Introduction
Solid organ transplant requires the removal of an organ from one individual, the donor, and its placement in the recipient. Whether the donor is living or deceased, this process inevitably requires a temporary cessation of circulation and hence oxygenation, with attendant cellular dysfunction and damage. Thus when the blood supply is restored to the allograft in the recipient, and the recipient’s immune system can access the transplant, there are broadly two main stimuli that may be recognized: damage-associated signals that activate the innate immune system, and differences in cell surface molecules (such as human leukocyte antigens [HLA] or blood group antigens) between donor and recipient that can activate the adaptive immune system. In the past 50 years, the increased understanding of cellular adaptive immunity has transformed our ability to suppress this arm of the immune system, such that T cell-mediated rejection (TCMR) is now uncommon, occurring in less than 20% of kidney transplant recipients, for example. However, the control of innate and humoral adaptive immunity remains challenging, and efforts to achieve this will need to be underpinned by a greater understanding of the basic biology of these important systems. Of note, attempts to understand the immune response to an allograft have historically relied on rodent and nonhuman primate models. Although useful, such studies do not always accurately reflect the alloimmune response in humans, and there is an increasing emphasis on the need for experimental medicine studies in transplantation to enable advances in genomic, transcriptomic, and proteomic technologies to be harnessed toward this goal. In this chapter, we will provide a description of the various arms of the immune system and consider how they contribute to the immune response to transplanted organs ( Fig. 2.1 ).

Activation of the Immune System Peritransplant
During the process of organ retrieval and reimplantation, there is an inevitable period of ischemia. The cessation of oxygen supply renders the cells unable to generate sufficient energy to continue homeostatic processes that maintain cellular integrity, leading to damage or even death of some cells. This cellular damage or death is associated with the release of molecules that can be detected by both the innate and adaptive immune system. During organ reperfusion, it is the innate immune system that is principally activated. This ancient system includes a soluble arm—the complement system and a variety of opsonins that have evolved to facilitate pathogen recognition, for example, C-reactive protein (CRP), complement activation products (C3b), natural immunoglobulin (Ig)M antibody, and a cellular arm, composed of phagocytes and innate lymphoid cells, including natural killer (NK) cells.
Damage Signals and their Receptors
The innate immune system has evolved to recognize molecules expressed by pathogens, known as pathogen-associated molecular patterns (PAMPs), including specific carbohydrates, lipopolysaccharide (LPS), flagellin, lipoteichoic acid, and double-stranded ribonucleic acid (RNA). This is achieved by an array of receptors, so-called pattern recognition receptors (PRRs), some of which are surface bound and survey the extracellular environment, and some of which are located within the cell, in the cytoplasm or endosomal compartments ( Fig. 2.2 ). PRRs include cell-associated receptors, such as toll-like receptors (TLRs), retinoic acid inducible gene-1–like receptors, and nucleotide-binding oligomerization domain (NOD)-like receptors, and soluble molecules, including CRP, ficolins, and mannan-binding lectin (MBL). Matzinger first proposed that the immune system may have the capacity to respond to damage signals, even in the absence of microbes—the danger hypothesis—and may have even evolved in response to these stimuli. It is now clear that many cell-damage or death-associated signals (termed danger-associated molecular patterns [DAMPs]) are recognized by the same PRRs that mediate responses to PAMPs. These DAMPs include extracellular adenosine triphosphate (ATP), hyaluronan, uric acid, heat-shock proteins (HSPs), and high-mobility group box 1 (HMGB1). These molecules are normally hidden from the immune system or are derived from degradation products of extracellular matrix components generated during ischemia reperfusion injury (IRI) and inflammation. Similarly, falling intracellular potassium and oxidative stress can act as intracellular danger signals.

Soluble Innate Immunity—Complement
The complement system is a series of protein kinases that are sequentially activated and culminate in the formation of the membrane attack complex (MAC). The MAC comprises complement components C5 to C9, which are inserted into the cell membrane (pathogen or host), disrupting integrity and causing cell lysis ( Fig. 2.3 ). In addition, many proximal complement components may augment the immune response to the allograft.

The complement system may be activated by three pathways: the classical pathway, the alternative pathway, and the MBL pathway. IgM or IgG immune complexes activate the classical pathway, and hence this pathway may become activated during antibody-mediated rejection (see section on B Cell Activation). The alternative pathway is constitutively active and must be controlled by a series of regulatory proteins. The mannose-binding pathway is activated by carbohydrates present on pathogens or by damaged endothelium. The net result of activating any of the three pathways is the formation of a C3 convertase (either C4bC2a or C3bBb), which cleaves C3. The resulting C3b cleaves C5 and activates a final common pathway resulting in MAC formation. Complement activation also leads to the formation of anaphylatoxins (C3a and C5a), which activate neutrophils and mast cells, promoting inflammation. In addition, C3b can opsonize pathogens for uptake by complement receptors CR1 and CR3 on phagocytes and can activate B cells; the latter may promote B cell activation in transplantation.
Because the alternative pathway is continuously activated, effective regulation is critical to prevent inappropriate activation. Regulatory proteins may be circulating or membrane-bound. Circulating inhibitors include C1 esterase inhibitor and factors H and I. Membrane-bound regulatory proteins include membrane cofactor protein (MCP), CD55 (decay accelerating factor [DAF]), and CD59 (protectin). Defects or mutations in complement regulatory proteins can result in severe renal pathology, for example, atypical hemolytic uremic syndrome (HUS), which can recur in the transplanted allograft. The C3 glomerulopathies , including dense deposit disease and type I and III mesangiocapillary glomerulonephritides, are also underpinned by complement mutations. Small case series suggest a recurrence rate of around 60% in the transplanted organ. The C5 inhibitor eculizumab may well have efficacy in both primary and recurrent forms of these diseases.
The endothelial cell damage associated with ischemia-reperfusion injury during transplantation leads to MBL and alternative complement pathway activation. Histologic evidence of complement activation (C3d deposition) is present in animal models and in human kidneys with acute tubular necrosis (ATN). Factor B-deficiency and a factor B-blocking antibody are protective in a murine model of IRI, suggesting alternative pathway involvement. Biopsies in murine and human kidneys with ATN also demonstrate MBL deposition, likely triggered by endogenous ligands expressed by dying cells, and MBL-deficient mice are protected from IRI. Transplantation of a kidney from a C3-deficient mouse into a C3-sufficient recipient results in significant attenuation of IRI, in contrast to the reciprocal transplant, suggesting that local C3 production in the kidney rather than circulating C3 is the major player in IRI. In human kidneys, cold ischemia may alter the methylation state of the C3 promoter, resulting in increased local expression of C3 after reperfusion, which is associated with a diminished graft survival. Silencing of the gene encoding C3 using small interfering RNA (siRNA) has been shown to reduce C3 expression, histologic and biochemical parameters of kidney injury, and mortality in an animal model of IRI. The terminal pathway products C5a and C5b–C9 appear to be critical in mediating cellular injury. A C5-blocking antibody and C5a receptor antagonist have both been shown to abrogate IRI and gene silencing of the C5a receptor also protects mice from IRI. Gene silencing may provide a promising tool in renal transplantation, because siRNA-to-complement components might be applied to the allograft during cold storage, before implantation. Similarly, other strategies to inhibit local complement activation may have utility in limiting allograft IRI. Ongoing clinical trial in renal transplantation to prevent IRI and delayed graft function include the use of C1 esterase inhibitors ( https://clinicaltrials.gov/ct2/show/NCT02134314 ) and the C5 inhibitor eculizumab ( https://clinicaltrials.gov/ct2/show/NCT02145182 ). However, although there was a reduced rate of delayed graft function in a small pediatric trial ( n = 57) using eculizumab in kidney transplantation, there was an unexpectedly high rate of graft loss because of thrombosis in eculizumab-treated subjects, necessitating caution in its future use in this context.
Innate Immunity—Cellular Components
Cellular innate immunity comprises a variety of hematopoietic myeloid and lymphoid cells, often poised within tissues for the rapid nonspecific detection of invading microorganisms and transformed cells. However, innate immunity also encompasses various nonhematopoietic cells, such as the gastrointestinal, respiratory, and urogenital epithelium, which, in addition to forming a physical barrier, also express PRRs and orchestrate local immunity ( Fig. 2.4 ).

Neutrophils
Although often viewed as nonspecific effector cells, granulocytes, such as neutrophils and eosinophils, are likely to play a significant role in transplant pathology through their potent effector functions and rapid recruitment to sites of inflammation during IRI and rejection. It is also increasingly appreciated that there may be tissue-resident populations within a variety of organs.
Neutrophils are the dominant circulating phagocyte in humans, and their recruitment into the graft involves a complex multistep process requiring a series of interactions between the surface of the leukocyte and the endothelial cell or its extracellular matrix. The proteins involved fall into three groups: the selectins, and members of the integrin and Ig superfamilies. Initial interaction and rolling of neutrophils along the endothelium allow the leukocyte to sample the endothelial environment, while maintaining its ability to detach and travel elsewhere. This step is largely controlled by the selectins, although α 4 integrins may also play a role. Endothelial cells express interleukin (IL)-8 and platelet-activating factor, which induces strong neutrophil adhesion. This interaction leads to signaling to the neutrophil, slowing and arresting the rolling process. Shedding of L-selectin by leukocytes allows their detachment and extravasation. The latter stages of leukocyte transmigration are regulated mainly by the β 2 integrins and adhesion proteins of the immunoglobulin superfamily.
The expression of adhesion proteins involved in these interactions is upregulated by proinflammatory cytokines. Ischemic damage alone results in increased expression of several cytokines that upregulate the expression of selectins. Other adhesion proteins, such as intercellular adhesion molecule (ICAM)-1 and vascular cell adhesion molecule (VCAM)-1 of the immunoglobulin superfamily and E-selectin (endothelial-specific selectin), are upregulated by cytokines induced by donor brain death and implantation.
After exit from the vasculature, neutrophil PRR engagement by DAMPs can induce the production of reactive oxygen species, hydrolytic enzymes, and cytokines, with graft neutrophilia linked to alloreactive T cell responses and disease activity in mouse models. Neutrophils can also undergo a form of programmed cell death known as NETosis, whereby activated neutrophils form so-called extracellular traps (NETs). These have been observed in human lung transplant recipients and in mouse models of allograft IRI, although how they contribute to inflammation remains controversial. Perhaps less appreciated is the potential role of neutrophils in the resolution of inflammation in alloimmunity: efferocytosis of apoptotic neutrophils leads to the production of antiinflammatory mediators, such as IL-10, while proresolving factors, including lipoxins and resolvins, are important in wound healing and may suppress ongoing rejection.
Macrophages
Tissue-resident macrophages represent the major innate leukocyte population in most tissues. Through their widespread expression of PRRs, these sentinel cells are specialized in antigen phagocytosis and cytokine production, being key drivers of inflammation in numerous settings. During inflammatory conditions, the macrophage pool is further reinforced by recruited monocytes from the bloodstream, with several macrophage- and monocyte-derived cytokines capable of contributing to tissue damage. For example, tumor necrosis factor (TNF)α can drive cellular necroptosis and the concomitant release of intracellular contents and DAMPs, in addition to augmenting angiogenesis, matrix metalloprotease (MMP) production, immune cell activation, and germinal center formation, all with particular importance in the context of transplantation. Furthermore, via production of IL-1β and IL-8, macrophages play a strategic role in the recruitment of neutrophils to inflamed sites through the induction of adhesion molecules on endothelial cells and direct chemotactic activity, respectively.
Endogenous ligands with the capacity to engage macrophage PRRs are generated during transplantation, either through IRI or as a consequence of ongoing rejection. Detection of DAMPs leads to the association of nucleotide-binding domain leucine-rich repeat containing protein (NLRP)3 with apoptosis-associated speck-like protein (ASC), and recruitment of procaspase-1, forming a complex known as the NLRP3 inflammasome ( Fig. 2.3 ). Inflammasome activation results in the cleavage of procaspase-1 to caspase-1, which subsequently cleaves IL-1β and IL-18 from their precursors. Of note, in vitro data suggests that in macrophages, inflammasome activation is a two-step process. First, macrophages must be “primed” by TLR stimuli, resulting in NFγB-dependent pro-IL-1β production and upregulation of NLRP3 expression. A number of DAMPs are thought to signal via TLRs; for example, HMGB1 activates TLR4. The second signal is provided by DAMP receptors, for example the ATP receptor, P2X7R.
IL-1 plays a pivotal role in initiating and amplifying sterile inflammation, as evidenced by experiments showing that mice deficient in the IL-1 receptor (IL-1R) or in the adaptor protein MyD88 (which is required for IL-1R signaling), demonstrate minimal neutrophilic inflammation after challenge with necrotic cells. IL-1β has multiple actions, including stimulation of nonhematopoietic cells to produce the neutrophil chemoattractants chemokine (C-X-C motif) ligand (CXCL)2 (also known as macrophage inflammatory protein [MIP]-2) and CXCL1 (also known as keratinocyte chemoattractant [KC]). DAMPs may also act directly as chemotactic agents for neutrophils. IL-1β also increases the expression of cell adhesion molecules (e.g., ICAM-1 [CD54]) on endothelial cells. ICAM-1 interacts with integrins (CD11 and CD18) on neutrophils and monocytes to promote endothelial adherence and subsequent entry into tissues.
There is a significant body of evidence that suggests that sterile inflammation contributes to the severity of IRI; neutralization of the DAMP HMGB1 with a monoclonal antibody attenuates renal injury after IRI, whereas recombinant HMGB1 exacerbates it. Some DAMPs stimulate TLRs, and mice deficient in TLR-2 and TLR-4 are protected from IRI with a reduction in neutrophil and macrophage infiltration. Furthermore, NLRP3, ASC, and caspase-1 deficient mice are protected from renal ischemic injury. Pharmacologic inhibition of caspase-1 has been shown to reduce renal IRI and may therefore be a viable therapeutic strategy in transplantation. In rodent models, treatment with monoclonal antibodies directed against ICAM-1, CD11a, or CD11b also protect against IRI. In a human phase I trial, ICAM blockade using a murine antibody BIRR1 was associated with a reduction in delayed graft function in renal transplant recipients. However, a randomized controlled trial of anti-ICAM-1 antibody in renal transplantation failed to demonstrate any significant improvement in delayed graft function. Blockade of another adhesion molecule, P-selectin, also attenuates leukocyte recruitment and IRI in rodent models and in humans.
Innate cells may also drive an adaptive alloimmune response. In TCMR, macrophages may act as antigen-presenting cells (APCs), and the IRI-associated inflammation may induce upregulation of major histocompatibility complex (MHC) class II (MHC-II) on resident cells, augmenting their antigen-presenting functions. In antibody-mediated rejection (ABMR), IgG and complement are deposited in peritubular capillaries, facilitating monocyte, macrophage, and neutrophil activation via their Fcγ receptors (FcγR) and complement receptors. Indeed, the presence of neutrophils within peritubular capillaries is one of the diagnostic features of ABMR, and increased numbers of intraglomerular monocytes and macrophages have been observed in C4d+ ABMR. Macrophages may also contribute to chronic ABMR; early macrophage infiltration is predictive of chronic allograft nephropathy and long-term graft survival.
NK Cells and Innate Lymphoid Cells
In the context of organ transplantation, it is increasingly clear that NK cells play a significant role. NK cells are a distinct class of cytotoxic lymphocyte characterized by the production of perforin, granzymes, and IFNγ that play a role as effector cells, lysing sensitive targets according to the presence or absence of specific target antigens. Two subsets of NK cells exist in humans, CD56 bright cells and CD56 dim cells, with CD56 dim NK cells comprising approximately 90% of blood and spleen NK cells. This subset expresses FcγRIIIA (CD16) and undergoes antibody-dependent cell-mediated cytotoxicity (ADCC), the targeted release of cytotoxic molecules in response to FcγR ligation by IgG-opsonized cells. The relevance of ADCC to organ rejection will be discussed in more detail when discussing mechanisms of ABMR. NK cells also express an array of other activating and inhibitory cell surface receptors that dictate cellular activation depending on the microenvironment encountered by the cell. Although the importance of NK cells in bone marrow transplantation has been long established, their role in solid organ transplantation has taken longer to be recognized. Several laboratories using different experimental models found that grafts survive indefinitely in the presence of demonstrable NK effector activity, although more recently CD28-independent rejection in mouse models of transplantation has been shown to be NK dependent and sensitive to blockade of NKG2D. The activating receptor NKG2D is engaged by MHC class I polypeptide-related sequence (MIC) A (MICA) and MICB, that are induced in allografts during acute and chronic rejection. The binding of these ligands to NKG2D activates NK cells to enhance effector functions, whereas the engagement of killer immunoglobulin-like receptors (KIRs) by KIR ligands such as HLA-C (KIR2DL1 and KIR2DL2) and HLA Bw4 (KIR3DL1) generally inhibit function. Genetic studies of donor and recipient HLA-C type (grouped as C1 and C2 depending on polymorphisms at position 77 and 80 and which seem to exhibit differential NK cell inhibition) suggest that long-term outcomes may be influenced by donor or recipient interaction with KIRs. This has also been observed when KIR HLA mismatches are analyzed in HLA-compatible transplantation.
Inhibitory receptor function underlies the phenomenon of responses to “missing self,” which contributes to tumor immunity, the killing of stem cells, and hybrid resistance in experimental models of transplantation.
Beyond NK cells, another class of innate lymphocyte are the recently described “helper” innate lymphoid cells (ILCs). The subject of intense research in the last decade, ILCs are characterized by their similarity to helper T (Th) cell subsets, with the notable absence of somatically recombined antigen-specific receptors or classical lineage markers. ILCs can subdivided into ILC1s, ILC2s, and ILC3s, which mirror Th1, Th2, and Th17 subsets in terms of transcription factor dependency and effector cytokine profile. The phenotype and dynamics of donor and recipient helper ILCs after transplantation remains poorly understood. However, it is likely that the nature of the transplanted organ dictates the relative contribution of ILCs to transplant phenomena: ILCs may be expected to have significant influence on transplanted mucosal tissues, because they are particularly enriched at these sites. For example, the production of homeostatic IL-22 and amphiregulin by ILC3s and ILC2s, respectively, may limit detrimental tissue destruction and reinforce antimicrobial defense at the mucosal epithelium in the gut and lung. Indeed, ILC3-derived IL-22 production has been implicated in reduced disease progression and intestinal tissue damage in murine models of graft-versus-host disease. Conversely, the transition to ILC1-like phenotypes is associated with increased inflammation and may promote early graft dysfunction. Curiously, the absence of ILC reconstitution in severe combined immunodeficiency (SCID) patients after hematopoietic stem cell (HSC) transplantation, including NK cells, was not associated with any overt susceptibility to disease. Therefore more investigation into the precise nature of ILCs within allografts is needed.
Cells at the Interface between Innate and Adaptive Immunity
Although sufficient for initial protection against most microorganisms and sterile insults, innate immunity plays a crucial role in shaping adaptive immune responses according to the context in which antigen is encountered. Indeed, complex mechanisms have evolved to ensure optimal targeting of different effector mechanisms against viruses, bacteria, fungi, protozoa, and multicellular parasites, while maintaining immunologic tolerance toward innocuous self and foreign antigens.
A critical class of innate immune cell mediating this cross-talk between innate and adaptive immunity is the dendritic cell (DC). DCs pick up antigen within tissues and migrate to local draining lymph nodes for MHC-mediated presentation to antigen-specific T cells and the initiation of adaptive immunity. Furthermore, DCs integrate a variety of secondary cues, such as PRR or cytokine stimulation, to dictate the fate of T cell activation. For example, it is not surprising that mucosal-resident DCs are locally primed for the homeostatic induction of peripheral regulatory T cells (Tregs) via production of TGFβ and retinoic acid, whereas those DCs elicited in the context of infection can skew T cell activation toward inflammatory Th1, Th2, or Th17 subsets, depending on the nature of the pathogen. A similar set of considerations can be applied to monocytes and tissue-resident macrophages. Although less efficient at antigen presentation than DCs, several macrophage-derived cytokines can influence T cell polarization and activation, including IL-1β, IL-23, IL-12, and TNFα. This communication with T and B cells is bidirectional. T cell-derived IFNγ and IL-4 or IL-10 are classically associated with the differentiation of monocytes and macrophages to so-called M1 and M2 phenotypes, respectively. M1 macrophages produce high levels of reactive oxygen species and proinflammatory cytokines and chemokines, whereas M2 macrophages produce high levels of IL-10 and tissue-remodeling factors. However, this remains an oversimplification of the complex macrophage phenotypes in vivo . Similarly, macrophages express high levels of FcγRs, cell surface receptors that bind to the Fc portion of IgG antibodies, and mediated potent cellular responses to opsonized microbes, immune complexes, or deposited IgG.
In recent years, there has been increasing appreciation for the role of certain subsets of granulocytes in the activation of adaptive immunity, particularly with regard to B cell activation and maintenance. Neutrophils have been described to promote antibody production by splenic marginal zone B cells via their production of B cell activating cytokines and costimulatory molecules, such as IL-21 and CD40L, respectively. Furthermore, these cells express FcγRs, with the potential for IgG-mediated feedback. There is also evidence that neutrophils can traffic to lymph nodes (LNs) for presentation of antigen to T cells or licensing DCs for T cell activation by TNFα-mediated maturation. Eosinophils have also been demonstrated to be a major determinant of B cell maintenance within the bone marrow and mucosal tissues via similar mechanisms. Given their residency and recruitment to numerous tissues, it is likely that these cells can influence the induction or progression of alloimmunity.
ILCs are critically dependent on, and influence the activity of, neighboring immune cells, including those of the adaptive immune system. Indeed, seminal work by Sonnenberg and colleagues has demonstrated that ILC3s are capable of MHC class II-mediated antigen presentation and the suppression of antigen-specific T cells within the gut. Similarly, others groups have shown that lung-resident and systemic ILC2s and ILC3s are capable of driving T cell activation in a MHC-II-dependent manner. Furthermore, human splenic ILCs support B cell antibody production through the production of B cell activating molecules, including a proliferation-inducing ligand (APRIL) and B cell activating factor (BAFF), and maintenance of B cell-helper neutrophils.
Stimulation of Adaptive Alloimmunity
The antigen-specific or adaptive immune response to a graft occurs in two main stages. In the afferent arm, donor antigens stimulate recipient lymphocytes, which become activated, proliferate, and differentiate while sending signals for growth and differentiation to a variety of other cell types. In the efferent arm, effector leukocytes migrate into the organ and donor-specific alloantibodies are synthesized, both of which cause tissue damage. To initiate adaptive immunity, the graft must express antigens that are recognized by the recipient as foreign, and these include ABO antigens, HLA, and non-HLA “auto-antigens” that are polymorphic.
ABO Blood Group Antigens
When allocating an organ to a potential recipient the first consideration is to ensure that it is compatible for the ABO blood group antigens. ABO antigens are expressed by most cell types in organ allografts and, were an ABO incompatible transplant to be performed, the presence of naturally occurring anti-A and anti-B antibodies in recipients will likely cause antibody-mediated hyperacute rejection and rapid graft loss. Organs from blood group O donors may be safely given to recipients of any blood groups (“universal donor”) and recipients who are blood group AB may safely receive organs from donors of any blood group (“universal recipient”). In practice recipients of organs from deceased donors receive ABO blood group identical organs to avoid inequity of access to organs, although recipients of kidneys from living donors often receive an ABO compatible but non-ABO identical kidney.
HLA Molecules
Histocompatibility antigens differ between members of the same species and are therefore targets of the immune response in allogeneic transplantation. In all vertebrate species, histocompatibility antigens can be divided into a single, albeit multigenic, MHC and numerous minor histocompatibility (miH) systems. Incompatibility between donor and recipient for either MHC or miH leads to an immune response against the graft, more vigorous for MHC than miH. Indeed rejection of MHC-compatible organ grafts is often delayed, sometimes indefinitely, although in some mouse strain and organ combinations miH differences alone can result in acute rejection similar to that observed across full MHC mismatch. On the other hand, the outcomes of allogeneic stem cell transplantation between HLA-identical siblings can be significantly affected by miH mismatches causing graft-versus-host disease.
Major Histocompatibility Antigens
MHC class I proteins are cell surface glycoproteins composed of two chains—the alpha chain, which is highly polymorphic and encoded by a class I gene, and a nonvariable β 2 -microglobulin chain (molecular weight approximately 12 kD). MHC class I proteins are expressed on most nucleated cells, albeit at variable levels, and they are generally responsible for activating cytotoxic CD8 T cells. MHC class II proteins are encoded entirely within the MHC and are composed of two membrane-anchored glycoproteins, an alpha and a beta chain. MHC class II molecules present peptides and activate CD4-expressing helper T cells. The tissue distribution of MHC class II proteins is far more restricted than that of class I, being expressed constitutively only by B lymphocytes, DCs, and some endothelial cells (particularly in humans). During an immune or inflammatory response, many other cell types may be induced to express MHC class II proteins.
Both MHC class I and MHC class II molecules have the capacity to present peptides but the origin of these peptides differs between the two. In the case of MHC-I, they are largely acquired from the intracellular environment, whereas MHC-II largely present peptides acquired from the extracellular environment. Nevertheless, so called “cross-presentation” between these pathways may occur, particularly in the context of specialized antigen presentation by DCs.
A combination of MHC and peptide forms a compound epitope that is engaged by the antigen-specific T cell receptor (TCR). The peptide-binding groove is usually occupied by many different peptides, derived from self-proteins (often those from the MHC) which, during infection, are replaced by those derived from pathogens. The TCR repertoire is subject to negative thymic selection so that autoreactive cells are purged and positive thymic selection for TCRs that engage with peptides presented by autologous MHC occurs. When a pathogen invades, MHC proteins become loaded with foreign peptides that are engaged by TCR in a self-restricted immune response.
In humans, the HLA class I molecules are HLA-A, -B, and -C; MHC class II molecules are HLA-DR, -DP, and -DQ. Their role in presenting antigenic peptide to the TCR has led to the evolution of a high level of genetic diversity such that there are thousands of variants of both MHC class I and class II genes in the human population. This is likely to have evolved in response to their role as restriction elements in the response to pathogen-derived peptides. Certain cohorts of animals within species that have limited polymorphism at MHC loci have been devastated by infections that are cleared without difficulty in closely related species with polymorphic MHC. This genetic diversity in the MHC loci is an important driver of alloimmune sensitization stimulated by pregnancy, blood transfusion, and prior transplantation. The immune mechanisms involved in these responses are not fundamentally different from those involved with any other antigen. The cellular immune response to alloantigen is, however, fundamentally different at least in magnitude, because MHC molecules bind a diverse range of endogenous peptides, which are therefore normally presented at the cell surface. Allogeneic MHC generate a correspondingly wide range of compound epitopes distinct from the repertoire generated by syngeneic MHC. These are therefore recognized as foreign and engaged by the TCR in the so-called “direct alloimmune response.” The cellular immune response to MHC alloantigens is consequently unique in its diversity and therefore the number of T cells that can be recruited to an immune response. Clinically, we currently assess and attempt to optimally match transplant donors and recipients according to the number of HLA-A, -B, and -DR mismatches, with a minimum of 0 mismatches (0-0-0) and a maximum of 6 mismatches (2-2-2) considered in the algorithm. In general, a greater emphasis is placed on matching at DR loci because of the capacity of MHC-II mismatches to activate CD4 T cells.
Minor Histocompatibility Antigens
Several genes within the class I and class II regions do not encode classical MHC proteins. In addition to those involved in antigen processing, others encode nonclassical MHC proteins that are similar in structure to classical MHC proteins but are nonpolymorphic. These may have antigen-presenting capacity for specialized antigens, such as lipids (e.g., mycolic acid and lipoarabinomannan from Mycobacterium ) or peptides of different sequence but with common characteristics (e.g., with N-formylated amino termini). Others such as HLA-G play a role in immune regulation particularly at the feto-maternal barrier.
The class III region of the MHC is large and contains genes encoding proteins with a wide range of functions including many with roles in the immune system, including TNFα and TNFβ. Polymorphisms that determine the production of such cytokines have also been linked to certain immune responses, including transplant rejection.
Although the highest degree of genetic polymorphism within a species lies within the MHC, many other loci encode proteins with a lower degree of variability. It is clear from genetic studies that these proteins can act as transplantation antigens; they are miH antigens. Their structure and distribution were for many years elusive. Although T cells could recognize and respond to cells from MHC-identical individuals, it was almost impossible to raise antibodies against the antigens involved, making biochemical characterization difficult. The knowledge that T cells recognize small peptides, together with the application of molecular genetic techniques, allowed the characterization of the prototypic miH antigen, the male antigen or H-Y. From such work, it is clear that miH antigens generally represent peptides from low-polymorphic proteins presented in the MHC groove, in the same way as a conventional antigen derived from infectious agents. The so-called H-Y antigen is actually derived from a group of such proteins encoded on the Y chromosome. The first observation explains why it has been difficult to raise antibodies to miH antigens: because the combination of autologous MHC with allogeneic peptide constitutes a relatively poor conformational determinant for antibody binding, despite being an adequate determinant for TCR engagement.
MiH antigens can play a prominent role in rejection in a recipient who is given an MHC-compatible graft but in whom preexisting sensitization to miH antigens exists. This situation can be shown in the rat and mouse and probably explains the occurrence of rejection episodes (which rarely result in graft loss) in renal transplants performed between HLA-identical siblings. Multiple miH differences have been shown to represent an immunogenic stimulus equivalent to that of the MHC in a nonsensitized recipient of a cardiac allograft in the mouse but it is difficult to gather similar data in clinical transplantation. Tissue-specific polymorphic protein antigens have also been described, for example, in mouse skin and rat kidney. An endothelial-monocyte antigenic system has been shown in humans, and it has been suggested that cells sensitized to these antigens can cause graft damage. This area has been reviewed by other authors.
Antigen Presentation
As discussed, donor antigens are presented to T cells in the context of MHC. Activation of CD4 T cells is of particular importance, given their ability to promote both cellular and humoral adaptive responses, and has been demonstrated in a number of experimental transplant systems. CD4 T cell activation requires the presentation of antigen in the context of MHC-II and is carried out by professional APCs, namely DCs, B cells, and some macrophages. DCs in particular, have received great attention in this context.
Dendritic Cells
DCs are present in all organs, including those that are routinely transplanted, and in secondary lymphoid organs. Much of the work on DC function has emerged from murine studies, with more limited data from human tissues, because of the challenge of obtaining fresh clinical samples for in-depth analysis. However, expression of CD11c and MHC-II are useful markers to identify classical DCs (cDC) in human tissues. cDC can be further subdivided into the cDC1 subset that are CD141 (THBD) and XCR1 + , the cDC2 subset that express CD1c + , and a further CD1c/CD141 − subset. All three subsets have recently been isolated in the human kidney using flow cytometric analysis.
After transplantation, these cells migrate out of the transplanted organ, into the bloodstream, and will subsequently encounter the recipient lymphoid system, where they are able to interact with and stimulate the host immune response. Tissue-resident DCs have an immature phenotype, but activation with PAMPs or DAMPs results in their rapid maturation into potently immunogenic APCs with high expression of MHC class I and class II antigens together with a range of costimulatory molecules and cytokines (see section on Direct, Indirect, and Semidirect Antigen Presentation).
DCs with an immature or partly mature phenotype deliver tolerogenic rather than activating signals and play a crucial role in the induction of Tregs, T cell anergy, and deletion. These effects are mediated by secretion of cytokines such as IL-10 and TGFβ, which promote the emergence of Treg cells and through the expression of “negative costimulatory molecules” such as programmed death-ligand (PD-L)1/2, inducible T cell costimulatory (ICOS) ligand, Ig-like transcript (ILT)3/4, and Fas ligand.
Direct, Indirect, and Semidirect Antigen Presentation
Allogeneic MHC on DCs derived from the graft can present a wide range of endogenous peptides derived from donor tissue, both from nonpolymorphic proteins but also from MHC proteins themselves. These combinations of peptide and MHC can be engaged by recipient TCR, in a process termed direct antigen presentation ( Fig. 2.5 ). The T cell response to allogeneic MHC occurs at a remarkably high frequency—in the order of 1 in 100. This relates in part to the wide range of endogenous peptides that occupy the MHC groove, generating an equivalent number of compound epitopes. It is also dependent on the capacity of the TCR to recognize multiple distinct ligands, that is to say polyspecificity is a feature of TCR engagement.

Alloantigens can also be processed and presented conventionally by recipient antigen-presenting cells. This is termed indirect antigen presentation (see Fig. 2.5 ). The indirect pathway accounts for the fact that, in animal models, elimination of passenger leukocytes from the graft does not abolish although it may alleviate rejection. Indeed, skin grafts from class II −/− mice transplanted onto normal mice were rejected in a CD4 + T cell dependent manner. These and other experiments demonstrated the potential role of self MHC class II restricted presentation of exogenous alloantigen to stimulate T cell responses through the indirect pathway.
In clinical transplantation, and in particular in the context of chronic allograft dysfunction, evidence for the role of indirect allorecognition has been presented in various reports using peptides derived from polymorphic regions of MHC antigens or cytoplasmic membrane protein preparations. These studies need careful interpretation, however, because such assays present particular difficulties for reproducibility and standardization in an outbred population.
It has been proposed that the direct allogeneic response dominates acute rejection and indirect allogeneic response allows for ongoing class II restricted responses after the loss of donor DCs. This is almost certainly an oversimplification, because there are significant differences between the expression of MHC molecules on the endothelium of mouse versus human, with long-term tonic expression of MHC-II on human endothelium even in the absence of inflammation. Therefore mouse transplant experiments may not reflect the response in humans. Nevertheless, these models have implicated indirect allorecognition in providing cognate help for B cell alloantibody formation, and are supported by the observation of an increased risk for graft loss associated with nondonor-specific and donor-specific antibody.
In addition to direct and indirect antigen presentation, there is evidence that intact proteins can be exchanged between cells in cell culture systems, that MHC proteins transfer in vivo and that transferred MHC stimulates allogeneic responses in vitro. The importance of this semidirect pathway of antigen presentation to transplant outcomes remains to be clearly established.
T Cell Activation
T cell activation requires not only engagement of the TCR by a peptide:MHC complex (signal 1), but also engagement of cell surface costimulatory molecules present on APC (signal 2) and T cell stimulation by cytokines (signal 3) ( Fig. 2.6 ).

Signal 1—TCR Activation
The T cell receptor comprises an alpha and a beta chain that recognize and bind to peptide:MHC complexes. The TCR has no catalytic activity of its own, but forms a complex with six CD3 subunits (γδɛ 2 ζ 2 ) that contain cytoplasmic immunoreceptor tyrosine-based activation motifs (ITAMs). Lymphocyte-specific protein tyrosine kinase (LCK) phosphorylates these ITAMs after TCR ligation resulting in association with the ζ-chain-associated protein kinase: zeta-chain-associated protein kinase 70 (ZAP70). ZAP70 recruitment results in phosphorylation of the adapter proteins SH2 domain-containing leukocyte protein of 76 kD (SLP76) and linker of activated T cells (LAT). LAT facilitates the formation of multiprotein complexes that drive multiple activation pathways leading to de novo expression of a wide range of genes encoding cytokines and cell surface proteins. These signaling pathways are the targets of a number of immunosuppressive medications, including calcineurin inhibitors, and are described in detail elsewhere.
Signal 2—Costimulation
To generate sustained T cell activation, engagement of surface costimulatory molecules is required in addition to signal 1 (see Fig. 2.6 ). These costimulatory molecules determine and mediate short-term function and long-term fate during priming, expansion, and death of T cells. There are many families of costimulatory molecules including those of the immunoglobulin superfamily (e.g., B7), tumor necrosis factor family (e.g., CD154), G protein-coupled receptors (e.g., C3a and C5a receptors), and lectin receptors (e.g., DC-SIGN). As increasing numbers of molecules have been described it has become evident that these interactions are highly complex, involving paracrine and cell contact dependent mechanisms.
In the absence of costimulatory signals, T cells may become anergic, that is they are unresponsive even if they subsequently receive an adequate second signal. Anergic cells can also inhibit the activation of neighboring T cells.
The molecular basis for the costimulatory or second signal for naive T cell activation was defined as that between CD28 and the B7 family molecule now known as CD80. These costimulatory interactions were not only the first to be described but also the first to be manipulated in the setting of clinical transplantation. The cell surface protein CD28 is now known to be a member of a family of similar proteins. Activation of downstream signaling via CD28 results from ligation with B7 family proteins, CD80 or CD86. These proteins are expressed by APCs such as DCs and engage CD28 during antigen presentation. Signaling through CD28 in the context of TCR ligation results in an increase in glucose metabolism and high levels of cytokine and chemokine expression, particularly IL-2, a cytokine that promotes T cell proliferation and survival.
CD28-deficient mice have impaired immune responses but can reject skin grafts, albeit in a delayed fashion ; this is likely because of other costimulatory proteins that can substitute the action of CD28. Blocking the CD28 pathway in normal animals inhibits the alloimmune response and results in prolonged graft survival or tolerance. The most widely used reagent for this purpose has been cytotoxic T lymphocyte-associated protein 4 (CTLA-4)-Ig. This blocks B7 engagement of both CD28 and CTLA-4, the latter of which could be counterproductive because CTLA-4 acts primarily as a coinhibitory molecule, counterbalancing the effects of CD28. This is evident in the severe phenotype of CTLA-4 −/− mice, in which animals die of lymphoproliferative disorder within a few weeks of birth. Similar in structure to CD28, CTLA-4 inhibits the earliest events in T cell activation. CTLA-4 has a higher affinity for CD80 and CD86 than does CD28 and its engagement with CD80 induces a lattice structure at the cell surface consisting of alternating CTLA-4 and CD80 homodimers. These properties of CTLA-4 may limit the ability of CD80 to interact with and cluster CD28 at the immune synapse, potentially explaining the finding that low levels of CTLA-4 can be effective at inhibiting immune responses. CTLA-4 may also deliver a negative intracellular signal independent of its effect on CD28 that halts cell cycle progression and IL-2 production or affects TCR engagement. The actions of CTLA-4 may be even more complex at a polyclonal level because CTLA-4 engagement may promote Treg function in vivo. A modified CTLA4-Ig fusion protein, belatacept, has undergone robust assessment in two large clinical trials, BENEFIT and BENEFIT-EXT, and these demonstrate that it is as effective as calcineurin-inhibitors (CNIs) in preventing acute TCMR in humans, but avoids CNI-associated nephrotoxicity resulting in better allograft function. Belatacept-treated subjects also had a significantly lower rate of development of de novo donor-specific antibody (DSA), and nonhuman primate studies suggest this may be because of inhibition of B cell-T follicular helper (Tfh) cell interactions.
Since the role of CD28 engagement was defined other members of this family of molecules have been identified; bound by various ligands, they generate effects that can broadly be termed costimulatory ICOS, DNAX accessory molecule 1 (DNAM-1) and cytotoxic and regulatory T-cell molecule (CRTAM) or coinhibitory CTLA-4, programmed cell death protein 1 (PD-1), B- and T-lymphocyte attenuator (BTLA), lymphocyte-activation gene 3 (LAG-3), T cell Ig and mucin-domain containing-3 (TIM-3), T cell immunoreceptor with Ig and ITIM domains (TIGIT), and leukocyte-associated Ig-like receptor 1 (LAIR-1). A second major family of costimulatory molecules on the T cell surface is the TNF superfamily including CD27, CD134 (OX40), and CD137 (4-1BB), which interact with a range of TNF-receptor family members. On the T cell surface the TNF receptor family member CD154 (CD40 ligand, gp39) itself interacts with CD40 on B cells, DCs, and monocytes. Larsen and coworkers showed that blocking this interaction could prolong graft survival in a mouse cardiac transplant model. Even more impressive, however, were data that combined CD28 and CD40 blockade induced permanent survival of allogeneic skin grafts in mice with no long-term deterioration of graft integrity. Tolerance to graft antigens could not be shown in these mice, despite the excellent survival of the transplant itself. In a large animal setting, kidney graft rejection in monkeys can be prevented completely with antibodies to CD154 ; however, its clinical application is limited by thromboembolic events associated with its expression on platelets. Alternative approaches using nondepleting antibodies to CD40 are therefore now being explored.
An understanding that memory T cells are an important barrier to successful engraftment in the presence of immunosuppression and to tolerance induction, combined with the fact that they may be relatively resistant to CD28 inhibition, has directed interest toward identifying molecular targets in T memory cells. The expression of CD2 on T effector memory cells, of and lymphocyte function-associated antigen (LFA)-1 on T memory cells, and even expression of a specific potassium channel on T effector memory cells have been investigated. These approaches require further assessment and are not without potential competing risks.
Signal 3—Cytokines
The consequences of TCR engagement and costimulation are proliferation and differentiation of the T cells into effector phenotypes and the concomitant production of cytokines required to stimulate themselves, and other immune cell types, including CD8 T cells, macrophages, DCs, and B cells. IL-2 is a potent activator and proproliferative cytokine for T cells. Its effects are dependent on binding to its cell surface receptor, which has three subunits, α (CD25), β, and γ. During T cell activation the α subunit becomes associated with the other subunits to form a high affinity receptor. Blockade of the IL-2 receptor by targeting the α-chain profoundly inhibits T cell proliferation. CD25 blockade with basiliximab or daclizumab have proven efficacy as induction agents in renal transplantation.
A number of other cytokines act to polarize CD4 T cells toward different fates, where they promote different types of immune responses. Th1 polarized cells mainly produce IFNγ and drive cell-mediated inflammation and immunoglobulin class switching to IgG antibodies. Th2 cells produce IL-4, IL-5, and IL-13, and are involved in IgE class switching and eosinophil recruitment. Th17 cells produce IL-17 and IL-22 and play an important role in the clearance of extracellular pathogens and in autoimmune pathology.
The role of different cytokines in allograft rejection has been approached using neutralizing antibodies and mice deficient in specific cytokines. However, interpretation of these experiments is complex, because the absence of the cytokines can influence immune system development, there is redundancy in the action of cytokines, and cytokines may have opposing actions depending on the context. This is illustrated by studies of IL-2 −/− or IFNγ −/− mice, which demonstrated that neither were required for rejection. IL-15 can for example substitute many of the actions of IL-2, and IL-15 transcripts are found in grafts placed in IL-2 −/− mice. Subsequent studies demonstrated that whereas neither IL-2 or IFNγ were required for rejection, both were required for tolerance induction. This suggested nonredundant functions for both IL-2 and IFNγ in the function of regulatory T cells (see section on Transplant Tolerance).
T Cell-Mediated Rejection
TCMR, previously known as acute cellular rejection, is the most common type of rejection observed in the current era. TCMR occurs in around 20% of kidney transplant recipients and most frequently occurs in the first 6 months posttransplant (see Fig. 2.1 ). TCMR is characterized by immune cell infiltration into the graft and may involve epithelial cells, for example a tubulitis in kidney transplants, or in more severe cases, an arteritis. This infiltrate is composed of CD8 T cells, CD4 T cells, monocytes, macrophages, and B cells. The sequence of events that culminate in TCMR include the migration of immune cells into the graft, which is dependent on the production of chemokines by graft-resident cells and on the interaction of adhesion molecules on endothelial cell and immune cells. Once in the graft, cellular effector functions are activated causing damage to the transplant, and the cytotoxic functions of CD8 T cells in particular play a key role in this process.
Migration of Activated Cells into the Graft
To enter a site of inflammation, leukocytes must migrate across the vascular endothelium. This migration process is controlled by chemokines, and by cell-cell interactions between the leukocyte and the endothelium. Activated and memory cells bear adhesion proteins, chemokine receptors, and addressins, which allow homing to and migration into peripheral tissues. In transplantation, this activation is thought to predominantly take place in secondary lymphoid organs, because aly mice lacking lymphoid organs have reduced graft rejection as do splenectomized mice deficient in LTα or LTβ (and therefore lacking in lymph nodes and Peyer’s patches).
In small bowel transplantation, recipient-derived leukocytes migrate in large numbers into the mesenteric lymph nodes and Peyer’s patches of the graft. This likely results from normal homing of immune cells to the large volume of lymphoid tissue within the graft. During chronic rejection, lymphoid neogenesis or ectopic accumulations of lymphoid cells may develop within transplants in mouse models and in humans, enabling local activation of immune cells within the organ. The movement of lymphocytes out of secondary lymphoid organs requires sphenogosine-1-phosphate receptor, a G protein‐coupled protein, and this has been targeted using FTY720 (fingolimod). FTY720 acts as a high-affinity agonist for S1P1R, inducing internalization of the receptor. This renders the cells unresponsive to the S1P1, depriving them of an obligatory signal required for egress from lymphoid organs. Lymphocytes are therefore unable to access the peripheral circulation and allograft. Although FTY720 was used in a number of clinical trials in transplantation, its use was associated with macula edema and bradycardia, and therefore it has not entered routine clinical use.
The processes underpinning the movement of lymphocytes into the graft are similar to those described for neutrophils in the section on Activation of the Immune System Peritransplant. Of note, antigen-activated lymphocytes migrate into nonlymphoid tissues and may show tissue-selective homing and preference for sites where they are most likely to reencounter their specific antigen. This process may be facilitated further by cognate recognition by the T cell of MHC class II/peptide complexes on the vascular endothelium. Blocking adhesion molecule interactions has been attempted in experimental and clinical transplantation. In general, cocktails of antibodies blocking multiple adhesion molecules are more potent than single antibodies but the results vary. Indeed, in one study a combination of antibodies blocking both ICAM-1 and LFA-1 led to accelerated rejection of rat cardiac allografts. Antisense oligonucleotides have also been used to prevent the expression of ICAM-1 and were effective in prolonging graft survival in experimental models. Small molecule inhibitors also may effectively interrupt the interactions required for leukocyte adhesion and extravasation, and these reagents may simultaneously be effective in blocking IRI and rejection.
Chemokines play a crucial role in leukocyte trafficking under both normal conditions and in the setting of sterile inflammation and rejection. There are more than 40 different chemokines belonging to two major structural families that are recognized by a range of chemokine receptors expressed on immune cells: CC or β chemokines (e.g., MIP-1α/β, [CCL3/4] regulated on activation, normal T cell expressed and secreted [RANTES; also known as CCL5], and monocyte chemoattractant protein [MCP-1; also known as CCL2]) which attract T cells, monocyte/macrophages, DCs, NK cells, and some polymorphs and CXC or α chemokines (e.g., IL-8 [CXCL8] and IFNγ-inducible protein) which primarily attract neutrophils and T cells.
A number of experimental transplant models have demonstrated the importance of chemokines in mediating immune cell infiltration in IRI and in acute and chronic rejection. For example, CCR1-deficient mice accept MHC class II mismatched grafts without immunosuppression and MHC class I and II mismatched grafts with only low-dose immunosuppression. CXCL10 −/− recipients show normal rejection kinetics of a wildtype graft, but CXCL10 −/− grafts placed into wildtype recipients show prolonged survival. These murine studies have sparked interest in therapeutic targeting of chemokine networks in human transplantation, but as yet, no agents are currently used in clinical practice.
Mechanisms of Cytotoxicity
CD8 cytotoxic T cells damage and destroy target cells by the production of lytic molecules such as perforin and granzyme, and through the induction of Fas-Fas-Ligand-mediated apoptosis ( Fig. 2.6 ). In cell culture systems, MHC-mismatched lymphocytes proliferate and produce cytokines in response to one another in the mixed lymphocyte reaction. This results in the differentiation of CD8 T cells into effectors that lyse target cells bearing the mismatched MHC antigens. There is considerable evidence that CD8 T cells are involved in TCMR. First, they make up a significant proportion of infiltrating leukocytes in rejection allografts, in contrast to the low number observed in grafts of animals treated with cyclosporine to prevent rejection. Second, cloned populations of CD8 T cells are capable of causing the type of tissue damage associated with rejection. Third, graft rejection may be delayed by CD8 T cell depletion.
The importance of the individual effector mechanisms of CD8 T cells in mediating rejection is debated. Mice deficient in perforin are still able to reject skin and organ grafts, even when the grafts are resistant to Fas-mediated and TNFα-mediated killing, but grafts mismatched only at the MHC class I were found to be rejected more slowly in perforin knockout mice.
A number of elegant experiments in animal transplant models demonstrate that both antigen-specific and nonantigen-specific effector mechanisms because of collateral damage of bystander cells may be involved in graft destruction.
Complement and TCMR
Murine models suggest that complement may play a role in acute TCMR. In a fully MHC-mismatched model (C57BL/6 to B10.BR), kidneys from C3-deficient donors survived for long periods without immunosuppressive treatment, in contrast to wildtype C57BL/6 donor grafts that were rejected within 2 weeks. Recipient C3 had only a minor effect in this model. Similarly, in a cardiac allograft model, deficiency of the complement regulator CD55 (DAF) in transplanted hearts resulted in aggressive TCMR. The mechanism by which complement augments TCMR may be a result of the effects of C3a and C5a on antigen-presenting cells or a result of a direct effect on T cells via ligation of C3aR and C5aR.
B Cell Activation and Antibody-Mediated Rejection
B Cell Activation
B cells are immune cells of the lymphoid lineage that develop in the bone marrow ( Fig. 2.7 ). They are characterized by the expression of antibody as their surface antigen receptor, the B cell receptor (BCR), and other markers such as CD20 and CD19. Antibodies comprise two heavy and two light chains, and are classified according to the heavy chain they express; IgM antibodies have a μ heavy chain, IgG antibodies a γ heavy chain, etc. When B cells emerge from bone marrow, they express an IgM antibody and pass through a transitional phase (expressing high levels of CD24 and CD38 on their surface) before becoming follicular B cells that reside within secondary lymphoid organs (the lymph node and spleen).

When B cells encounter an antigen that binds their surface BCR they may either develop into short-lived plasmablasts that produce early, germ-line encoded antibody or alternatively, become a germinal center B cell. Here, they divide and mutate their variable region genes (somatic hypermutation) in an attempt to produce antibody with a higher affinity for antigen. They also undergo class switching, so that the heavy chain present in antibody changes from μ to one of the other isotypes. During many rounds of division and hypermutation, B cells with a high affinity BCR are positively selected and differentiate into either memory B cells or plasma cells. A subset of CD4 T cells, known as Tfh cells, are engaged by germinal center (GC) B cells presenting antigen to them. This Tfh-B cell interaction is essential for the progress of the GC reaction and for the development of memory B cells (that express CD27) and plasma cells. Only a small proportion of plasma cells arising from the GC become established as long-lived plasma cells in the bone marrow. They reside within a number of limited niches, do not proliferate, but act as long-term antibody factories, producing IgG. Plasma cells have also been described in inflamed tissues in autoimmunity and within allografts.
B Cell Function
B lymphocytes are best known for their ability to produce antibodies—the soluble (humoral) mediators of an adaptive immune response. In addition, B cells can act as important antigen-presenting cells for CD4 T cells, thereby initiating cellular immunity, and may produce cytokines that can both activate and regulate a range of other immune cells. B cells also contribute to the development of secondary lymphoid organs (lymph node and spleen), and can therefore have broad effects on immune responses far beyond their most obvious function of antibody generation.
Alloantibodies
Antibodies are the only soluble components of the adaptive immune system and have wider tissue distribution than their cellular immune counterparts. Alloantibodies, particularly those that recognize donor HLA, can mediate hyperacute rejection, acute ABMR, and chronic ABMR. Early efforts to assess whether recipient antibodies could potentially recognize donor cells were based on the cytotoxic crossmatch assay, incubating recipient serum with donor cells in the presence of complement. Currently, HLA genotyping of the donor and recipient and the use of single HLA antigen beads (SAB) allow a more precise assessment of the titer of DSA, assisting in the assessment of pretransplant immunologic risk. Currently, around 30% of patients on the kidney transplant waiting list are sensitized and have varying levels of HLA antibodies that may preclude transplantation or require an antibody-reduction strategy to allow transplantation to proceed. A meta-analysis of published data suggests that even low titers of pretransplant DSA (detectable by SAB but with a negative flow cytometric crossmatch), doubles the risk of ABMR and increases the risk of graft failure by 76%. In addition, the development of de novo DSA posttransplant in nonsensitized transplant recipients is associated with an increased frequency of acute ABMR and worse graft survival. The pathogenicity of HLA DSA also varies according to its specificity, with MHC class II DSA having a worse effect on the allograft than class I DSA. In addition, non-HLA antibodies such as those binding MICA or angiotensin II type 1 receptors may also have a deleterious effect on grafts. Sigdel and colleagues used high-density protein arrays to analyze serum samples obtained from 172 renal transplant recipients and identified 38 de novo non-HLA antibodies that significantly associated with the development of chronic allograft injury on protocol biopsies. Other non-HLA binding antibodies that may negatively affect the allograft include antibodies binding apoptotic cells.
Antigen Presentation to CD4 T Cells
In order for CD4 T cells to orchestrate adaptive immune responses, they must be activated by antigen presented to them in the context of an MHC class II molecule, together with costimulatory signals. B cells are very effective APCs and have several advantages over DCs, traditionally considered to be the principle APC. First, they may have a high-affinity, specific receptor for antigen (the BCR), and can therefore efficiently and rapidly acquire large amounts of antigen for presentation. Second, they can clonally proliferate, and therefore may rapidly become the numerically dominant APC. Murine models have shown the importance of B cells for T cell activation in vivo, including in the context of autoimmunity and alloimmunity. In human kidney transplant recipients, transcriptomic analysis of kidney biopsies with TCMR supports the notion that B cells contribute to worse outcomes in TCMR. Sarwal and colleagues identified a B cell transcriptomic signature (CD20, CD74, immunoglobulin heavy and light chains) in allografts with steroid-resistant TCMR and a poorer outcome. Furthermore, the 11 genes found to comprise a “common rejection module,” present in samples obtained from a variety of organs during rejection, include the chemokines CXCL9 and CXCL10 that are produced by B cells after interactions with cytotoxic T cells. Two B cell genes (CD72 and BTLA) are also among those most differentially expressed in biopsies with TCMR. Together, these data emphasize the potential importance of B cells as APCs in transplantation, and in this context, it is notable that B cell depletion has proven to be an effective treatment for autoimmune diseases considered to be mediated by T cells, including rheumatoid arthritis, multiple sclerosis, and type 1 diabetes mellitus.
Formation and Maintenance of Secondary Lymphoid Tissue
B cells produce lymphotoxins (LTs) and thereby initiate the formation and shape the architecture of lymph nodes and spleen. Their ongoing production of LTα1β2 is also required for the maintenance and integrity of subcapsular sinus macrophages that form a protective screen around the perimeter of lymph nodes. B cell production of VEGF-A may also promote intranodal lymphangiogenesis, increasing antigen and DC distribution in the lymph nodes. They also may be involved in the generation of tertiary lymphoid structures that emerge in inflamed organs affected by autoimmunity, for example in Sjogren disease, rheumatoid arthritis, or type 1 diabetes mellitus, that may be the source of some autoantibodies.
Production of Proinflammatory Cytokines
B cells have the capacity to produce a number of cytokines after stimulation and can thereby affect a variety of immune cells. IL6 is a cytokine required for T-dependent antibody responses, but B cells can themselves produce this cytokine, and this may contribute to autoimmune pathology, for example, via Th17 cell activation leading to exacerbation of experimental autoimmune encephalomyelitis. Indeed, B cells from patients with multiple sclerosis also demonstrate increased IL-6 production compared with healthy controls. B cell production of TNFα and IFNγ can also promote Th1 T cells and macrophage activation.
Recently so-called innate response activator (IRA) B cells have been described. These splenic IgM+ B cells can produce granulocyte macrophage-colony stimulating factor (GM-CSF) in response to LPS, a TLR4 ligand, facilitating the mobilization of neutrophils. This makes an important contribution to pathogen containment in mouse models of gram-negative sepsis and in pleural responses to gram-positive bacteria but may exacerbate atherosclerosis via Th1 cell activation. These B cells are thought to originate from B1 B cells, a subset enriched in the peritoneal and pleural cavities.
B Cells as Regulators of the Immune Response
There is increasing evidence that B cells can not only act as effectors of the immune response but can also play an immunoregulatory role, particularly via the production of IL-10 but also by contact-dependent mechanisms (e.g., via PD-L1 expression ) and perhaps by direct granzyme-B-dependent cytotoxicity.
IL-10-producing B cells have been shown to be important in limiting autoimmunity in mouse models. In mice, several groups have identified IL-10-producing B cells within a number of B cell populations including B1, transitional, and marginal zone subsets. IL-10-producing B cells have also been identified in humans and comprise around 5% of circulating B cells, although cells with the potential to produce IL-10 may be found at higher frequencies. As in mice, human IL-10-producing B cells are present within different subsets, including transitional B cells. Several markers, such as CD5, CD1d, Tim-1, CD9, and CD80, have also been reported to localize to these “regulatory cells.” Unlike regulatory T cells that can be identified by expression of Foxp3, a subset-specific transcription factor has not been identified in regulatory B cells. Some recent data suggest that plasma cells may be the main source of IL-10 in vivo.
The potential importance of B cells with a regulatory capacity has now been identified in a number of disease contexts, including systemic lupus erythematosus (SLE), allergy, transplant tolerance, and protection from allograft rejection. In particular, the balance between the production of IL-10 and proinflammatory cytokines such as IL-6 and TNFα may be important in regulating immune responses. For example, a lower number of CD24/CD38 high transitional B cells that produce high levels of IL-10 versus TNFα is associated with worse allograft outcome in renal transplant recipients. In contrast, in patients with drug-free long-term graft function that are deemed “operationally tolerant” there is a significant increase in the number of total B cells, particularly memory B cells and B cells expressing CD1d and CD5. In vitro, B cells purified from these subjects had a relative increase in FcγRIIB expression and an increase in B cell scaffold protein with ankyrin repeats 1 (BANK1), a negative regulator of CD40 signaling. Other studies show a higher proportion of CD24/CD38 high transitional B cells associated with long-term transplant tolerance and B cells from tolerant subjects produce more IL-10 after in vitro stimulation.
Antibody-Effector Function in ABMR
Acute ABMR is uncommon in nonsensitized transplant recipients and is difficult to treat. The diagnosis of ABMR has been facilitated by more sensitive methods of alloantibody detection and by the identification of complement activation in allograft biopsies via C4d staining. Clinical features of ABMR include a decline in allograft function, the presence of donor-specific HLA antibodies, C4d deposition in peritubular capillaries, and evidence of acute vascular injury (e.g., capillaritis, with neutrophils in capillaries). ABMR, particularly in its chronic form, may occur in the absence of C4d deposition on biopsy. Analysis of transcripts may also assist in making a more accurate assessment of the type of rejection, particularly in diagnosing antibody-mediated rejection, compared with standard histopathological analysis, even in the absence of C4d staining.
A gradual decline in allograft function with time is almost universally observed. This was previously termed chronic allograft nephropathy but the more recent Banff Classifications have sought to distinguish nonimmunologic insults, for example, CNI toxicity, from chronic rejection, particularly chronic ABMR. Chronic ABMR is characterized by vasculopathy and in the kidney is evidenced by glomerulopathy (double contouring in peripheral capillary loops) and peritubular capillary basement. There may also be peritubular capillary C4d staining, although this is not universal. Clinically, this usually occurs in patients with detectable HLA DSA, often in the context of noncompliance.
The histologic features of ABMR result from well-established pathways of alloantibody effector function, namely direct activation of endothelial cells via binding to MHC complement activation (via the classical pathway) or recruitment and activation of immune cells that express receptors for the Fc portion of IgG or complement receptors (including neutrophils, macrophages, monocytes, DCs, and NK cells ; Fig. 2.8 ).

Direct Stimulation of Endothelial MHC
Independently of FcγR engagement, IgG can directly activate allograft endothelium. Binding of DSA to HLA induced intracellular signaling and endothelial cell survival and proliferation, mediated by upregulation of the fibroblast growth factor (FGF) receptor and increased FGF ligand binding. Furthermore, DSA deposition could induce expression of endothelial P-selectin for adherence of monocytes. Reed and colleagues have produced an elegant body of work demonstrating that HLA antibodies can have direct effects on allograft endothelial cells via variable region binding.
Complement Activation
IgG immune complexes can activate complement via the classical pathway and this process is evident in ABMR by the detection of C4d on peritubular capillaries. Further evidence that complement fixation may contribute to the pathogenicity of alloantibodies is provided by the studies investigating C1q or C3d binding. Loupy et al. investigated 1016 antibody-compatible renal transplant recipients and demonstrated that patients that developed C1q-binding DSA after transplantation had the lowest 5-year rate of graft survival (54%), compared with those with noncomplement-DSA (93%). A more recent study suggests that the detection of HLA antibodies that bind to C3d may have an even greater prognostic significance in patients with acute ABMR. A number of complement inhibitors, including eculizumab and C1 esterase inhibitors, are currently being trialed for both the prevention of ABMR in antibody-incompatible transplant recipients, and for the treatment of ABMR.
FcγR Activation
The absence of C4d staining in more than half of biopsies with late ABMR highlights the importance of complement-independent mechanisms in mediating the deleterious effects of DSAs. Furthermore, some IgG isotypes (IgG4) cannot fix complement, whereas IgG2 has a limited complement-activating capacity compared with IgG1 and IgG3. FcγRs bind to the Fc portion of IgG and mediate activation of the immune cells that express them ( Fig. 2.8 ). In humans, there are several activating receptors (FcγRIIA, FcγRIIC, FcγRIIIA, and FcγRIIIB) and a single inhibitory receptor, FcγRIIB, which plays a critical role in suppressing IgG-mediated inflammation. FcγRs are widely expressed on immune cells, including neutrophils, monocytes, macrophages, DCs, mast cells, NK cells, and B cells but the effect of FcγR ligation varies between cells. For example, neutrophils express FcγRIIA and FcγRIIIB, and cross-linking leading to phagocytosis, cytokine and superoxide production, neutrophil adhesion, and extracellular trap formation (NETosis), whereas on macrophages and dendritic cells, FcγR ligation induces proinflammatory cytokine production, including TNFα and IL-1β, phagocytosis, CCR7-dependent migration, and antigen presentation.
There are several lines of evidence implicating FcγR activity in rejection severity and graft survival in ABMR. NK cells, in particular, have received significant attention as an immune cell subset mediating FcγR-dependent inflammation in AMBR. Hirohashi et al. demonstrated that chronic allograft vasculopathy in murine heat allografts was NK cell and FcγR dependent, inflammation being attenuated after anti-NK1.1 IgG-treatment or in the presence of F(ab′) 2 fragments of IgG2a DSA. Consistent with a role in human ABMR, FCGR3A and other NK cell-associated transcripts correlate with the presence of DSA and ABMR. Furthermore, several groups have examined activating FcγR single nucleotide polymorphisms (SNPs) in kidney transplant recipients. A recent study of a cohort of 85 DSA-positive kidney allograft recipients by Arnold et al. demonstrated that individuals with the high-affinity FcγRIIIA-V158 variant showed higher rate of peritubular capillaritis, an effect independent of C1q-binding or capillary C4d. Functionally, a model NK cell line expressing FcγRIIIA-V158 produced over two fold more IFNγ upon incubation with HLA antibody-coated cells compared with those expressing low-affinity FcγRIIIA-F158, consistent with the potential role of NK cells in driving ABMR.
FcγR associations have also been identified independently of NK cells and FcγRIIIA. Whereas allograft survival was increased in patients with the FcγRIIA-R/R131 genotype in one study, in subsequent studies the same genotype was associated with acute rejection. The FcγRIIA-R131 variant exhibits reduced binding affinity for IgG1 and IgG2 subclasses and has been postulated to contribute to pathology through inefficient clearance of deposited IgG within allografts. This is consistent with the high levels FcγRIIA expression on professional phagocytes. Indeed, monocytes expressing the high-affinity FcγRIIA-H/H131 variant were found to adhere more strongly to HLA antibody-activated endothelium. In a larger study of 200 kidney transplant recipients who had lost their grafts, the FcγRIIA-R/R131 genotype was associated with early graft loss, particularly in those patients who were DSA positive.
In mouse models, FcγRIIB-deficient mice develop alloantibody-driven chronic vasculopathy analogous to human chronic rejection in a cardiac allograft model (BM12 organs into C57BL/6 mice). This study is consistent with the known role of FcγRIIB in suppressing humoral immunity, although the exact mechanism of IgG-mediated pathology was not dissected. Indeed, in a model of antibody-mediated nephritis, myeloid-specific FcγRIIB deficiency is sufficient to exacerbate tissue inflammation. A number of nonsynonymous SNPs have been identified in the FCGR2B gene in humans, with one occurring at a notable frequency (rs1050501). This SNP encodes an isoleucine-to-threonine substitution at position 232, located within the transmembrane domain. This substitution results in receptor loss of function as a result on impaired lateral mobility and recruitment to signaling domains. Surprisingly, despite being a major risk factor in SLE and the heightened inflammatory responses of monocytes from FcγRIIB-T232 individuals to IgG, no association was observed between FcγRIIB-T232 and graft or patient survival in a large study of more than 2800 renal transplant recipients. However, patients were not stratified, for example based on DSA or ABMR status, possibly masking effects.
Curiously, human cultured aortic endothelial cells also express FcγRI and FcγRII and mediated IgG internalization, cytokine production, and the upregulation of adhesion molecules directly. This expression can be further enhanced with IFNγ and TNFα in vitro. However, whether this occurs in vivo and whether it contributes to IgG-mediated phenomena in allografts remains to be addressed.
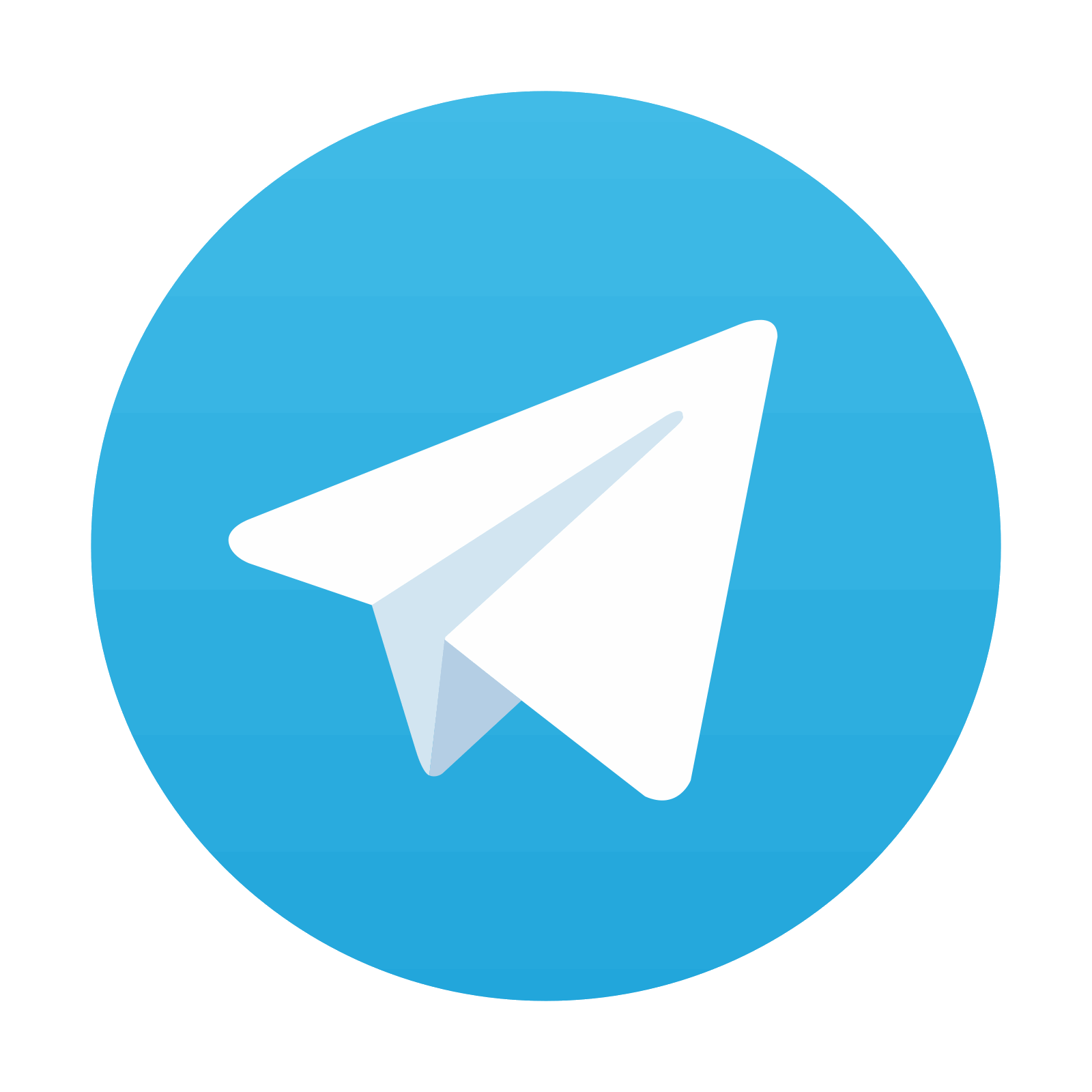
Stay updated, free articles. Join our Telegram channel
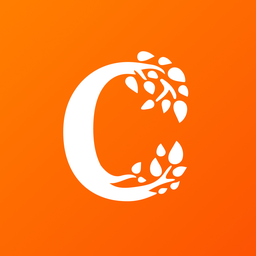
Full access? Get Clinical Tree
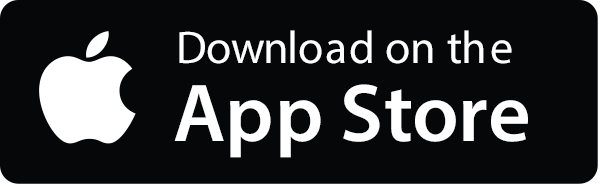
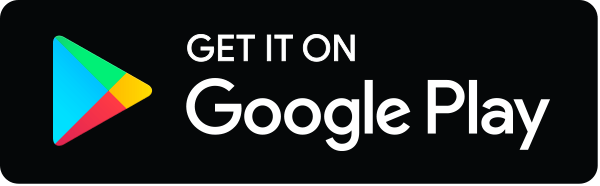