobehavioral system. The GI sensory spectrum invokes activity in numerous subcortical and cortical structures via projections from vagal and spinal afferents, which cumulatively results in an individual’s unique sensory experience. This chapter will focus on the processing of GI sensation within the brain in health that results in conscious perception with particular attention being paid to the neuroanatomical representations that have been derived from recent functional neuroimaging studies.
16.2
Neuroanatomy of GI Sensation
Traditionally, vagal and spinal afferents that innervate the GI tract have been considered to hold distinct roles in mediating GI sensation. For instance, spinal afferents have historically been considered to encode the perception of pain and inhibition of the digestive process in contrast to vagal pathways, which sensed hunger, satiety, and reflex control. However, given the marked multilevel supraspinal convergence of these pathways it is likely that this view is an over-simplification. For example, although vagal afferents synapse in the nucleus of the solitary tract (NTS) in the brainstem, this major integrative visceral sensory relay nucleus also receives inputs from spinal afferents as well as other brain regions. Subsequently, the principal projections from the NTS are to the parabrachial nucleus, within the pons, which receives further visceral sensory input from several spinal dorsal horn laminae. From the parabrachial nucleus, projections ascend toward higher structures including the hypothalamus, thalamus, insula, amygdala, prefrontal cortex (PFC) as well as many other cortical regions; see Fig. 16.1 for a schematic representation.

16.3
Thalamus
The thalamus is the largest structure in the dorsal portion of the brainstem and comprises multiple nuclei arranged into six groups in each thalamic hemisphere. Within these groups, nuclei can be broadly divided into two functional types: relay (specific) nuclei and diffuse projecting (nonspecific) nuclei. Relay nuclei convey information between subcortical and cortical regions and mediate perception, voluntary movement, emotion, and language. In contrast, diffuse projecting nuclei receive numerous converging sources of neural input, and project to numerous cortical regions.
16.3.1
Lateral Thalalmus
Within the lateral portion of the thalamus, neurons in both the ventroposterolateral (VPL) and ventroposteromedial (VPM) respond to noxious sensations. The receptive field of VPL displays somatotropic organization, although visceral input into the VPL is not visceroptopic . Projections from the lateral thalamic nuclei are believed to mediate the sensory discriminatory aspects of pain perception. However, this viewpoint has been challenged with the proposition that this discriminatory function is arguably more attributable to the thalamic area that contributes to the posterior part of the ventromedial nucleus. Nuclei in the ventroposterior inferior (VPI) and medial part of the posterior complex contain nociceptive neurons that project toward the insula and secondary somatosensory cortex (S2). In humans, Lenz et al. showed in a single patient with a background history of angina that micro-stimulation of the VPL nucleus reproduced angina-like symptoms but also concomitant paresthesia in the leg, suggesting that the VPL has a role in both visceral and somatic sensation.
16.3.2
Medial Thalamus
Studies have demonstrated that nuclei within the medial thalamus have large bilateral receptive fields. They do not directly contribute to the localization of pain but do encode its relative intensity, suggesting that they have a role in some aspects of sensory discrimination. The ventromedial nucleus contains nociceptive neurons which respond to noxious mechanical, heat, and cold stimuli. Albeit with relatively small receptive fields, these neurons are somatotopically organized and project primarily to the insular cortex as well as the primary somatosensory cortex (S1). In addition, projections from the medical thalamic nuclei also reach the PFC and the anterior cingulate cortex (ACC), areas well characterized as important in the processing cognitive and emotional aspects of pain. The ventromedial nucleus also receives input from the vagus-NTS-parabrachial pathway, which projects onwards toward cortical areas that receive inputs from lamina I neurons from the spinal dorsal horn. Lamina I neurons have been implicated in homeostatic regulation and indeed pain has been regarded by some as a “homeostatic emotion.” Interestingly the vagus nerve also serves a function similar to lamina I neurons in the neurophysiology of the GI tract, with the exception that it does not assist in the pure sensory encoding of noxious sensation. The vagus has inputs into the thalamic reticular nucleus which itself projects to other thalamic nuclei, influencing the synchronicity of thalamocortical projections and to the intralaminar nucleus of the thalamus, which has widespread projections to the striatum and cerebral cortex.
Overall, the thalamus plays a central integrating and relay role in the process of nociceptive and homeostatic information to higher centers of the brain. Moreover, it also interacts with descending pathways that modulate the autonomic nervous system and visceral sensation.
16.4
Cerebral Cortex
Although the thalamus extensively projects toward the cerebral cortex, until relatively recently the role of the cortex in pain processing has remained rather uncertain. However, by utilization of human functional neuroimaging, a wealth of evidence has been provided, illustrating that the cerebral cortex plays a central role in sensory-discriminative and affective components of the pain experience. These subsequent sections provide a summary of the role of the S1 and S2, as well as the insula and cingulate cortices.
16.4.1
Primary Somatosensory Cortex
In humans, lesions within S1 have been shown to result in hyperalgesia, hypoalgesia, and no difference in pain perception. However, this seemingly contradictory evidence may be partially explained by the relative size and location of the lesion, as nociceptive neurons are not uniformly distributed in S1. It should be noted that S1, which importantly includes the cortical sensory homunculus, predominantly processes nonnoxious external somatosensory information, such as pressure and warmth, and thus neurons that encode tactile sensation far outnumber their nociceptive counterparts. There are plentiful projections from S1 to the VPL and medial thalamic nuclei; these neurons are somatotopically organized and have limited receptive fields, with activity directly correlated with the intensity and duration of the noxious stimulation. Taken together, these data suggest that nociceptive neurons in S1 encode the sensory discriminatory aspects of pain.
16.4.2
Secondary Somatosensory Cortex
S2 is located in the parietal operculum in the upper aspect of the Sylvian fissure. In contrast to tactile sensation, the processing of pain in S1 and S2 occurs concurrently. S2 receives bilateral input from the VPI thalamic nuclei, which have bilateral receptive fields. Projections from S2, via the insula, enter the limbic structures of the temporal lobe. S2 is considered to be involved in the recognition of stimulus type and the cognitive-evaluative aspects of the pain experience. Evidence from lesion studies demonstrate that disruption of S2 limits the ability to identify the nature of noxious stimuli such that subjects cannot differentiate between mechanical or heat pain.
16.5
Insula
The insula (or insular cortex) yields several complex roles in pain processing that are incompletely understood, but are postulated to include interoceptive awareness and emotion. The insula has two distinct regions. Firstly, the granular posterior insula processes tactile, auditory, and visual somatosensation. Secondly, the dysgranular anterior insula is involved in olfactory, gustatory, and viscero-autonomic functions. It receives projections from S2 and the posterior ventromedial (VMpo) nucleus of the thalamus, and has been shown to be activated by visceral stimuli. As mentioned, the insula receives input from S2 but also direct projections from nociceptors within the VPM of the medial thalamus. Nociceptive neurons within the insula have large receptive fields that respond to different modalities of sensory simulation but are particularly activated by noxious visceral stimuli. The insula is thought to integrate information from the thalamus and somatosensory cortices (both S1 and S2), relaying this to limbic regions. Indeed, the insula has been termed the visceral sensory cortex. Direct stimulation of the anterior insula produces sensations of nausea and fullness in contrast to the posterior insula, which produces somatic sensation including pain.
16.6
Cingulate Cortex
The cingulate cortex, consisting of the anterior, medial, and posterior divisions, is an extensive area of the limbic system. There is growing evidence surrounding the role of the ACC in the processing of visceral and somatic sensation. The perigenual ACC has functional connections with the brainstem autonomic nuclei, and is involved in visceromotor control and the modulation of autonomic and emotional responses to aversive stimuli. For instance, in patients who have had therapeutic bilateral cingulotomy, there is a reduction in the affective and intensity ratings of noxious heat and cold stimuli, thus providing experimental support for the role of the cingulate cortex in the perception of innocuous and noxious thermal stimuli. See Fig. 16.2 for a schematic representation of ascending pathways, subcortical structures, and cerebral cortical structures involved in processing pain.

16.7
Additional Brain Regions
There are numerous other brain regions implicated in the brain processing of visceral pain, which include the dorsolateral prefrontal cortex (DLPFC), posterior parietal cortex, primary motor (M1), supplementary motor area (SMA), hypothalamus, amygdala, hippocampus, cerebellum, and basal ganglia (see Fig. 16.2 ). Taken together, a complex brain network for visceral pain is evident, albeit far from comprehensively understood. The following section will discuss the contribution of functional neuroimaging on the current understanding of the processing of GI sensory signals.
16.8
Neuroimaging Modalities
Over the past three decades, the use of rapidly advancing neuroimaging methods has significantly broadened our understanding of how the brain processes GI signals. In particular, functional brain imaging permits a noninvasive method to record brain activity in both human and animal models in real time, so as to study a GI stimulus and associated brain activity. Functional imaging encompasses multiple modalities, and includes functional magnetic resonance imaging (fMRI), positron emission tomography (PET), electroencephalography (EEG), and magnetoencephalography (MEG). The utility of these methods differs as each holds particular advantages and disadvantages which, depending on one’s experimental design, may merit their use over another modality. For example, EEG and MEG utilize electromagnetic signals, permitting high temporal resolution of brain neural processes (often to a remarkable timescale of 10–100 ms), although EEG yields have a poor spatial resolution (ranging from one to several centimeters). The converse is largely true for PET and fMRI, both of which take advantage of the brain’s changes in regional blood perfusion either by the use of injected magnetic resonance contrast agents or by the distinct magnetic properties of oxygenated and deoxygenated hemoglobin. PET and fMRI yield a superior spatial resolution in the range of millimeters, yet a temporal resolution in the region of seconds. These methods, along with their advantages and disadvantages, will be discussed in more detail below, although for more detailed and technical information we would also direct the reader to the following references.
16.9
Functional Imaging
16.9.1
Electroencephalography and Magnetoencephalography
Both EEG and MEG are neuroimaging modalities that allow one to directly measure brain electrical activity to a highly accurate temporal resolution within the range of milliseconds. The relative low cost and ease of use strengthen its use clinically. As of their temporal resolution, both MEG and EEG are largely used for stimulus-evoked potential experimental designs, whereby one can elucidate cortical regions receiving information, inferred by capture of signal, be it electrical or magnetic. MEG specifically detects magnetic activity in the brain, and is both proportional and orthogonal to local electrical activity. The MEG signal is thought to reflect primarily intracellular currents that flow though the apical dendrites of pyramidal cells running parallel to the skull surface. Per each cubic millimeter of the brain cortex there are more than 100,000 pyramidal cells, each with thousands of synapses generating a neuromagnetic signal. The signal from a single synapse is too small for MEG detection. In fact, typically in the region of 1 million synapses synchronously active are required for MEG signal detection. However, due to the high cortical synaptic density, MEG signals can be measured from relatively small areas of the cortex. Furthermore, both gyral geometry and local electro-magnetic field orientation influence whether evoked potentials are better detected by MEG or by EEG. As described above, while EEG permits data collection to a highly temporal specificity, it is limited by spatial resolution, such that the specific localization of a signal to a brain region may be difficult to ascertain. Furthermore, imaging of deep brain regions is limited by the distance between region and signal sensor. That being said, these are continually advancing and improving neuroimaging methods. For further reading of both MEG and EEG we direct the reader to Refs. .
16.9.2
Positron Emission Tomography
PET utilizes positron-emitting radionucleotides to infer brain activity. These radionucleotides are intravenously injected into a subject and captured by a specialized detection system the subject is positioned inside. There are numerous usable radiolabelled compounds, and these can be used as biomarkers for metabolic processes such as neuronal glucose uptake. This captured signal may then be used as a surrogate for neuronal activity. In GI research, the most commonly used radionucleotide is 15 O, prepared as H 2 15 O-labeled water. This method creates perfusion images, illustrating brain blood flow. Cerebral blood flow has also been used as a surrogate marker of brain activity (including in fMRI, see below), and changes in regional cerebral blood flow (rCBF) are well correlated both with regional glucose consumption illustrated in PET and with neuronal activity. The nature of the PET modality permits a spatial resolution in the range of millimeters, yet a temporal resolution within the region of seconds. This allows for experimental designs that compare images between different conditions, such as between a GI stimulus and rest, including both block- and event-related designs. The limitation of PET, however, is that it relies on the use of intravenous radionucleotide compound injection to allow any signal acquisition, which additionally is associated with problems in repeatedly scanned individuals.
16.9.3
Functional Magnetic Resonance Imaging
fMRI infers neuronal activity on the premise that for a brain area to be more active there will be an increase in local regional arterial blood flow. Originally, this method was undertaken using contrast agents ; however, it was later shown that by taking advantage of the disparity in magnetic properties of oxygenated and deoxygenated hemoglobin images could be acquired without the need for contrast. Most commonly, blood oxygen level dependent (BOLD) is the fMRI technique of choice, in that deoxygenated hemoglobin is paramagnetic compared to the diamagnetism of oxyhemoglobin. Although it lacks the superior temporal resolution of EEG and MEG, it arguably represents an overall balanced technique with both good temporal and spatial resolution, with the ability to repeatedly study human subjects, and is likely the most common neuroimaging modality adopted in current practice. As an alternative to the use of BOLD, arterial spin labeling (ASL) is becoming increasingly popular. In ASL, the water content of arterial blood is magnetically labeled as a form of tracer, which when exchanged with tissue water, alters total tissue magnetization and produces an MR signal and image.
fMRI permits “brain activity” maps, illustrating regions of the brain inferred to be more active by means of the disparity in magnetic properties imaged by BOLD or ASL. However, the use of fMRI has evolved significantly over the past decade with the advent of functional connectivity and further complex measures. In functional connectivity, mathematical image intensity (such as with BOLD) is extracted from multiple regions and correlated to produce brain “connectivity” maps, whereby it is inferred that if two regions have comparable activity patterns in a time series they may, by some means, be functionally connected. Connectivity analysis permits an alternative tangible result from mere brain “activity,” as it illustrates how (and which) regions may be interacting secondary to a stimulus (e.g., with visceral pain). These methods have advanced further by means of network analysis, whereby the connectivity patterns of multiple regions are accounted for to produce a brain network (as opposed to functional connectivity that infers connection between two brain regions only ). Increasingly, “network based statistics” have become a key area in functional imaging, not least with further graph-theory-based techniques permitting the understanding of how networks differ depending on human conditions or a given variable. These complex methods are beyond the scope of this chapter, but are explained in greater detail here.
16.10
Functional Neuroimaging of GI Sensation
Over the past three decades, there have been numerous studies using functional neuroimaging to investigate GI sensation. These range from that of nociception to subliminal stimulation. By and large , with repeated study it has become apparent that somatic and visceral pain broadly activate a similar collective of cortical and subcortical brain regions. For example, Strigo et al. have previous shown that both esophageal distension and cutaneous heat stimulation activate a similar set of brain regions, including the anterior insula, somatosensory cortex, basal ganglia nuclei, and thalamus. That being said, differences have been elucidated, both comprising specific regional brain activity and functional connectivity. Furthermore, numerous studies have investigated the interindividual factors that appear to influence the brain processing of visceral sensation, including that of physiological and psychophysiological differences. Herein, we will review the neuroimaging literature pertaining to the visceral pain “neuromatrix,” which can be viewed in Fig. 16.2 . Numerous stimulation paradigms have been adopted to investigate the brain signature of sensation to a myriad of visceral regions, and include but are not restricted to that of the esophagus, stomach, rectum, bladder, and vagina. Typically, these bodily sites are stimulated by means of an elasticated balloon which is insufflated automatically or manually, but in addition electrical stimulation or even conceptually interesting stimulants such as fatty acid infusion have also been used. Fig. 16.3 shows a typical fMRI image depicting regions of cortical regions of activity commonly observed in studies of GI sensation.

16.10.1
Thalamus
The thalamus has been extensively studied previously with regard to the processing of pain signals. Interestingly, thalamic brain activity is reported only in approximately half of the studies, most of which refer to a noxious visceral stimulus. Using PET and a gastric distension stimulus, Ladabaum et al. report significant thalamic activity only to the highest distension volumes that the stimulus was noxious. In particular, the bilateral VPL nuclei and left dorsomedial nuclei were noted to show increased activity, such that the authors concluded that painful gastric distension stimulates both medial and lateral pain systems. In a later fMRI study, these findings were confirmed, showing that thalamic activity is not “dose-dependent” by means of increasing visceral stimulation intensity from nonpainful to painful.
As briefly introduced above, psychophysiological differences have been shown to influence brain activity with GI pain. Thalamic activity is no exception to that, whereby Rosenberger et al. have shown using fMRI that increased psychological stress was associated with increased left thalamic activity during painful rectal distension in healthy subjects. To reiterate the nondose-dependent activity of the thalamus described above, the effect of stress was additionally only associated with thalamic activity during painful distension, adding further weight to the specific function of the thalamus in processing noxious visceral signals only. Furthermore, thalamic activity has been shown to be influenced by personality traits during pain, whereby Coen et al. have shown that greater thalamic activity was apparent in higher neuroticism subjects during the anticipation of a painful esophageal stimulus, but decreased activity during the pain itself. Neuroticism is an umbrella term in Eysenck’s “Trait Theory,” associated with traits of high anxiety or negative affect, and Coen’s study showed that personality influences thalamic activity not only during pain, but also during the anticipation of the stimulus.
16.10.2
Primary Somatosensory Cortex
Similar to that of the thalamus, activation of the S1 during experimental visceral pain is also seen in approximately half of the studies. The reason for this unreliability of S1 activation is thought attributable to the stimuli paradigms used, which are not compatible with response characteristics of S1 neurons. Interestingly, one of the major differences shown between comparing somatic and visceral sensory stimulation is that, while somatic stimulation will evoke activity in the contralateral S1, visceral sensory stimulation appears to consistently evoke activity bilaterally, which spreads diffusely from superior (trunk representation) to inferior (intraabdominal representation) S1 subregions. Neuroanatomically, this is explained by the fact that spinal afferent signals that originate from visceral regions such as the esophagus are divergent, and thus enter the central spinal cord at multiple dermatomal levels. Physiologically, this explains the diffuse nature and poor two-point discrimination characteristic of visceral sensation.
fMRI has been used to investigate the topographic differences between disparate body regions, for instance the brain processing differences between the anorectum and the esophagus. Hobday et al. showed that rectal (visceral) stimulation activated the inferior S1 bilaterally, while anal canal (somatic) stimulation activated S1 more superiorly. Further study has shown that visceral sensation is primarily represented in S2, while somatic sensation has a clearer topographical map for S1. Having said that, both the work of Aziz et al. and Hobday et al. do elude to an S1 viscerotopic organization for GI sensation. Moreover, MEG studies have shown that esophageal sensation is processed throughout S1, but that this activity occurs in parallel to that of S2 and additionally the posterior insula. Notably this is similar to the processing of somatic pain, but in contrast to tactile processing whereby a temporal sequence of S1 sensory activity before S2 is noted.
The visceral sensory processing properties of S1 in many ways contrast to that of the thalamus. Firstly, the S1 appears more sensitive in activating following visceral stimuli. In fact, it has been shown that even subliminal intensities of rectal distension can evoke S1 activity, such that S1 activity was noted before subjects even perceived the stimulus (i.e., prior to liminal and supraliminal level). Secondly, a study using fMRI suggests that S1 activity follows a more “dose-dependent” pattern following visceral sensation. In this study, Coen et al. showed that with four incremental levels of esophageal stimulation, ranging from simple sensation to pain, bilateral S1 activity was evoked, which increased with increasing sensory stimulus, thus suggesting a role for S1 in the sensory-discriminative processing of visceral sensation. The S1 is also suggested to affect the attentional modulation of visceral pain, and also plays a role in the processing of food-related signals whereby decreased activity is noted in S1 following nutrient infusion.
16.10.3
Secondary Somatosensory Cortex
We briefly introduced the visceral sensory roles of the S2 above, and how these appear to differ from that of the S1. The S2 receives afferent fibers from S1 and the thalamus, and is suggested to have a role in the secondary processing of an incoming sensory signal, after the initial processing is computed in the S1. In addition, the S2 also may be involved in mediating attention to a painful stimulus, as opposed to the sensory discriminative aspect of sensation, which may invoke an aversive reaction as a protective mechanism so as to avoid harm. The S2 additionally has efferent projections to the primary motor cortex, which strengthens the above notion. Lastly, lesion studies of primate models have suggested that SII contributes to texture and shape discrimination (somesthetic function).
16.10.4
Insula Cortex
As described above, the insula has been a region of interest in visceral sensation and interoception for some time now. It is further subdivided into anterior and posterior subcompartments, with differing roles suggested for each.
16.10.5
Anterior Insula
While in somatic sensory studies the anterior insula has been shown to have a role in encoding stimulus intensity, it additionally is shown to be modulated by changes in the emotional state the person is in during the perception of pain. For instance, activity of the anterior insula increases when esophageal stimulation is accompanied by a negative emotional context, compared to a neutral one. In addition, insula activity itself appears to be influenced by psychological factors. Subjects with high neuroticism scores have been shown to have increasing anterior insula activity during the anticipation of visceral pain and decreasing during the pain itself. Furthermore, subjects with high extraversion scores display greater anterior insula activity during both anticipation of visceral pain and its actual experience. Interestingly, while bilateral insula activation is seen in most studies of visceral sensation, emotional factors appear to affect only the right insula. This influence of psychophysiological variables is not only confined to GI sensation, and has also been shown in studies of somatic sensation. Craig et al. have suggested that the right anterior insula is particularly important in the evaluation of internal feelings with emotional significance, including that of unpleasantness, threat, and the notion of need to withdraw from the stimulus. These studies of the right anterior insula also further suggest a role in interoception, that is to say one’s understanding of the physical and emotional state of the body. In summary, data suggest a role for the right anterior insula in the psychological response to visceral pain and interoception.
16.10.6
Posterior Insula
In contrast to that of the anterior insula, its posterior aspect is not typically activated by visceral stimulation, nor does it appear to be influenced by attention or emotion. Moreover, the role of the posterior insula appears centered around aspects of somatically painful events, including that of sensory recognition and intensity encoding, specific localization, and learning and memory of the stimulus experience.
As discussed above, MEG provides the ability to establish accurately the temporal sequence of neural signals, and indeed has been used to study the insula cortex. Specifically, it has been shown that, following painful esophageal stimulation, activity in the posterior insula occurs approximately 20 ms earlier than in the anterior insula. Although the anterior and posterior insula have dense interconnections, the significant disparity in signal latency and activation between these two subregions likely illustrates their differing roles in aspects of the pain experience.
16.10.7
Anterior Cingulate Cortex
Like many of the aforementioned brain regions, the ACC is also an “umbrella-term” for a series of multiple subregions. On the whole, the ACC is typically activated during both somatic and visceral pain, and most commonly activation is localized to the mid, rostral, and perigenual cingulate subregions. The ACC is a frequently studied area in not just pain research, but also in research related to emotion, attention, mood, and sensory intensity. Buchel et al. explored the functional roles of the different ACC subregions and demonstrated an increase in rCBF to the dorsoposterior ACC with incrementally increasing stimulus intensity, leading the authors to suggest that the region is associated with sensory processing. Contrastingly, the dorsoanterior ACC rCBF increased only between no sensation and innocuous sensation, suggesting a role of cognitive processing. Lastly, an aspect of the ventroposterior ACC was suggested to be specific for pain, as increased signal strength correlated with increasing pain intensity. Fig. 16.4 shows brain areas that increase in activity to increasing levels of GI sensation ranging from nonpainful to painful.
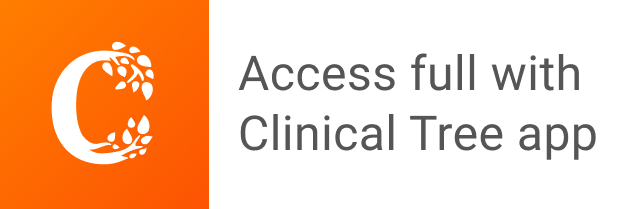