Fig. 5.1
Structural relationship between the luminal and mucosal microbiota and the enteric nervous system (From Reigstad CS, Kashyap PC. Beyond phylotyping: understanding the impact of gut microbiota on host biology. Neurogastroenterol Motil. 2013;25(5):358–72, with permission)
Bacterial toxins mediate a wide range of effects on motility through different cells, receptors, and mechanisms. Early studies with purified cholera toxin [15] and conditioned media from either toxigenic Escherichia coli [52] or Clostridium difficile [53] provided direct evidence that bacterial secretion products can enhance intestinal myoelectric activity and accelerate transit. However, not all toxins function similarly. C. difficile toxin A inhibits small bowel motility [54] and evokes capsaicin-sensitive afferent neuron and immune cell responses [55]. Cholera toxin excites multiple contractile circuits, affecting propulsive and segmentation reflexes via separate pathways [56].
Bacterial cell wall components, which may be toxic, may also affect motility. In a rat model of endotoxemia-induced dysmotility, intravenous administration of E. coli-derived lipopolysaccharide (LPS) increases activity of nitric oxide synthase, delays gastric emptying, and accelerates small bowel transit [57]. An elegant set of studies evaluated germfree mice, mice with antibiotic-depleted microbiota, and mice lacking components of LPS receptor signaling (toll-like receptor 4 or its adaptor protein Myd88). Each of these three “LPS-deficient” animal models exhibited delayed intestinal motility and reduced numbers of nitrergic neurons. LPS was also shown to increase survival of neuronal cells in what appears to be a nuclear factor-KB (NF-KB)-dependent mechanism [58]. In a separate model, intraperitoneal administration of LPS induced nuclear translocation of NF-KB in mouse intestinal smooth muscle and myenteric plexus cells [59]. Furthermore, recent studies suggest that LPS induces muscularis macrophages to increase their expression of bone morphogenic protein 2, which in turn influences motility via receptors on enteric neurons in a pSMAD-dependent fashion [60].
Microbiota-produced nontoxic compounds also affect host motility. It has been known for decades that some unicellular organisms produce biologically active hormones [61]. For example, Bacillus subtilis synthesizes a bioactive somatostatin-like molecule [62], and multiple pathogenic bacteria produce γ-aminobutyric acid (GABA) [63]. Gnotobiotic mice “humanized” by colonization with a simplified human-derived microbiota then given either Lactobacillus paracasei or Lactobacillus rhamnosus had elevated urine concentrations of the metabolite tryptamine [64]. Tryptamine is an aromatic amino acid compound that enhances intestinal contractility in ex vivo preparations and stimulates the release of other neurotransmitters [65]. Clostridium sporogenes was recently discovered to have a tryptophan decarboxylase enzyme capable of synthesizing the neurotransmitter tyramine. Microbial tyramine synthesis is now believed to be present in the intestinal tracts of more than 10 % of the human population [66]. Other studies have linked gut bacteria to increased levels of the enteric gaseous neurotransmitters hydrogen sulfide [67] and nitric oxide [68, 69].
Microbes also can receive signals from the host neurobiological environment. Quorum sensing is the microbial regulation of gene expression in response to fluctuations in cell population density [70]. Quorum sensing could provide an evolutionary explanation for the presence of a GABA uptake system in a Pseudomonas species [71], if this system evolved to detect the density of other GABA-producing bacteria. However, bacterial functions are likely influenced by differing concentrations of GABA derived from the host as well. In turn, there are multiple examples of human catecholamines that influence a range of bacterial processes including growth, attachment, and virulence [72] (Fig. 5.2).


Fig. 5.2
Influence of microbiologic and neurologic factors on microbiota population structure and function (From Lyte M. Microbial endocrinology and infectious disease in the 21st century. Trends Microbiol. 2004;12(1):14–20, with permission)
Short chain fatty acids (SCFAs), which are fermentation products that fuel intestinal epithelial cells in a site-specific and dose-dependent manner [73], are the most extensively studied class of microbial-derived molecules that influence host motility. Since the discovery that intraluminal infusion of SCFAs stimulates local motility in the human distal intestine [74], key mechanistic insights have been revealed using in vivo and ex vivo models from rat [75–79] and guinea pig [80]. One potential mechanism by which SCFAs affect motility is by increasing choline acetyltransferase activity in myenteric neurons through a monocarboxylate transporter 2-dependent mechanism. Altered choline acetyltransferase activity was found in rats given either butyrate or a resistant starch diet [81]; the resistant starch may act as a prebiotic to increase the numbers or activity of butyrate-producing microbes in the intestine.
Other bacterial signaling molecules that affect motility are just being discovered or have yet to be fully defined. Early studies revealed that infusion of bile acids, many of which are deconjugated by gut bacteria, into the human distal intestine stimulates local motility [82]. The G-protein-coupled bile acid receptor TGR5 is now thought to be an essential part of this bile acid motor reflex response [83]. An undefined product of the probiotic E. coli Nissle 1917 has myoelectric effects in human colonic strips [84], and membrane vesicles from L. rhamnosus JB-1 influence peristalsis in mouse colon via interactions with epithelial cells [85]. Further work is needed to define specific bacterial products and their site-specific mechanisms of action in the human intestine.
A fascinating link has emerged between commensal bacteria and intestinal motility via metabolism of serotonin, one of the key mediators of propulsive transit. Conventionally reared mice have threefold increased quantities of plasma serotonin compared to germfree animals [86, 87]. Germfree mice have reduced colonic expression of tryptophan hydroxylase 1 (Tph1), the rate-limiting gene in serotonin synthesis, and increased expression of the serotonin reuptake transporter [87]. Recent studies in germfree and gnotobiotic mice confirmed that gut microbes stimulate enterochromaffin cells to increase Tph1 expression, raise intestinal and plasma serotonin levels, and increase GI transit rates [88, 89]. These studies also used culture models of enterochromaffin cells to implicate a number of microbial-derived molecules, including SCFAs, secondary bile acids, and intermediates of vitamin synthesis, as stimulating serotonin production [88, 89]. Some of these secreted signals may cross the blood brain barrier, given that germfree mice also have altered hippocampal levels of serotonin metabolites [90]. Butyrate, in particular, increases quantities of serotonin through an inducible zinc finger transcription factor that binds directly to Tph1; mice lacking this gene known as ZBP-89 had lower intestinal and plasma levels of serotonin and were more susceptible to infection [91]. Notably, the presence of gut bacteria does not affect a second source of serotonin, Tph2 in enteric neurons. Studies of Tph2-deficient mice reveal this enzyme is the more important isoform in terms of myenteric plexus architecture and constitutive intestinal transit [92]. Whether therapeutic remodeling of the microbiome to influence serotonin metabolism may have roles in FGIDs remains uncertain.
Host Factors
Complicating matters further is the fact that the host’s motility itself influences the microbiome. In animal models, disruption of regular motor patterns produces SBBO [17–19]. In addition, analysis of stool obtained from healthy volunteers with pharmacologically altered GI transit times found a significant positive correlation between transit rate and total bacterial mass [93]. However, while some bacteria flourish during rapid transit, others prefer a static luminal environment. For example, in mice with congenital colorectal aganglionosis modeling Hirschsprung disease, there were both increased proportions of Bacteroidetes and decreased Firmicutes [94].
In addition to altering microbiota composition, host motility also affects microbial function. The controlled environment of an in vitro continuous culture system was used to confirm that flow rate is a key determinant of both composition and function of human fecal microbial communities [95]. In one example, fecal microbes obtained from adult volunteers with pharmacologically increased transit rates correlated with increased substrate fermentation into SCFAs and decreased pH of the culture medium; the opposite was true for microbes harvested from adults with decreased transit rates [96].
Mechanisms governing the associations between intestinal microbial composition and function are even less clear in human disorders of dysmotility, particularly given the coexistence of other host factors intimately linked to GI pathology including physiologic stress. Mouse models reveal that catecholamine release drastically increases certain intestinal microbial populations [97] and may prime the host mucosa to be more permissive to the attachment of enteric pathogens [98]. These and other host-derived factors must be considered in the context of GI diseases associated with altered rates of transit, including but not limited to irritable bowel syndrome (IBS), inflammatory bowel disease, acute gastroenteritis, and delayed gastric emptying.
In addition to physiologic stress, two recent studies convincingly show that intestinal transit time is inextricably linked to both diet and gut bacteria. The first report analyzed germfree mice, conventionally raised mice, and gnotobiotic mice colonized with a simple humanized microbiota. Transit rates in all animals were accelerated either pharmacologically with polyethylene glycol or via the diet with a nonfermentable polysaccharide, cellulose. In contrast, administration of fructooligosaccharide, a fermentable polysaccharide, decreased SCFA production and slowed transit rates only in mice with microbes. Similarly, germfree mice receiving fructooligosaccharide exhibited more rapid transit similar to that following administration of polyethylene glycol. Likewise, decreasing transit rates with a polysaccharide deficient diet was successful only in mice with intestinal bacteria. These experiments indicate that diet influences motility through both microbiota-dependent and microbiota-independent pathways (Fig. 5.3) [99]. In the second study [100], six groups of germfree mice were humanized by fecal microbes from six different environments: a twin pair from the United States discordant for obesity, a lean US consumer of a protein- and fat-rich primal diet, a Venezuelan living in the rural Amazon, a Bangladeshi living in an urban slum, and a Malawian from a rural village. These groups of mice were in turn fed a succession of different diets with carbohydrate, protein, and fat contents representative of each of the 6 donor types. These experiments found diet-dependent correlations between specific bacterial groups and whole-intestinal transit time. Fecal metabolomic analyses revealed deconjugated bile acids, which are metabolized from conjugated bile acids by bacterial bile salt hydrolases, to be associated with faster transit. Metatranscriptomic analyses revealed that a component of the Bangladeshi diet, turmeric, influenced transit times in a manner that was dependent on the amount of bile salt hydrolase activity present in the host [100]. These experiments illustrate not only the complexity of host-microbiome-diet interactions but also the utility of employing top-down, systems-based approaches to complex mechanism discovery.


Fig. 5.3
Interactions between diet, intestinal microbiome, and GI transit rates (From Kashyap PC, Marcobal A, Ursell LK, Larauche M, Duboc H, Earle KA, Sonnenburg ED, Ferreyra JA, Higginbottom SK, Million M, Tache Y, Pasricha PJ, Knight R, Farrugia G, Sonnenburg JL. Complex interactions among diet, gastrointestinal transit, and gut microbiota in humanized mice. Gastroenterology. 2013;144(5):967–77, with permission)
Mechanisms of Cross Talk Between Microbiota and the Central Nervous System
In comparison to those governing motility, the mechanisms by which gut microbiota communicate with the CNS to influence processes such as pain perception and behavior are less well defined. Much of the current mechanistic knowledge has been obtained using a diverse array of labor-intensive animal model techniques. Neuronal function may be assessed using in situ gene expression or ex vivo patch-clamp action potential recording devices; visceral sensitivity can be assessed by measuring abdominal wall contractions or heart rate during colorectal or gastric distention via intraluminal balloon; and anxiety phenotypes can be replicated by assessing freezing behaviors or other responses to water-avoidant stress, open-field novelty tests, marble burying, and maternal separation of pups. These and other techniques have uncovered novel mechanisms of brain-gut-microbiota interactions in three main categories: modified signaling pathways in enteric nerves and epithelial cells which affect the CNS, CNS structural or functional changes, and induction of systemic responses that may influence host neurobiology.
Gut bacteria may have direct effects on sensory neurons. Infection of mice with Campylobacter jejuni increased the expression of the neuronal activation marker c-Fos both in vagal sensory ganglia and in the nucleus of the solitary tract, a region of the brain where vagal sensory inputs converge [101]. Likewise, Citrobacter rodentium enhanced c-Fos expression in vagal sensory ganglia, while eliciting anxiety-like behavior patterns on open-field testing [102]. Sensory function also was altered in a postinfectious hypersensitivity model. Afferent neurons from mice infected with Trichinella spiralis demonstrated a biphasic response to ex vivo stimulation, showing hyposensitivity during the acute infection and then increased basal activity with hypersensitivity several weeks later. This finding appears to be partially mediated by altered serotonin metabolism and has potential relevance to postinfectious IBS [103]. Although most of the signals mediating communication of sensory information between pathogens and enteric nerves are unknown, one study revealed, as measured by increased excitability and expression of proinflammatory markers, that either lysates from E. coli cell walls or LPS activates mouse colonic nociceptive dorsal root ganglion neurons [104]. Further work remains to identify the specific bacterial secreted products that influence nociception and the ENS sensory pathways that these products activate.
Early studies with probiotics revealed that Lactobacillus farciminis minimizes the effect of partial-restraint stress on visceral hypersensitivity in mice, perhaps by inhibiting colonic mucosal expression of epithelial cell cytoskeletal contractile element phosphorylated myosin light chain [105]. Similarly, the probiotic Lactobacillus reuteri decreases dorsal root ganglion nerve firing and blunts the pain response to colorectal distention in rats [106]; this may occur by inhibiting a calcium-dependent potassium channel on neurons of the myenteric plexus thus affecting both sensory and motor reflex pathways [107, 108]. Another mechanism by which probiotics may reduce nociceptive neurotransmission was elegantly illustrated in a classic study by Rousseaux et al. [109] where Lactobacillus acidophilus induced upregulation of μ-opioid and cannabinoid receptor 2, mediators of nociceptive signal transmission. This occurred both in enterocyte cultures and in murine models, leading to increased rat colorectal distention pain thresholds [109]. Decreased enteric neuronal excitability also has been demonstrated in mice treated with the probiotic Bifidobacterium longum [110, 111].
It is important to note that neuron-modulating effects may not be restricted to specific pathogens and probiotics. Normal activity by intrinsic primary afferent neurons depends on the presence of intestinal microbes in general. Neurons harvested from germfree mice demonstrated lower resting membrane potentials, decreased excitability [112], and decreased responsiveness to agonist-induced firing [113].
Recent data indicate that CNS structure and function is influenced by the intestinal microbiota. Metabolomic analyses reveal that nearly 20 % of mouse cerebral metabolites are altered by the germfree state [114]. In a landmark study, Diaz Heijtz and colleagues [115] reported that germfree mice and gnotobiotic mice colonized by microbes as adults exhibit different behavior including both increased locomotor and decreased anxiety-like behavior. That gnotobiotic mice colonized in adulthood behaved like germfree mice suggests a critical early-life window of brain development mediated by a normal microbiota. The authors then used metabolite and gene expression analyses to correlate the altered behavior phenotype with processes influencing the development of neuronal circuits that mediate locomotor and anxiety behaviors [115]. Conventionally reared mice with antibiotic-depleted microbiota demonstrated similar behavioral changes, correlating with increased hippocampal expression of brain-derived neurotrophic factor, a key mediator of memory, learning, anxiety, and depression [116]. A different study linked behavior changes in germfree mice to altered CNS expression of not only brain-derived neurotrophic factor but also N-methyl-D-aspartate receptor subunit NR2B and serotonin receptor 1A [117]. Other mediators of CNS effects have yet to be identified. For example, Acinetobacter lwoffii, an organism that blooms during antibiotic treatment of rats with chemically induced fulminant hepatic failure, produces an uncharacterized, inactive plasma compound that appears to be converted in the brain to a benzodiazepine receptor ligand that worsens hepatic encephalopathy [118]. Behavioral and gene expression or metabolite changes in the brain also have been reported in conventionally reared mice infected with Trichuris muris [119] or C. rodentium [120] or in animals receiving either the probiotic L. rhamnosus [121] or Bifidobacterium infantis [122, 123] by unknown mechanisms. Whether these reported CNS effects in conventional mice are attributed to direct effects of these individual bacteria or to their secondary alterations to the resident microbiome is unclear.
Recent data from studies in humans support some of the observations made in these mouse models. Administration of Lactobacillus helveticus R0052 and Bifidobacterium longum R0175 reduced psychological distress and anxiety in healthy women in a double-blind trial [124]. In another double-blind trial in healthy women, consumption of a fermented drink containing Bifidobacterium, Streptococcus, and two strains of Lactobacillus altered brain activity as measured by functional magnetic resonance imaging (fMRI) [125]. Changes in fMRI were also seen in patients with cirrhosis and encephalopathy treated with the antibiotic rifaximin [126].
Gut microbes have been shown to alter systemic stress response and inflammatory pathways. Germfree mice have exaggerated ACTH and corticosterone responses to partial-restraint stress. This effect is both blunted by monocolonization with the probiotic B. infantis and exacerbated by monocolonization with enteropathogenic E. coli. Similar to the developmental window revealed for the influence of gut microbes on locomotor and anxiety-like behavior, the exaggerated stress response was ameliorated by early but not late colonization of germfree animals [127]. Bacteria can alter the body’s response to stress through inflammatory pathways. Sun et al. found that exposing mice to 10 days of water-avoidant stress caused several inflammatory- related changes including increased corticotropin-releasing hormone, inhibition of the NLRP6 inflammasome, intestinal inflammation, and microbial dysbiosis. The dysbiosis was characterized by decreased Bacteroidetes, increased Firmicutes, and increased γ-Proteobacteria. Intriguingly, healthy mice acquired dysbiosis, increased levels of corticotropin-releasing hormone, and decreased NLRP6 when co-housed with mice naïve to stress. Each of these effects was ameliorated by giving the co-housed mice either broad-spectrum antibiotics or a mixture of 3 lactic acid-producing probiotics [128]. These data suggest that a portion of the physiologic effects of psychological stress may be driven by various microbial populations.
In summary, gut bacteria exert a myriad of effects on genes, metabolites, and physiologic processes governing multiple neurogastroenterological phenomena. As we continue to develop technology pipelines that enrich our understanding of both known and novel intestinal microbes along with their myriad of secreted products, we will continue to discover new mechanisms of communication between the microbiota and mammalian CNS and new preclinical and clinical tools for maintaining the microbiome in a state of relative health [129] (Fig. 5.4).


Fig. 5.4
Potential pipeline for development of therapeutics for disorders of neurogastroenterology (From Gilbert JA, Krajmalnik-Brown R, Porazinska DL, Weiss SJ, Knight R. Toward effective probiotics for autism and other neurodevelopmental disorders. Cell. 2013;155(7):1446–8, with permission)
Irritable Bowel Syndrome and Related Functional Disorders
Altered gut microbiota populations have been found in patients with FGIDs. Balsari and colleagues were the first to show, using culture-dependent techniques , that fecal microbial populations from adults with IBS were different than those from healthy adults. Compared to controls, they found that symptomatic patients had decreased coliforms, lactobacilli, and bifidobacteria [130]. Altered microbial populations in IBS were subsequently reported using more advanced techniques including real-time PCR [131], PCR combined with a phylogenetic microarray [132], PCR with HPLC-based bile acid profiling [133], percent G + C profiling with a 16S rRNA clone library [134], fluorescent in situ hybridization on rectal biopsy samples [135], and ultimately high-throughput 16S rRNA gene sequencing [136, 137].
Only recently have microbial communities in pediatric IBS been defined. Children with IBS diagnosed by Rome III criteria had higher proportions of γ-Proteobacteria, a class containing multiple pathogens such as Haemophilus parainfluenzae. In addition, supervised machine-learning algorithms identified specific taxonomic units that distinguished with 98.5 % accuracy children with constipation-predominant from those with unsubtyped IBS [138]. In another study, children with diarrhea-predominant IBS had increased proportions of the genera Veillonella, Prevotella, Lactobacillus, and Parasporobacterium and decreased proportions of Bifidobacterium and Verrucomicrobium [139]. Given these associated changes in gut microbiota composition, manipulation of the microbiome has the potential to address processes that may contribute to IBS pathogenesis including microbial fermentation, nociceptive pathways, inflammatory signaling pathways, and abnormal intestinal motility. Multiple meta-analyse s (Table 5.1) have evaluated the more than 60 published clinical trials that examine effects of probiotics in patients with IBS. One early review of eight randomized, placebo-controlled trials found that probiotics conferred a small but statistically significant improvement in symptoms vs. placebo [140]. Other meta-analyses evaluating the efficacy of probiotics for adults with IBS found similarly small yet statistically significant effects [141–145]. The largest pooled relative risk for symptom improvement (RR 17.6, 95 % CI 5.1–61) was reported for adults receiving Lactobacillus spp. for IBS, although beneficial effects reported with Lactobacillus for children in this analysis were less striking [146].
Table 5.1
Select systematic reviews of randomized controlled clinical trials that tested microbiota manipulations in the treatment of neurogastroenterological disorders
Indication | Patient population | N | Species/intervention | Result | Ref |
---|---|---|---|---|---|
Irritable bowel syndrome | Children and adults with IBS established by Rome criteria | 1011 | Bifidobacterium spp. Lactobacillus spp. P. freudenreichii VSL#3 | Improved clinical outcomes [RR 1.2, 95 % CI, 1.07–1.4] | Nikfar et al. [140] |
Children and adults with IBS | 1404 | B. subtilis Bifidobacterium spp. E. coli Lactobacillus spp. L. lactis P. freudenreichii S. boulardii Streptococcus spp. VSL#3 | Reduced global IBS symptoms [RR 0.77, 95 % CI 0.62–0.94] Reduced abdominal pain [RR 0.78, 95 % CI 0.69–0.88] | McFarland and Dublin [141] | |
Adults >16 years with IBS | 1650 | B. subtilis Bifidobacterium spp. Lactobacillus spp. L. lactis P. freudenreichii Streptococcus spp. VSL#3 | Lowered risk of persisting symptoms [RR 0.71, 95 % CI 0.57–0.88] Improved IBS score [SMD −0.34, 95 % CI −0.60 to −0.07] | Moayyedi et al. [142] | |
Children and adults with IBS | >1225 | Bifidobacterium spp. Lactobacillus spp. P. freudenreichii Streptococcus spp. VSL#3 | Improved overall symptoms [RR 1.6, 95 % CI 12–22] Improved overall symptoms [SMD 0.23, 95 % CI 0.07–0.38] | Hoveyda et al. [143] | |
Children and adults with IBS | 440 | Lactobacillus spp. | Improved overall symptoms [RR 7.7, 95 % CI 2.3–25] | Tiequn et al. [146] | |
Children and adults with IBS | 1793 | Bifidobacterium spp. E. faecalis E. coli Lactobacillus spp. L. lactis P. freudenreichii S. thermophilus | Improved global IBS symptoms [RR 2.5, 95 % CI 1.1–5.2] Improved abdominal pain score [RR 2.0, 95 % CI 1.1–3.4] | Didari et al. [145] | |
Adults with IBS and IBS-type symptoms | 234 | Metronidazole Neomycin Rifaximin | Improved IBS symptoms [RR 2.0, 95 % CI 1.2–3.4] | Rezaie et al. [154] | |
IBS and CIC | Adults >16 years | 2575 | B. subtilis Bifidobacterium spp. E. faecalis E. coli Lactobacillus spp. L. lactis P. freudenreichii S. boulardii Streptococcus spp. VSL#3 | Lowered risk of persisting IBS symptoms [RR 0.79, 95 % CI 0.70–0.89] Increased mean stools/week in CIC by 1.49 [95 % CI 1.02–1.96] | Ford et al. [144] |
Functional Gastrointestinal Disorders | Children and adolescents with FGIDs established by Rome III criteria | 741 | Bifidobacterium spp. Lactobacillus spp. VSL#3 | Improved outcomes in abdominal pain-related FGIDs [RR 1.50, 95 % CI 1.22–1.84] No significant improvement for defecation-related FGIDs | Korterink et al. [147] |
Abdominal pain-related FGIDs | Children up to 18 years with FGIDs established by Rome II criteria | 290 | L. rhamnosus | Higher rate of treatment responders [RR 1.31, 95 % CI 1.08–1.59] | Horvath et al. [148] |
To date, two meta-analyses have evaluated probiotics for functional abdominal disorders exclusively in children. In a review of nine trials enrolling 741 children, different probiotic strains significantly improved functional abdominal pain symptoms , although no significant improvement of defecation-related symptoms was reported [147]. Another meta-analysis reviewed three placebo-controlled trials of L. rhamnosus GG enrolling 290 children with abdominal pain-related FGIDs by Rome II criteria. This analysis reported a modest but significant benefit for L. rhamnosus GG, due primarily to a benefit for children with IBS [148]. These meta-analyses are complicated by multiple factors, including heterogeneity of the FGIDs and the use of different microbial species, strains, combinations of agents, doses, and durations of therapy. Combining data from such a heterogeneous group of studies limits the information gleaned from specific interventions. As such, any conclusions based on a small number of studies of varying quality must be interpreted with caution.
Although antibiotic use for nongastroenterological indications may increase the risk of developing functional bowel symptoms [149, 150], there is also evidence that manipulating the microbiota through antibiotic therapy may be beneficial in IBS. Pimentel and colleagues studied a subset (78 %) of adults with IBS meeting Rome I criteria who had a positive lactulose hydrogen breath test suggestive of SBBO. These subjects were treated with a 10-day course of oral antibiotics after which they returned for repeat breath testing and symptom assessment. Patients with a negative breath test at follow-up reported significant improvements in diarrhea and abdominal pain; furthermore, half of the follow-up breath test-negative patients no longer met criteria for IBS [151]. Subsequent double-blind placebo-controlled trials enrolling adults with Rome criteria FGIDs found significant but modest benefits with the nonabsorbable antibiotic rifaximin [152, 153]. The only meta-analysis that has evaluated the effectiveness of antibiotics for IBS found a small but statistically significant benefit. However, the authors warned there is overall insufficient evidence to recommend the routine use of antibiotics in this setting [154]. In children with IBS, a double-blind placebo-controlled trial did not find rifaximin to be superior to placebo [155].
Dietary interventions targeting the intestinal microbiota show promise in treating FGIDs. Ingestion of a fermentable prebiotic may stimulate the growth or activity of a beneficial group of commensal bacteria [5]. The most commonly studied prebiotics are fructooligosaccharides, a fermentation substrate for multiple genera including Bifidobacterium in the production of SCFAs. Fructooligosaccharides demonstrated beneficial effects in randomized placebo-controlled trials of adults with IBS [156] and minor FGIDs [157]. Likewise, a prebiotic mixture containing galactooligosaccharides increased fecal bifidobacteria counts and improved symptoms in adults with Rome II IBS [158]. However, the paucity of published studies precludes evidence-based recommendations regarding prebiotics for FGIDs [144]. Fiber supplementation , which alters colonic microbiome composition and function [159], has been used as a therapy in adults and children with IBS. A meta-analysis [160] which included two fiber supplementation studies in children with functional abdominal pain [161, 162] did not demonstrate significant efficacy. However, the quality of these studies limits their interpretation. Recently, psyllium fiber supplementation was found to decrease abdominal pain frequency in children with IBS [163]. A meta-analysis of fiber supplementation in adults with constipation-predominant IBS (including 3 studies) found efficacy [164]. A clear correlation of dietary fiber supplementation with changes in gut microbiome composition correlating with IBS symptom improvement has not been established [163].
As an alternative strategy, fermentable carbohydrates , which are associated with increased intraluminal gas production and osmotic activity [165, 166], may be restricted in the diet. An open-label low carbohydrate diet improved symptoms in adults with diarrhea-predominant IBS [167]. Several studies have evaluated the role of a low fermentable oligosaccharide, disaccharide, monosaccharide, and polyol (FODMAP) diet in reducing symptoms in adults and children with IBS. One randomized, double-blind, quadruple-arm, placebo-controlled rechallenge trial found significantly higher symptom severity scores in adults with IBS given fructose, fructans, or a combination of the two versus glucose (placebo) [168]. Another randomized, double-blind, crossover trial found amelioration of GI symptoms (including abdominal pain and bloating) in adults with IBS while on the low FODMAP diet [169]. In children with Rome III IBS, both an open-label pilot study and a randomized, double-blind, crossover study found that a low FODMAP diet ameliorated abdominal pain frequency [170, 171]. In addition to ameliorating GI symptoms in those with IBS, low fermentable substrate diets have been found to alter gut microbiome composition and function (decreased fermentation). A low carbohydrate diet decreased gas (hydrogen and methane) production in adults with IBS [165]. Halmos et al. found that when compared to a typical Australian diet, a low FODMAP diet was associated with higher fecal pH, greater microbial diversity, and reduced total bacterial abundance [172]. The authors also found decreased hydrogen production while on the low FODMAP diet [172]. Staudacher et al. found that in comparison to a habitual diet, a 4-week low FODMAP diet lowered both concentrations and proportions of bifidobacteria [173]. In children with IBS, Chumpitazi et al. also found decreased hydrogen production while on the low FODMAP diet [171].
Given that commensal microbes utilize a wide variety of organic substrates for fermentation, a deeper understanding of how the metabolic machinery encoded within the intestinal microbiome affects functional abdominal symptoms may facilitate the discovery of novel prebiotics that have predictable effects on intestinal physiology. In this respect, baseline gut microbiome composition may play a role in determining whether IBS symptoms are ameliorated during a low FODMAP diet . In children with Rome III IBS, those who markedly improved on a low FODMAP diet had a different gut microbiome composition at baseline (prior to any dietary intervention) in comparison to those who did not. These responders were enriched in taxa with known greater saccharolytic metabolic capacity (e.g., Bacteroides, Ruminococcaceae, and Faecalibacter prausnitzii) [171]. Further studies are needed to determine whether one’s gut microbiome may predict dietary intervention efficacy.
Prospectus
At present we have only begun to understand the numerous and complex ways in which intestinal microbes impact human neurogastroenterology. As next-generation sequencing and related technologies, including statistical methods for drawing meaning from these enormous and complex data sets, continue to evolve at a rapid pace, these new tools will further add to our knowledge base. For example, whole metagenome sequencing ushered in a paradigm shift, as we begin to understand intestinal microbial communities in terms of their function rather than simply their composition. Improved approaches to sequencing the intestinal pool of microbial mRNA, or metatranscriptomics, will also shed light on microbiome function. Another important avenue of research will be to explore the contributions of nonbacterial members of the microbiota, including the virome (both eukaryotic viruses and phages) and the mycobiome. As metabolomics approaches become more readily available, the measurement of microbial metabolites in multiple body compartments will lend further insight into beneficial or harmful functions conferred by specific microbes and microbial populations. Another key challenge is to understand the structure and function of the mucosal microbiota; these microbial communities are much more difficult to access and study from patients and, particularly, healthy controls, compared to luminal microbes found in feces.
Understanding how microbes influence neurogastroenterological processes will be essential to determining whether altered microbial communities result from disease states (e.g., dysmotility or inflammation) or whether altered populations actually contribute to pathology of FGIDs. The ultimate goal is to facilitate more rational selection of probiotic strains, combinations of strains, and prebiotics for therapeutic trials based on mechanistic principles. Given the multitude of effects conferred by gut microbes on host neurobiology, it is possible that in the future gastroenterologists will be able to identify unhealthy components of the gut microbiome of patients with IBS and other FGIDs and through strategic manipulations may replace pathologic microbial functions with those that promote health.
References
1.
Hooper LV, Midtvedt T, Gordon JI. How host-microbial interactions shape the nutrient environment of the mammalian intestine. Annu Rev Nutr. 2002;22:283–307. doi:10.1146/annurev.nutr.22.011602.092259.PubMed
2.
Backhed F, Fraser CM, Ringel Y, Sanders ME, Sartor RB, Sherman PM, Versalovic J, Young V, Finlay BB. Defining a healthy human gut microbiome: current concepts, future directions, and clinical applications. Cell Host Microbe. 2012;12(5):611–22. doi:10.1016/j.chom.2012.10.012.PubMed
3.
Hansen CH, Nielsen DS, Kverka M, Zakostelska Z, Klimesova K, Hudcovic T, Tlaskalova-Hogenova H, Hansen AK. Patterns of early gut colonization shape future immune responses of the host. PLoS One. 2012;7(3), e34043. doi:10.1371/journal.pone.0034043.PubMedPubMedCentral
4.
Food and Agricultural Organization of the United Nations (FAO)/World Health Organization (WHO). Health and nutritional properties of probiotics in food including powder milk with live lactic acid bacteria. Report of a Joint FAO/WHO Expert Consultation on Evaluation of Health and Nutritional Properties of Probiotics in Food Including Powder Milk with Live Lactic Acid Bacteria. Basel, Switzerland; 2001.
5.
Gibson GR, Roberfroid MB. Dietary modulation of the human colonic microbiota: introducing the concept of prebiotics. J Nutr. 1995;125(6):1401–12.PubMed
6.
Montalban-Arques A, De Schryver P, Bossier P, Gorkiewicz G, Mulero V, Gatlin 3rd DM, Galindo-Villegas J. Selective manipulation of the gut microbiota improves immune status in vertebrates. Front Immunol. 2015;6:512. doi:10.3389/fimmu.2015.00512.PubMedPubMedCentral
7.
Kelly JR, Kennedy PJ, Cryan JF, Dinan TG, Clarke G, Hyland NP. Breaking down the barriers: the gut microbiome, intestinal permeability and stress-related psychiatric disorders. Front Cell Neurosci. 2015;9:392. doi:10.3389/fncel.2015.00392.PubMedPubMedCentral
8.
Wostmann B, Bruckner-Kardoss E. Development of cecal distention in germ-free baby rats. Am J Physiol. 1959;197:1345–6.PubMed
9.
Skelly BJ, Trexler PC, Tanami J. Effect of a Clostridium species upon cecal size of gnotobiotic mice. Proc Soc Exp Biol Med. 1962;100:455–8.PubMed
10.
Savage DC, Dubos R. Alterations in the mouse cecum and its flora produced by antibacterial drugs. J Exp Med. 1968;128(1):97–110.PubMedPubMedCentral
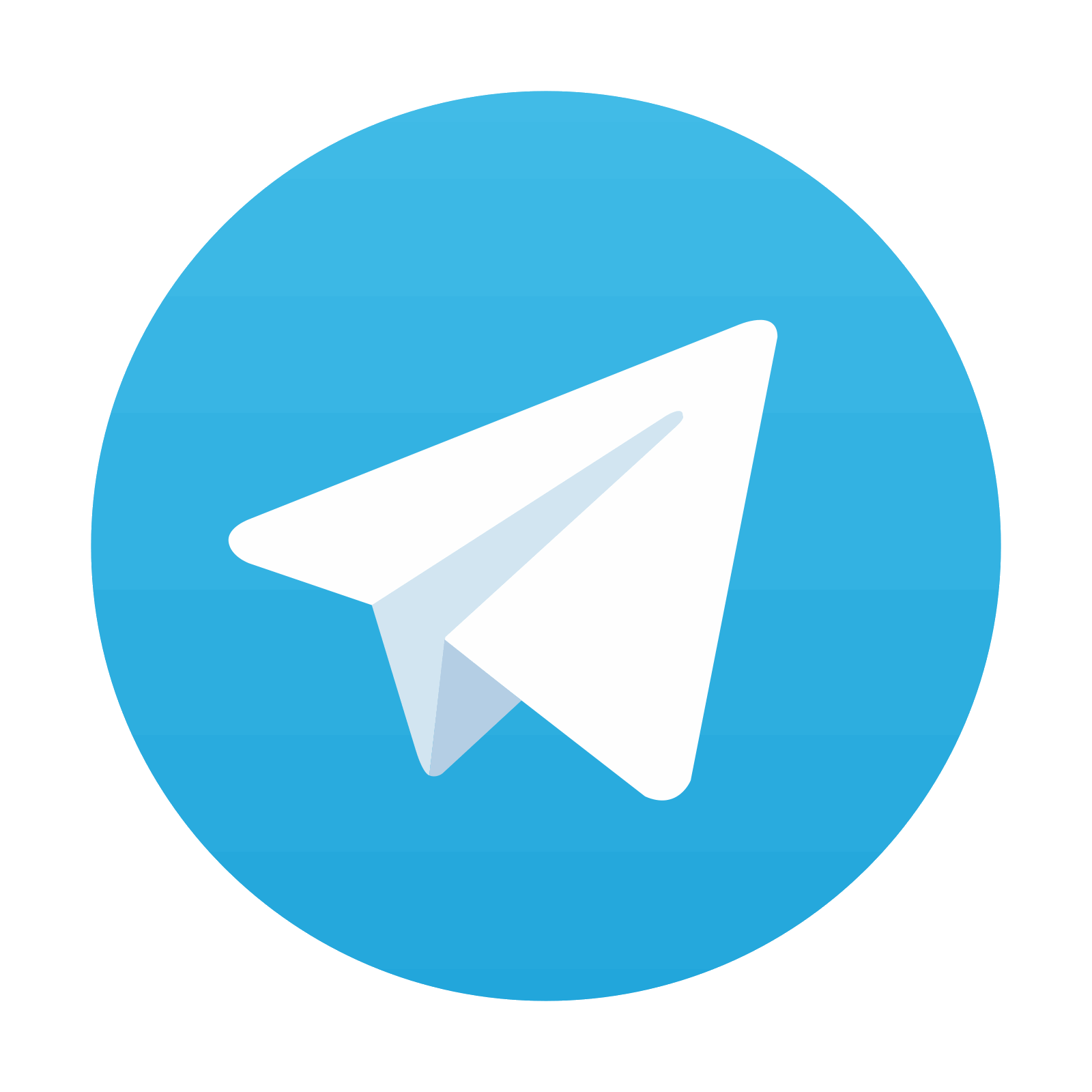
Stay updated, free articles. Join our Telegram channel
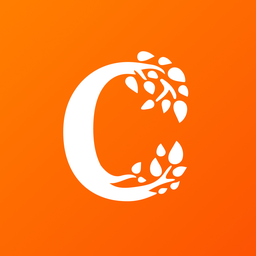
Full access? Get Clinical Tree
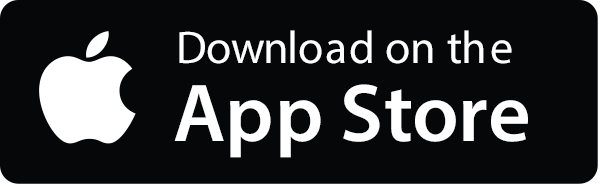
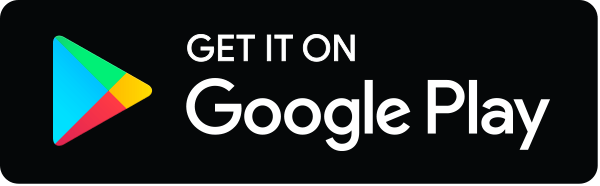