Abstract
The cell cycle is a process in which a cell first duplicates its genetic information and then divides to give rise to two daughter cells. To avoid mis-segregation of the genetic materials and incorporation of errors in the genome, the cell cycle is carefully monitored. This is achieved by positioning throughout the cell cycle a number of checkpoints designed to detect DNA damage, misaligned chromosomes, and incompletely replicated DNA. Checkpoints are mediated by cyclin-dependent kinase inhibitors (CKIs) in interphase and mitosis. On the other hand, progression through the cell cycle requires the combination of cyclins and cyclin-dependent kinases (CDKs), together of which regulate activities of target proteins by phosphorylation. The proper regulation of the cell cycle is critically important for cell proliferation as mutations in genes involved in cell cycle control often lead to cancer. This chapter reviews the components of the cell cycle and its control, checkpoint mechanisms, and provide examples of pathological consequences of dysregulation of the cell cycle machinery. This information is crucial for understanding the mechanisms regulating growth, proliferation, and differentiation of cells in the gastrointestinal tract.
Keywords
Cyclins, Cyclin-dependent kinases (CDKs), CDK inhibitors (CKIs), Checkpoint, Cancer, DNA damage repair, Mitosis, RB, p53, FBXW7, Ubiquitination, Growth factors, Mitogens, Spindle assembly checkpoint (SAC)
Acknowledgment
This work was in part supported by grants from the National Institutes of Health (DK052230, DK093680, CA084197, and CA172113).
8.1
Components of the Cell Cycle
A typical eukaryotic cell cycle contains several distinct phases, which progress in an orderly fashion—a phase cannot commence without completion of the previous one. The four phases of the cell cycle are G 1 (G for gap), S (synthesis), G 2 , and M (mitosis) phases ( Fig. 8.1 ). The G 1 , S, and G 2 phases collectively make up the interphase. The DNA content of a cell in the G 1 phase is 2N (N is the number of chromosomes), also known as diploid, whereas the DNA content of a cell in the G 2 phase is 4N (tetraploid). The DNA content of a cell in the S phase varies between 2N and 4N, depending on the stage of replication. The M phase is in turn comprised of two processes: mitosis, in which the cell’s chromosomes are equally divided between the two daughter cells, and cytokinesis (or cell division), in which the cytoplasm of the cell divides in half to form two distinct daughter cells. Typically, the amount of time required for a single-cell cycle in actively proliferating human cells in culture is 24 hours. Of these, the M phase takes approximately 1 hour to complete and interphase takes up the remaining 23 hours.

In addition to the four phases of the cell cycle listed above, one phase that lies outside the cell cycle is called the G 0 (0 for zero) phase. Cells in this phase are in the resting phase, which is often the result of their leaving the G 1 phase of the cell cycle. Typically, a cell enters G 0 phase if the environment is not conducive for the progression of the cell cycle, as in the event of deprivation of essential nutrients or growth factors, or if a cell has reached a fully differentiated state such as a hepatocytes or neuron. In these conditions, the cell is sometimes referred to as in a quiescent state. Additionally, a cell can enter the G 0 phase and become senescent due to DNA damage or telomere attrition. This is often an alternative to self-destruction of the damaged cell by apoptosis.
In the M phase, mitosis commences when DNA condenses into visible chromosomes, followed by the separation of the chromosomes into two identical sets. Cytokinesis is the last phase of mitosis when the two daughter cells separate, each with a nucleus and cytoplasmic organelles. Mitosis begins with nuclear membrane breakdown followed by condensation of the chromosomes and separation of the centrosomes (prophase). This is accompanied by the formation of mitotic spindles, which are attached on one end, the centrosomes, and the other end, kinetochore, a protein structure located at or near the centromeres of mitotic chromosomes (prometaphase). At this point, kinetochores that are unattached to the mitotic spindles generate a “wait” signal that delays the onset of anaphase until all chromosomes are properly attached and aligned. This signal is also called mitotic checkpoint or spindle assembly checkpoint (SAC), which is satisfied once all chromosomes are congregated at the equatorial plate (metaphase). This is followed by separation of the chromosomes to the opposite poles (anaphase) and formation of new nuclear membranes around the daughter nuclei and uncoiling of the chromosomes (telophase), eventually forming a cleavage furrow that leads to the formation of two daughter cells (cytokinesis). Fig. 8.2 illustrates the various stages of mitosis.

8.2
Control of the Cell Cycle
The organization of the cell cycle and its control system are highly conserved among eukaryotic organisms. Abundant information on how the cell cycle is regulated in vertebrates was derived from earlier studies in yeast. These studies demonstrate that both cell cycle progression and cell division are driven by the sequential activation, and subsequent inactivation, of two key classes of regulatory molecules, cyclins, and cyclin-dependent kinases (CDKs) Cyclins and CDKs form heterodimers with the former acting as the regulatory subunits and the latter catalytic subunits; CDKs are inactive in the absence of their cyclin partners. Specific pairs of cyclin-CDK dimers function in specific phases of the cell cycle ( Fig. 8.3 ). For example, the cyclin E-CDK2 complex becomes active late in the G 1 phase and is responsible for the transition from G 1 into S phase. When activated by a bound cyclin, CDKs phosphorylate a series of downstream target proteins, either activating or inactivating them, which then orchestrate the coordinated entry into the next phase of the cell cycle. The identities of the target proteins depend on the particular combination of cyclin-CDK complexes. While CDKs are constitutively expressed throughout the cell cycle, cyclins are synthesized (and destroyed) in specific stages of the cell cycle (hence the name cyclin), which is often dependent upon various signaling molecules. This cyclic nature of cyclin expression ensures that CDKs are activated and inactivated in a precise manner and safeguards the orderly progression of the cell cycle ( Fig. 8.4 ).


8.2.1
Cyclins
Cyclins were named because they undergo a cycle of synthesis and degradation in each cell cycle. Depending on the timing of their expression and functions in the cell cycle, cyclins are divided into four classes. Three of these classes, the G 1 /S cyclins, S cyclins, and M cyclins are directly involved in the control of cell cycle events. The fourth class, the G 1 cyclins, controls the entry into the cell cycle in response to extracellular growth factors or mitogens. In the G 1 phase, growth factors are necessary to initiate and maintain the proper transition to the S phase. Early in the G 1 phase, growth factors stimulate the synthesis of G 1 cyclins, represented by the cyclin D family of cyclins, which activates CDK4/6 to induce synthesis of downstream targets, one of which is cyclin E. The rise in cyclin E (a G 1 /S cyclin) levels and activity of its partner, CDK2, drive the cell past a restriction point (R in Fig. 8.4 ) in the cell cycle after which the cell is irreversibly committed to proceeding to DNA synthesis, even if the growth factors are withdrawn. Subsequently, the S cyclins (represented by cyclin A) and M cyclins (represented by cyclin B) are required for the initiation of DNA replication and entry into mitosis, respectively ( Fig. 8.4 ).
8.2.1.1
G 1 Cyclins
The G 1 cyclins are composed of the D-type cyclins that include cyclins D1, D2, and D3. Along with their partners, CDK4 and CDK6, G 1 cyclins act early in the G 1 phase of the cell cycle. The levels of G 1 cyclin are low in G 0 phase and increase progressively upon addition of growth factors or mitogens to the cells. The mechanisms by which mitogens or growth factors activate cyclin D1 are complex and occur at both transcriptional and posttranscriptional levels. At the transcriptional level, induction of cyclin D1 by growth factors is dependent on the RAS/RAF/mitogen- activated kinase (MEK)/extracellular signal-regulated kinase (ERK) signaling pathway. Once synthesized, cyclin D1 protein has a short half-life —its turnover being governed by ubiquitination and proteasomal degradation, which in turn are dependent on phosphorylation of cyclin D1 by glycogen synthase kinase-3β (GSK-3β). Growth factors prevent cyclin D1 degradation by inhibiting GSK-3β-dependent phosphorylation of cyclin D1 through the Ras/phosphatidylinositol-3-OH kinase (PI3K)/AKT pathway. It was subsequently demonstrated that growth factor induced cyclin D1 gene transcription by the MEK/ERK pathway and cyclin D1 protein stabilization by the PI3K/AKT pathway need to occur in a sequential manner with the former occurring early and the latter occurring late in the G 1 phase in order to drive the progression of the cell cycle.
One of the key targets of an activated cyclin D-CDK4/6 complex is the retinoblastoma (RB) protein. RB is one of three “pocket protein” family of cell cycle regulator proteins—the other two being p107 and p130—and has a major role in restraining the transition between G 1 and S phases of the cell cycle. In the absence of mitogenic stimuli, RB interacts with and inhibits the activity of the transcription factor E2F. As E2F-binding sites are present in the promoters of many genes required for cell cycle progression, the inhibition of E2F by RB prevents entry into the cell cycle. In addition to physically interacting with E2F, RB also recruits chromatin remodeling enzymes such as histone deacetylases (HDACs) that often serve as transcription corepressors. Thus, the binding of RB to E2F not only simply inhibits E2F activity, but the RB-E2F complex binds to promoters and actively represses transcription by blocking activity of the surrounding enhancers on the promoter.
The activity of RB is governed by phosphorylation catalyzed by CDKs. RB contains 16 potential phosphorylation sites by CDKs and oscillates between hypophosphorylated and hyperphosphorylated forms during the cell cycle. The form that inhibits E2F is the hypophosphorylated form. CDKs, in complex with their cyclin partners, phosphorylate the hypophosphorylated form of RB, leading to the hyperphosphorylated form. At least three different cyclin-CDK complexes are known to phosphorylate RB during the cell cycle-cyclin D-CDK4/6 acts early in G 1 ; cyclin E-CDK2 in late G 1 ; and cyclin A-CDK2 in the S phase. In this way, RB becomes sequentially phosphorylated in the cell cycle. It has been shown that successive phosphorylation of RB by cyclin D-CDK4/6 and cyclin E-CDK2 is necessary for the complete hyperphosphorylation of RB. The hyperphosphorylation of RB prevents its binding to E2F, thus releasing E2F from transcriptional repression. Once liberated from RB, E2F, along with its partner transcription factor DP, activate transcription of genes that are involved in nucleotide metabolism and DNA synthesis, thus allowing entry into the S phase . It is of interest to note that one of the target genes of an active E2F encodes cyclin E. It was also shown that phosphorylation of RB by cyclin D-CDK4/6 and cyclin E-CDK2 triggers sequential intramolecular interactions in RB that progressively block RB functions as cells move through G 1. The complete hyperphosphorylation of RB by cyclin D-CDK4/6 and cyclin E-CDK2 coincides with the R point ( Fig. 8.4 ), beyond which the cell is committed to completion of the cell cycle.
8.2.1.2
G 1 /S Cyclins
Cyclins E1 and E2 (collectively considered as cyclin E) are the G 1 /S cyclins. Transcription of the cyclin E gene is regulated by E2F, which, as described above, is activated due to cyclin D-CDK4/6-stimulated phosphorylation of RB. The amounts of cyclin E protein and its associated kinase (CDK2) activity are maximal in late G 1 and early S phases ( Fig. 8.4 ). Cyclin E-CDK2 completes RB phosphorylation in the G 1 phase and the transition from cyclin D-CDK4/6 to cyclin E-CDK2 accounts for the loss of dependency on growth factors. Cyclin E-CDK2 phosphorylates RB on different sites from cyclin D-CDK4/6, and these kinases may differentially impact the interaction between RB and E2Fs, HDACs, and other chromatin remodeling proteins. In contrast to cyclin D-CDK4/6, the functions of cyclin E-CDK2 are not limited to G 1 control. Thus, cyclin E-CDK2 phosphorylates other substrates that are more directly involved in the control of DNA replication, centrosome duplication, replication origin licensing and firing. The timing of cyclin E-CDK2 activation and its broader range of substrates suggest that cyclin E-CDK2 spans the interface between G 1 regulation and core cell cycle machinery during S phase.
In early S phase, cyclin E-CDK2 activity abruptly ceases as a consequence of cyclin E degradation ( Fig. 8.4 ). This is mediated by phosphorylation by both GSK-3β and CDK2, which target cyclin E for ubiquitination by the SCF Fbw7 E3 ligase, leading it to proteasomal degradation. Like cyclin D1, the involvement of GSK-3β, an enzyme that is inhibited by the PI3K/AKT signaling pathway, in regulating stability of cyclin E suggests that cyclin E can be influenced at least by one mitogen-dependent signaling cascade.
8.2.1.3
S Cyclins
The S cyclins include both cyclins A1 and A2. While cyclin A1 is restricted to the germ cell lineages, cyclin A2 is ubiquitously expressed in all cell types. Low levels of cyclin A2 are first detected at the G 1 /S boundary. The levels then rise steadily as cells begin to replicate their DNA and do not decline until cyclin A is degraded in late G 2 ( Fig. 8.4 ). In S phase, cyclin A and its partner, CDK2, phosphorylate substrates that commence DNA replication from preformed replication initiation complexes. Cyclin A-CDK2 are also required to coordinate the end of the S phase with activation of the mitotic cyclin-CDKs.
8.2.1.4
M Cyclins
During G 2 , A-type cyclins (the S cyclins) are degraded by ubiquitin-mediated proteolysis whereas B-type cyclins (the M cyclins) are actively synthesized ( Fig. 8.4 ). As a consequence, CDK1 (also known as Cdc2) binds to B-type cyclins—an association required for the commencement of mitosis. CDK1 preferentially binds to two main B-type cyclins, cyclins B1 and B2. In contrast, the third isoforms, cyclin B3, may have a function in the meiotic cell cycle. Cyclin B-CDK1 regulate events during both the G 2 /M transition and progression through mitosis. This is accomplished by the phosphorylation of over 70 proteins by the cyclin B-CDK1 complexes although the number of substrates could be much larger. Phosphorylation of target proteins leads to numerous events that include separation of centrosomes. condensation of chromosomes, breakdown of the nuclear lamina, and disassembly of the Golgi apparatus, among others. Finally, inactivation of the cyclin B-CDK1 complexes is required for proper exit from mitosis and this inactivation is achieved by the degradation of B-type cyclins by ubiquitin-mediated proteolysis that is regulated by the anaphase-promoting complex/cyclosome (APC/C).
8.2.2
Cyclin-Dependent Kinases (CDKs)
CDKs are the catalytic subunits of a relatively large family of serine/threonine protein kinases with a primary role in cell cycle progression. The mammalian genome contains 11 genes encoding CDKs and nine others encoding CDK-like proteins with conserved structure. The prototype CDK, CDK1, was first identified in yeasts and designated as Cdc2 in Saccharomyces pombe or Cdc28 in Saccharomyces cerevisiae . The mammalian homologue of yeast Cdc2, CDK1, was subsequently identified due to its ability to complement the yeast mutants. Other mammalian CDKs were then identified by a host of techniques including complementation, homology probing, differential display, and PCR amplification with degenerate primers. Again, as described above, progression through the G 1 phase of the cell cycle requires at least three CDKs—CDK4, CDK6, and CDK2—which, along with their regulatory cyclins, target the RB family of proteins that include RB, p107, and p130. CDK2 is also important for S phase progression and CDK1 for G 2 /M transition and mitosis.
The kinase activities of CDKs are regulated at multiple levels, including interaction with their regulatory subunits (cyclins), binding to negative regulators called CDK inhibitors or CKIs (see below), phosphorylation-dephosphorylation, folding and subcellular localization. Among these, phosphorylation-dephosphorylation can have activating or inhibitory effects on CDK activity. For example, active cyclin- CDK complexes need to be phosphorylated in the T-loop of the CDK subunit by CDK-activating kinase (CAK), which contains a complex of CDK7, cyclin H, and MAT1 ( Fig. 8.5 ). In contrast, cyclin-CDK complexes can be negatively regulated by phosphorylation in adjacent threonine and tyrosine residues of the CDK subunit by the dual-specificity protein kinases WEE1 and MYT1. Conversely, these inhibitory phosphorylations can be reversed by the ability of the dual-specificity CDC25 phosphatases (CDC25A, CDC25B, and CDC25C) to dephosphorylate the same threonine and tyrosine residues and thus act as positive regulators of cyclin-CDK activity ( Fig. 8.5 ). If both activating and inactivating phosphorylations exist in the same molecule, they result in an inactive kinase.

8.2.3
In Vivo Functions of Cyclins and CDKs as Revealed by Gene Knockout in Mice
Numerous studies have investigated the in vivo functions of cyclins and CDKs in knockout mice with the genes deleted either singly or in combination. Since it is not the intent of this chapter to review all of the phenotypes of the knockout mice, the readers are referred to the many excellent recent review articles that summarize the findings of these studies. From these studies, it becomes clear that most of the cyclins and CDKs, although previously considered essential for cell proliferation, have turned out to be dispensable. Several compensatory mechanisms were uncovered among cyclins and CDKs. The particularly unexpected finding is that CDK2, thought to be a master regulator of the cell cycle, is dispensable for the regulation of the cell cycle with both CDK4 and CDK1 covering CDK2’s functions. In fact, CDK1 alone is able to drive the mammalian cell cycle, indicating that the regulation of the mammalian cell cycle is highly conserved.
8.2.4
CDK Inhibitors (CKIs)
CDK inhibitors (CKIs) are proteins that constrain the activities of CDKs. Two classes of CDK inhibitors have been described. The first class includes the INK4 proteins ( in hibitors of CD K4 ). Four such INK4 proteins have been identified: p16 INK4a (also known as CDK inhibitor 2A or CDKN2A), p15 INK4b (CDKN2B), p18 INK4c (CDKN2C), and p19 INK4d (CDKN2D). INK4 proteins specifically bind to and inhibit monomeric CDK4 and CDK6 proteins. The second class of CKIs includes the Cip/Kip ( C DK- i nteracting protein/CD K – i nteracting p rotein) family of proteins which are more broadly acting than the INK4 family of proteins and do so by binding to cyclin-CDK complexes. There are three members of the Cip/Kip family of CKIs: p21 Cip1 (also called CDK inhibitor 1A or CDKN1A), p27 Kip1 (CDKN1B), and p57 Kip2 (CDKN1C). Cip and Kip inhibitors block CDK activity by forming inactive trimeric complexes (cyclin E-CDK2, cyclin A-CDK2, cyclin B-CDK1, and possibly cyclin D-CDK4 and cyclin D-CDK6), thus exerting a much broader effect on the progression of the cell cycle. Fig. 8.5 summarizes the multiple regulatory mechanisms by which CDK activities are regulated.
8.3
Checkpoints
The cell cycle contains several checkpoints to monitor and regulate its progression. Checkpoints are positioned at specific locations in the cell cycle to allow verification of phase processes and repair of DNA damage. A cell cannot proceed from one phase to the next without satisfying all of the checkpoint requirements. An important function of many of the cell cycle checkpoints is to assess DNA damage. Upon detection of DNA damage, the checkpoint initiates a signal cascade to either arrest the cell cycle until repairs are properly made, or if repairs are not possible, to target the cell for destruction via apoptosis as a means to maintain genomic integrity. In the cell cycle, there are three specific checkpoints for damaged or incompletely replicated DNA: G 1 /S, G 2 /M, and intra-S checkpoints. These checkpoints are patrolled by some of the CKIs described above. A fourth important and specific checkpoint occurs in mitosis, the so-called mitotic checkpoint or SAC. This checkpoint is designed to monitor proper alignment of the chromosomes during mitosis. Anaphase cannot proceed unless this checkpoint is satisfied. The locations of the various checkpoints in the cell cycle are illustrated in Fig. 8.6 . The various cell cycle checkpoint pathways in response to DNA damage are summarized in Fig. 8.7 .


8.3.1
G 1 /S Checkpoint
The G 1 /S checkpoint (also called the G 1 checkpoint) is located near the end of the G 1 phase; just before the entry into S phase ( Fig. 8.6 ). In mammalian cells, the G 1 checkpoint is the restriction or R point ( Fig. 8.4 ). This is a point where cells typically arrest the cell cycle if environmental conditions are unfavorable for cell division, such as the presence of DNA damage or lack of growth factors. The G 1 checkpoint is controlled by both the INK4 and Cip/Kip families of CKIs. INK4 proteins specifically bind to CDK4 and CDK6 and inhibit their activity. Enforced expression of INK4 proteins arrest cells in the G 1 phase in an RB-dependent manner. Here CDK4 is redistributed from cyclin D-CDK4 complexes to INK4-CDK4 complexes, and unbound D-type cyclin is rapidly degraded by ubiquitination-mediated proteasomal pathway. Also, in early G 1 phase, the cyclin E-CDK2 and cyclin A-CDK2 complexes are inhibited by bound p21 Cip1 and p27 Kip1 . In addition, cyclin D-CDK4/6 complexes bind p21 Cip1 and p27 Kip1 . Loss of D-type cyclins therefore prevents the titration of p21 Cip1 and p27 Kip1 by cyclin D-CDK4/6 complexes away from the cyclin E-CDK2 and cyclin A-CDK2 complexes. As a result, there is complete inhibition of cyclin E-CDK2 and cyclin A-CDK2 activities as well as RB phosphorylation, leading to exit from the G 1 phase. Conversely, growth-induced or oncogenic-induced expression of cyclin D allows its interaction with CDK4/6 by competing with INK4 for binding. The binding of cyclin D-CDK4/6 complexes to p21 Cip1 and p27 Kip1 releases the inhibitors from the cyclin E-CDK2 and cyclin A-CDK2 complexes. This unleashes cyclin E-CDK2 and cyclin A-CDK2 activity, allowing further RB phosphorylation, exit from G 1 and entry into S phase.
The G 1 /S checkpoint is activated upon detection of DNA damage. The mammalian DNA-damage response is a complex network, involving a multitude of proteins that include “sensor” proteins that sense the damage and transmit signals to “transducer” proteins, which, in turn, convey the signals to numerous “effector” proteins implicated in specific cellular pathways, including DNA repair mechanisms, cell cycle checkpoints, cellular senescence, and apoptosis. In response to DNA damage, signals initiated by the sensors rapidly transduce to the ATM (ataxia telangiectasia, mutated) and ATR (ataxia telangiectasia and Rad3-related) kinases, which phosphorylate a great number of substrates. Among the substrates phosphorylated by activated ATM and ATR are the checkpoint serine/threonine kinases, CHK1 (checkpoint kinase 1) and CHK2 (checkpoint kinase 2). To prevent entry into S phase, CHK1 and CHK2 phosphorylate the cell cycle regulatory phosphatase CDC25A, leading to its ubiquitin-mediated proteolysis. Inactivation of CDC25A leads to sustained inhibitory phosphorylation of cyclin E-CDK2 complexes, thus preventing G 1 /S transition ( Fig. 8.5 ). Both ATM and ATR belong to the phosphatidylinositol-3-kinase-like kinase family (PIKKs), which also includes a third member of the DNA damage response transducer, DNA-PKcs (DNA-dependent protein kinase). DNA-PKcs appears to regulate a smaller number of targets and plays a role primarily in nonhomologous end joining (NHEJ) of double-strand DNA breaks. ATM/ATR and CHK1/CHK2 kinases also target the tumor suppressor, p53. The phosphorylation of p53 by ATM/ATR inhibits its association with MDM2, an E3 ubiquitin ligase normally functions to keep the p53 level low, and this results in stabilization of the p53 protein. Subsequently, p53 transcriptionally activates expression of the p21 Cip1 gene, which in turn inhibits cyclin E-CDK2 and prevents G 1 /S transition. Lastly, rapid degradation of cyclin D1 in response to DNA damage occurs that is independent of p53. The reduced cyclin D1 protein decreases the amount of cyclin D-CDK4/6 complexes, resulting in the redistribution of p21 Cip1 to cyclin E-CDK2 complexes, resulting in the latter’s inactivation.
8.3.2
G 2 /M Checkpoint
The G 2 /M checkpoint (also known as G 2 checkpoint) prevents cells from initiating mitosis when they experience DNA damage while in G 2 , when they progress into G 2 with either unrepaired DNA sustained during the previous S or G 1 phase, or when they possess incompletely replicated DNA from S phase. The critical target of the G 2 checkpoint is the mitosis-promoting activity of the cyclin B-CDK1 complexes, whose activation after genotoxic stresses is inhibited by ATM/ATR, CHK1/CHK2-mediated degradation of CDC25 family of phosphatases, which normally activate CDK1 at the G 2 /M boundary. In addition, other regulators of CDC25 and cyclin B-CDK1, such as the Polo-like kinases (PLKs) are targeted by DNA damage-induced mechanisms. Finally, the maintenance of the G 2 checkpoint is dependent on the transcriptional programs regulated by p53, leading to an induction of cell cycle inhibitors such as p21 Cip1 , growth arrest and DNA damage-inducible 45 (GADD45), and 14-3-3σ proteins. These proteins cooperatively inhibit cyclin B-CDK1 activity by directly binding to cyclin B-CDK1 (p21 Cip1 ), dissociating CDK1 from cyclin B (GADD45), and sequestering CDK1 in the cytoplasm (14-3-3σ), resulting in G 2 arrest.
8.3.3
Intra-S Checkpoint
While proliferating cells respond to genotoxic stresses by activating checkpoint responses that impose durable cell cycle arrest in G 1 or G 2 , before entry into S phase or mitosis, respectively, cells that experience genotoxic stresses during DNA replication can only delay their progression through the S phase in a transient fashion. If the damage is not repaired during the delay, cells exit S phase and arrest upon reaching the G 2 checkpoint. Nonetheless, the intra-S checkpoint is important for the maintenance of genomic stability as DNA replication is a vulnerable period of the cell cycle in which errors occur both endogenously or are introduced exogenously. When the intra-S checkpoint is activated, both replication initiation and fork progression are inhibited, thus reducing the rate of DNA replication. Studies have revealed numerous proteins that are involved in the control of intra-S checkpoint. Among these, activation of ATM and ATR is necessary to initiate intra-S checkpoint upon sensing DNA double-strand breaks (DSBs). Activated ATM and ATR then activates CHK1 and CHK2 kinases, which phosphorylate CDC25A, leading to its ubiquitin-proteosome-dependent degradation. This results in persistent inhibitory phosphorylation of CDK2 in the cyclin A-CDK2 complexes, which, in turn, prevents firing of the replication origins. A second independent pathway is involved in slowing down the replication rate in cells that have suffered from DNA damage. This effect is mediated by ATM-dependent phosphorylation of a cohesion protein, structural maintenance of chromosomes-1 (SMC-1). However, the mechanism by which phosphorylated SMC-1 interferes with DNA replication remains unknown.
8.3.4
Mitotic Checkpoint or SAC
Mitosis is the process in which a cell divides itself into two halves, each with an identical set of chromosomes ( Fig. 8.2 ). The central regulator of this process is the mitotic checkpoint, also known as the spindle assembly checkpoint or SAC, a signaling mechanism that arrests the progression of metaphase to anaphase until all chromosomes are attached to the mitotic spindles. This signal is akin to an “anaphase wait” signal that is generated at the kinetochores of unattached chromosomes and is extinguished once all kinetochores are properly attached to the spindles ( Fig. 8.2 ). Thus, sister chromatids are separated only when they are in a position to be equally distributed to the two daughter cells. Accordingly, the mitotic checkpoint serves to prevent chromosome mis-segregation.
The proteins that control mitotic checkpoint were originally identified by screens for mutations that bypassed the ability of wild type S. cerevisiae to arrest in mitosis in the presence of spindle poisons. The genes identified include MAD (mitotic-arrest deficient), MAD1, MAD2, MAD3 (BUBR1 in humans), and BUB1 (budding uninhibited by benzimidazole 1). It was later found that these genes are conserved in all eukaryotes. When activated, these SAC proteins target CDC20, which is a co-factor of the ubiquitin ligase anaphase promoting complex/cyclosome (APC/C). Specifically, SAC inhibits the ability of CDC20 to activate APC/C-mediated ubiquitination of two key substrates, cyclin B and securin, thus preventing their degradation by the 26S proteosome. Proteolysis of cyclin B inactivates CDK1, allowing the cells to exit from mitosis. On the other hand, destruction of securin leads to activation of separase, which is required to cleave the cohesion complex that holds sister chromatids together in order to execute anaphase. Therefore, by keeping CDC20 in check, the SAC allows the chromosomes to properly align on the metaphase plate and attached to the two spindle poles through mitotic spindles. Once the chromosomes are properly oriented, the checkpoint is extinguished, relieving the mitotic arrest and allowing anaphase to proceed.
8.4
Noncanonical Functions of Cyclins, CDKs, and CKIs
Close cooperation among cyclins, CDKs, and CKIs is necessary for ensuring orderly progression through the cell cycle. However, evidence has emerged that the roles of this trio are beyond cell cycle regulation. The “noncanonical” functions of cyclins, CDKs and CKIs involve myriads of other cellular processes such as transcription, DNA damage repair, apoptosis, cell differentiation, epigenetic regulation, stem cell self-renewal, metabolism, and the immune response. Some of these functions are performed by cyclins or CDKs independent of their respective cell cycle partners, suggesting that there is substantial divergence in their functions during evolution. For example, D-type cyclins, independent of any associated kinase activity, are known to have direct roles in regulating transcription by interacting with many transcription factors to activate or repress transcription of specific genes. Similarly CDK6, but not CDK4, can regulate angiogenesis and myloid differentiation, respectively, by modulating the transcriptional activity of JUN and RUNX1.
In addition to regulating the cell cycle, the trio of cyclins, CDKs, and CKIs exerts important functions in the repair of DNA damage sustained from DSBs. DNA DSBs are repaired by two different mechanisms: homologous recombination and NHEJ. Cyclin D1 has been shown to localize to DNA DSBs and to recruit RAD51, which activates HR-mediated DNA repair. CDK2 was also shown to support HR by promoting the interaction between breast cancer type 1 susceptibility protein (BRCA1) and the MRE11 exonuclease, leading to the resection of DSBs. These observations suggest that there is a profound, two-way connection between DNA damage repair and cell cycle control.
The Cip/Kip family of CKIs (p21 Cip1 , p27 Kip1 , and p57 Kip2 ) also exhibit functions independent of their ability to inhibit cyclin-CDK complexes. They have been found to be key regulators of transcription, apoptosis, and actin cytoskeletal dynamics. For example, p21 Cip1 binds to the transcription factors E2F-1, STAT3 and c-Myc to inhibit their transcriptional activities, and binds to p300/CBP to block its transcriptional repressor activity. p21 Cip1 also binds and inhibits JNK1/SAPK kinase and MAPK-kinase-kinase ASK1/MEKK5 to block stress-induced apoptosis. The antiapoptotic activities are attributed to the cytoplasmic pool of p21 Cip1 . Finally all three Cip/Kip CKIs regulate actin cytoskeletal dynamics by modulating the RhoA-ROCK-LIMK-cofilin pathway, leading to redistribution of monomeric actin and contributing to increase cell motility and morphological changes. These divergent functions are performed in distinct cellular compartments and contribute to the seemingly contradictory observation that the CKIs can both suppress or promote tumor formation.
8.5
Pathological Consequences of Cell Cycle Deregulation or Dysregulation
Because regulation of the cell cycle is central to the control of cell proliferation, it is not surprising that cancers are often the results of deregulation or dysregulation of the cell cycle. Take colorectal cancer, for example, recent genomic-scale sequencing studies have identified numerous somatic mutations in genes that possibly are involved in the formation of cancer. Among these, some of the most highly ranked “cancer genes” are either directly or indirectly involved in the regulation of the cell cycle. Examples include p53, adenomatous polyposis coli (APC), KRAS, F-box and WD40 domain protein 7 (FBXW7), and phosphatidylinositol 3-kinase, catalytic, alpha subunit (PI3KCA). Here we briefly review some of the common cancer genes with cell cycle regulatory functions.
8.5.1
Retinoblastoma (RB) Tumor Suppressor Gene
The RB tumor suppressor protein limits cell proliferation by preventing entry into the S phase of the cell cycle. RB achieves its inhibitory effect by blocking the activity of E2F. Progression into S phase occurs when the ability of RB to suppress E2F is disrupted by the hyperphosphorylation of RB by cyclin D- and cyclin E-dependent CDKs in the G 1 phase of the cell cycle. The INK4 family of CKIs, particularly p16 INKa , directly inhibits activities of the cyclin D-dependent kinases, CDK4 and CDK6, thus maintaining RB in its active, antiproliferative state. Functional disruption of the tumor suppressors, p16 INKa and RB, or overexpression of the proto-oncogene products, cyclin D1 and CDK4, occur in many human cancers, prompting the speculation that disabling the “RB pathway” is an essential part of cancer formation. In addition to being causative in RB, loss of RB has been found in many other cancers. Similarly, inactivating mutations of 16 INK4a have been identified in numerous tumors. The often exclusive nature of mutations that result in RB or p16 INK4a loss, and/or cyclin D1 or CDK4 overexpression, suggest that each of these cell cycle regulatory genes exerts a critical function in the RB pathway of cancer formation. As such RB and its effectors prove to be potentially powerful predictive, prognostic and therapeutic target in cancer.
8.5.2
p53 and INK4a/ARF Tumor Suppressor Genes
The tumor suppressor p53 is mutated in more than 50% of human cancers. It has been estimated that cancers derived from over 50 human cell types or tissues contain mutations in the p53 gene. p53 is a labile protein but accumulates in response to cellular stresses from DNA damage, hypoxia, or oncogenic activation. Upon stabilization and activation, p53 initiates a transcriptional program that triggers either cell cycle arrest or apoptosis. Among the p53-responsive genes are p21 Cip1 , BCL2-associated X protein (BAX), and mouse double-minute 2, homolog (MDM2). While p21 Cip1 regulates progression of the cell cycle by inhibiting cyclins (E, A, and B)-CDK2 complexes, BAX causes apoptosis. The transcriptional induction of MDM2 by p53 is a negative feedback mechanism as binding of MDM2, an E3 ubiquitin ligase, to p53 induces ubiquitination of p53 and subsequent degradation. MDM2, in turn, is negatively regulated by the ARF (alternative reading frame) tumor suppressor (p14 ARF in humans and p19 ARF in mice). ARF directly associates with MDM2 to block its interaction with p53, therefore stabilizing p53. Thus, disruption of the ARF-MDM2-p53 signaling pathway is a common feature in cancers. This is supported by the finding that MDM2 is overexpressed in 5%–10% of human cancers, whereas ARF is silenced or deleted in many others. It is of interest to note that ARF is derived from an alternative reading frame in exon 2 of the INK4a gene. Loss of the INK4a/ARF locus therefore predisposes to many tumor types due to the dual disruption of the RB-E2F and MDM2-p53 pathways.
8.5.3
hCDC4/FBXW7 Tumor Suppressor Gene
Abundant evidence indicates that ubiquitin-dependent proteolysis regulates many aspects of the cell cycle and that dysregulation of the ubiquitin-proteosome pathway contributes to the formation of cancers. The proteolytic regulation of cell division is primarily controlled by two ubiquitin ligase systems, APC/C and SCF. The APC/C ubiquitin ligase is a multimeric protein complex that regulates chromosomes segregation (see above, The Mitotic Checkpoint or Spindle Assembly Checkpoint). SCF ligase complexes are structurally similar to APC/C and composed of an invariable core complex of SKP1, CUL1, and RBX1, and associated with a variable member of the F-box protein family that serves as the substrate recognition component. Among the approximately 70 different F-box proteins that were identified, the human homologue of yeast CDC4 (hCDC), also known as F-box and WD40-domain protein 7 (FBXW7), has been extensively characterized and implicated in human tumorigenesis. For example, among the approximately 140 mutated “cancer genes” identified in a recent genomic screen in colorectal cancers, FBXW7 ranks fifth as the most frequently mutated gene (after APC, KRAS, p53, and PI3K). Recurrent alterations in FBXW7 are also found in colorectal adenomas.
Human FBXW7 exists in three different isoforms, α, β, and γ, each with a unique amino terminal end fused to a common carboxyl terminal. The interaction between FBXW7 and its substrates depends on phosphorylation of the substrate within a motif called the CDC4-phophodegredron or CPD. This feature enables FBXW7 to simultaneously regulate a host of substrates by ubiquitination. Among the many substrates for FBXW7, some are critically involved in the regulation of the cell cycle such as cyclin E, c-Myc, c-Jun, and Notch. Mutations in the FBXW7 gene therefore lead to stabilization and elevated levels of these substrates. It is no wonder that FBXW7 is such a commonly mutated gene in human cancers.
One of the best characterized substrates of FBXW7 is cyclin E, which is essential for entry into S phase from G 1 phase in the cell cycle. Cyclin E level is elevated or dysregulated in many human cancers, resulting in dysfunction of the cell cycle. The consequences of cyclin E deregulation are multitude and include genetic instability, centrosome amplification, and fork collapse during DNA replication. Several studies subsequently identified cyclin E as a substrate for FBXW7, which mediates the phosphorylation- and ubiquitination-dependent degradation of cyclin E. Thus, it appears that tumorigenesis secondary to cyclin E deregulation is linked to altered function of FBXW7/hCDC4.
8.5.4
Chromosomal Instability (CIN) and Aneuploidy as Consequences of an Aberrant Mitotic Checkpoint
Genetic instability has long been recognized as an integral part of human cancers. In colorectal cancer (CRC), there are two major forms of genetic instability: microsatellite instability (MIN) and chromosomal instability (CIN). MIN tumors have mutations in the DNA mismatch repair (MMR) genes and accounts for approximately 15%–20% of sporadic CRC. The remainders of the sporadic CRC have CIN, which are frequently aneuploid, that is, they exhibit alterations in the number of chromosomes. It has been suggested that CIN is the driving force for the formation of aneuploidy and tumorigenesis. Although there has not been a unified mechanism responsible for CIN, defects in several cellular processes have been causally linked to its formation. These include chromosome dynamics (e.g., chromosome condensation, segregation, cohesion, and kinetochore-spindle interaction), centrosome duplication, cell cycle checkpoints (include G 1 , S, G 2, and the SACs), DNA damage repair pathway, and telomere functions. Among these potential factors contributing to CIN, the mitotic checkpoint is probably the most important one since it is an essential part of the cell cycle that ensures equal distribution of chromosomes upon the conclusion of cell division. Studies in mice lacking specific components of the mitotic checkpoint support this view. Mice with genetically reduced levels of mitotic checkpoint proteins including MAD1, MAD2, BUB1, BUB3, BUBR1, and centromeres protein E (CENP-E) all have increased level of aneuploidy and CIN, with the eventual formation of tumors in some animals. Importantly, somatic mutations of many of the same genes have been identified in human cancers, indicating the importance of the mitotic checkpoint in maintaining genomic integrity. These findings render the concept of exploiting the genetic instability of cancer cells through therapeutic intervention of the mitotic checkpoint a distinct possibility.
8.5.5
The Adenomatous Polyposis Coli Tumor Suppressor Gene
Germline mutations of the APC (note: different from APC/C) tumor suppressor gene cause familial adenomatous polyposis (FAP), an autosomal dominant disorder characterized by the presence of numerous colonic adenomas and colon cancer early in life. Somatic mutations in the APC gene were subsequently found to be present in the majority of sporadic colorectal cancer. Its involvement in tumor initiation leads to the hypothesis that APC functions as a “gatekeeper” of colonic epithelial cell proliferation and is responsible for the maintenance of colonic epithelial cell renewal. A telling insight into the function of APC came from the observation that it interacts with β-catenin. β-Catenin is implicated in regulating the Wnt signaling pathway of growth control. Particularly, Wnt signaling is now a well-established central mechanism for regulating proliferation of intestinal epithelial stem cells that are the precursors to all subsequent intestinal epithelial cell lineages.
Wnt signaling is normally absent in a quiescent, noncycling cell. This is accompanied by the sequestration of β-catenin in a “destruction complex” in the cytoplasm that includes APC, Axin, casein kinase 1 (CK1), and glycogen synthase kinase 3 (GSK3) ( Fig. 8.8 A). This complex leads to the phosphorylation of β-catenin, which is then degraded by ubiquitin-mediated proteasomal degradation. When Wnt, a secreted glycoprotein, is present, it binds to the cell surface receptors Frizzled (Fz) and lipoprotein receptor-related protein (LRP). This leads to activation of the protein disheveled (Dsh) and subsequent release of β-catenin from the destruction complex, resulting in accumulation of free β-catenin in the cytoplasm ( Fig. 8.8 B). Some of this free β-catenin is shuttled into the nucleus where it becomes associated with the transcription factor, T-cell factor (TCF) to activate target gene expression ( Fig. 8.8 B). Among the genes stimulated by the β-catenin/TCF complexes are those encoding cyclin D1 and c-Myc, both of which are critical for the progression of the cell cycle. It is important to note that mutational inactivation of APC or constitutional mutational activation of β-catenin, two common events in the pathogenesis of colorectal cancer, results in a similar net effect of nuclear β-catenin accumulation to that seen during Wnt signaling. These mutations then lead to unregulated cell proliferation.

APC has also been implicated in the regulation of mitosis in a β-catenin-independent manner. Here APC is localized to the ends of microtubules embedded in kinetochores and forms a complex with several SAC proteins. This finding suggests that APC is involved in chromosome segregation. The role of APC in regulation of mitosis is consistent with the finding that mutations in the APC gene cause chromosomal instability. Thus, APC may fulfill two requirements for a colonic epithelial cell to develop into colon cancer—the cell must acquire a selective advantage to allow for an initial clonal expansion, and generate genetic instability to allow for multiple hits in other genes that are responsible for tumor progression and malignant transformation.
8.5.6
Cell Cycle Regulators as Targets for Cancer Treatment
Because deregulation or dysregulation of the cell cycle is frequently found in cancer, the cell cycle regulatory proteins are logical targets for development of novel theories for cancer. Recent studies have demonstrated that orally available small-molecule inhibitors of the cyclin D-dependent CDK4 and CDK6, when combined with established therapies, have potential in the treatment of certain cancers. A recently completed phase 3 clinical trial is an example of a success story that the combination of palbociclib, a CDK4-CDK6 inhibitor, and letrozole, an aromatase inhibitor, is highly effective in the treatment of advanced breast cancer. Given that many additional novel similar agents are in various stages of development, cell cycle-based targeted therapy promises to become part of a new armamentarium in the combat against cancer.
8.6
Conclusion
The cell cycle is a fundamental cellular process in which a cell duplicates itself in a highly faithful manner to maintain genomic integrity. The cell cycle is divided into several distinct phases that are controlled by specific pairs of cyclin-CDK. The cell cycle also contains specific checkpoints with which to monitor the presence of damaged or incompletely replicated DNA in interphase, or unaligned chromosomes in mitosis. Dysregulation or deregulation of the cell cycle often have pathological consequences and a good example is cancer. Hence, many of the cell cycle regulators act as tumor suppressors or oncoproteins such as Rb, p53, FBXW7, and cyclins. The relevance of cell cycle regulation also has an impact on the physiology of the gastrointestinal tract as the epithelium is composed of rapidly proliferating cells. A prominent example is the crucial role of the Wnt signaling pathway in regulating proliferation of intestinal stem cells and that a critical component of the Wnt pathway, the APC protein, is mutated in the majority of colorectal cancer. Recent clinical studies have also shown promising results in cancer treatment by targeting cell cycle regulatory proteins. Thus, a clear understanding of the cell cycle machinery is essential not only for understanding the mechanisms regulating proliferation of intestinal epithelial cells, but developing novel therapies for cancer.
References
- 1. Sanso M., and Fisher R.P.: Modelling the CDK-dependent transcription cycle in fission yeast. Biochem Soc Trans 2013; 41s: pp. 1660-1665
- 2. Chang F., and Nurse P.: Finishing the cell cycle: control of mitosis and cytokinesis in fission yeast. Trends Genet 1993; 9: pp. 333-335
- 3. Bartlett R., and Nurse P.: Yeast as a model system for understanding the control of DNA replication in Eukaryotes. BioEssays 1990; 12: pp. 457-463
- 4. Nurse P.: The Josef Steiner lecture: CDKs and cell-cycle control in fission yeast: relevance to other eukaryotes and cancer. Int J Cancer 1997; 71: pp. 707-708
- 5. Woo R.A., and Poon R.Y.: Cyclin-dependent kinases and S phase control in mammalian cells. Cell Cycle 2003; 2: pp. 316-324
- 6. Malumbres M., and Barbacid M.: Mammalian cyclin-dependent kinases. Trends Biochem Sci 2005; 30: pp. 630-641
- 7. Sherr C.J., and Roberts J.M.: Living with or without cyclins and cyclin-dependent kinases. Genes Dev 2004; 18: pp. 2699-2711
- 8. Sanchez I., and Dynlacht B.D.: New insights into cyclins, CDKs, and cell cycle control. Semin Cell Dev Biol 2005; 16: pp. 311-321
- 9. Harper J.V., and Brooks G.: The mammalian cell cycle: an overview. Methods Mol Biol 2005; 296: pp. 113-153
- 10. Nurse P.M.: Nobel lecture. Cyclin dependent kinases and cell cycle control. Biosci Rep 2002; 22: pp. 487-499
- 11. Doree M., and Hunt T.: From Cdc2 to Cdk1: when did the cell cycle kinase join its cyclin partner? J Cell Sci 2002; 115: pp. 2461-2464
- 12. Blagosklonny M.V., and Pardee A.B.: The restriction point of the cell cycle. Cell Cycle 2002; 1: pp. 103-110
- 13. Musgrove E.A.: Cyclins: roles in mitogenic signaling and oncogenic transformation. Growth Factors 2006; 24: pp. 13-19
- 14. Choi Y.J., and Anders L.: Signaling through cyclin D-dependent kinases. Oncogene 2014; 33: pp. 1890-1903
- 15. Sherr C.J., and Roberts J.M.: CDK inhibitors: positive and negative regulators of G1-phase progression. Genes Dev 1999; 13: pp. 1501-1512
- 16. Filmus J., Robles A.I., Shi W., Wong M.J., Colombo L.L., and Conti C.J.: Induction of cyclin D1 overexpression by activated ras. Oncogene 1994; 9: pp. 3627-3633
- 17. Winston J.T., Coats S.R., Wang Y.Z., and Pledger W.J.: Regulation of the cell cycle machinery by oncogenic ras. Oncogene 1996; 12: pp. 127-134
- 18. Lavoie J.N., L’Allemain G., Brunet A., Muller R., and Pouyssegur J.: Cyclin D1 expression is regulated positively by the p42/p44MAPK and negatively by the p38/HOGMAPK pathway. J Biol Chem 1996; 271: pp. 20608-20616
- 19. Aktas H., Cai H., and Cooper G.M.: Ras links growth factor signaling to the cell cycle machinery via regulation of cyclin D1 and the Cdk inhibitor p27KIP1. Mol Cell Biol 1997; 17: pp. 3850-3857
- 20. Koepp D.M., Harper J.W., and Elledge S.J.: How the cyclin became a cyclin: regulated proteolysis in the cell cycle. Cell 1999; 97: pp. 431-434
- 21. Diehl J.A., Zindy F., and Sherr C.J.: Inhibition of cyclin D1 phosphorylation on threonine-286 prevents its rapid degradation via the ubiquitin- proteasome pathway. Genes Dev 1997; 11: pp. 957-972
- 22. Diehl J.A., Cheng M., Roussel M.F., and Sherr C.J.: Glycogen synthase kinase-3beta regulates cyclin D1 proteolysis and subcellular localization. Genes Dev 1998; 12: pp. 3499-3511
- 23. Jones S.M., and Kazlauskas A.: Growth-factor-dependent mitogenesis requires two distinct phases of signalling. Nat Cell Biol 2001; 3: pp. 165-172
- 24. Bartek J., Bartkova J., and Lukas J.: The retinoblastoma protein pathway and the restriction point. Curr Opin Cell Biol 1996; 8: pp. 805-814
- 25. Weinberg R.A.: The retinoblastoma protein and cell cycle control. Cell 1995; 81: pp. 323-330
- 26. Harbour J.W., and Dean D.C.: The Rb/E2F pathway: expanding roles and emerging paradigms. Genes Dev 2000; 14: pp. 2393-2409
- 27. Cobrinik D.: Pocket proteins and cell cycle control. Oncogene 2005; 24: pp. 2796-2809
- 28. Chellappan S.P., Hiebert S., Mudryj M., Horowitz J.M., and Nevins J.R.: The E2F transcription factor is a cellular target for the RB protein. Cell 1991; 65: pp. 1053-1061
- 29. Nevins J.R.: Toward an understanding of the functional complexity of the E2F and retinoblastoma families. Cell Growth Differ 1998; 9: pp. 585-593
- 30. Dyson N.: The regulation of E2F by pRB-family proteins. Genes Dev 1998; 12: pp. 2245-2262
- 31. Blake M.C., and Azizkhan J.C.: Transcription factor E2F is required for efficient expression of the hamster dihydrofolate reductase gene in vitro and in vivo. Mol Cell Biol 1989; 9: pp. 4994-5002
- 32. Thalmeier K., Synovzik H., Mertz R., Winnacker E.L., and Lipp M.: Nuclear factor E2F mediates basic transcription and trans-activation by E1a of the human MYC promoter. Genes Dev 1989; 3: pp. 527-536
- 33. Dalton S.: Cell cycle regulation of the human cdc2 gene. EMBO J 1992; 11: pp. 1797-1804
- 34. DeGregori J., Leone G., Ohtani K., Miron A., and Nevins J.R.: E2F-1 accumulation bypasses a G1 arrest resulting from the inhibition of G1 cyclin-dependent kinase activity. Genes Dev 1995; 9: pp. 2873-2887
- 35. Magnaghi-Jaulin L., Groisman R., Naguibneva I., Robin P., Lorain S., Le Villain J.P., et al: Retinoblastoma protein represses transcription by recruiting a histone deacetylase. Nature 1998; 391: pp. 601-605
- 36. Kingston R.E., and Narlikar G.J.: ATP-dependent remodeling and acetylation as regulators of chromatin fluidity. Genes Dev 1999; 13: pp. 2339-2352
- 37. Kornberg R.D., and Lorch Y.: Chromatin-modifying and -remodeling complexes. Curr Opin Genet Dev 1999; 9: pp. 148-151
- 38. Collingwood T.N., Urnov F.D., and Wolffe A.P.: Nuclear receptors: coactivators, corepressors and chromatin remodeling in the control of transcription. J Mol Endocrinol 1999; 23: pp. 255-275
- 39. Wolffe A.P., and Hayes J.J.: Chromatin disruption and modification. Nucleic Acids Res 1999; 27: pp. 711-720
- 40. Weintraub S.J., Chow K.N., Luo R.X., Zhang S.H., He S., and Dean D.C.: Mechanism of active transcriptional repression by the retinoblastoma protein. Nature 1995; 375: pp. 812-815
- 41. Meloni A.R., Smith E.J., and Nevins J.R.: A mechanism for Rb/p130-mediated transcription repression involving recruitment of the CtBP corepressor. Proc Natl Acad Sci U S A 1999; 96: pp. 9574-9579
- 42. Ferreira R., Magnaghi-Jaulin L., Robin P., Harel-Bellan A., and Trouche D.: The three members of the pocket proteins family share the ability to repress E2F activity through recruitment of a histone deacetylase. Proc Natl Acad Sci U S A 1998; 95: pp. 10493-10498
- 43. Chow K.N., Starostik P., and Dean D.C.: The Rb family contains a conserved cyclin-dependent-kinase-regulated transcriptional repressor motif. Mol Cell Biol 1996; 16: pp. 7173-7181
- 44. Hinds P.W., Mittnacht S., Dulic V., Arnold A., Reed S.I., and Weinberg R.A.: Regulation of retinoblastoma protein functions by ectopic expression of human cyclins. Cell 1992; 70: pp. 993-1006
- 45. Lundberg A.S., and Weinberg R.A.: Functional inactivation of the retinoblastoma protein requires sequential modification by at least two distinct cyclin-cdk complexes. Mol Cell Biol 1998; 18: pp. 753-761
- 46. Chen P.L., Scully P., Shew J.Y., Wang J.Y., and Lee W.H.: Phosphorylation of the retinoblastoma gene product is modulated during the cell cycle and cellular differentiation. Cell 1989; 58: pp. 1193-1198
- 47. Sherr C.J.: Cancer cell cycles. Science 1996; 274: pp. 1672-1677
- 48. Bertoli C., and de Bruin R.A.: Turning cell cycle entry on its head. elife 2014; 3:
- 49. Narasimha A.M., Kaulich M., Shapiro G.S., Choi Y.J., Sicinski P., and Dowdy S.F.: Cyclin D activates the Rb tumor suppressor by mono-phosphorylation. elife 2014; 3:
- 50. Lam E.W., and La Thangue N.B.: DP and E2F proteins: coordinating transcription with cell cycle progression. Curr Opin Cell Biol 1994; 6: pp. 859-866
- 51. La Thangue N.B.: DP and E2F proteins: components of a heterodimeric transcription factor implicated in cell cycle control. Curr Opin Cell Biol 1994; 6: pp. 443-450
- 52. Adams P.D., and Kaelin W.G.: Transcriptional control by E2F. Semin Cancer Biol 1995; 6: pp. 99-108
- 53. La Thangue N.B.: E2F and the molecular mechanisms of early cell-cycle control. Biochem Soc Trans 1996; 24: pp. 54-59
- 54. Lavia P., and Jansen-Durr P.: E2F target genes and cell-cycle checkpoint control. BioEssays 1999; 21: pp. 221-230
- 55. Berckmans B., and De Veylder L.: Transcriptional control of the cell cycle. Curr Opin Plant Biol 2009; 12: pp. 599-605
- 56. Brehm A., Miska E., Reid J., Bannister A., and Kouzarides T.: The cell cycle-regulating transcription factors E2F-RB. Br J Cancer 1999; 80: pp. 38-41
- 57. Hateboer G., Wobst A., Petersen B.O., Le Cam L., Vigo E., Sardet C., et al: Cell cycle-regulated expression of mammalian CDC6 is dependent on E2F. Mol Cell Biol 1998; 18: pp. 6679-6697
- 58. Black A.R., and Azizkhan-Clifford J.: Regulation of E2F: a family of transcription factors involved in proliferation control. Gene 1999; 237: pp. 281-302
- 59. Dimova D.K., and Dyson N.J.: The E2F transcriptional network: old acquaintances with new faces. Oncogene 2005; 24: pp. 2810-2826
- 60. Bracken A.P., Ciro M., Cocito A., and Helin K.: E2F target genes: unraveling the biology. Trends Biochem Sci 2004; 29: pp. 409-417
- 61. Ohtani K.: Implication of transcription factor E2F in regulation of DNA replication. Front Biosci 1999; 4: pp. D793-D804
- 62. Ohtani K., Iwanaga R., Nakamura M., Ikeda M., Yabuta N., Tsuruga H., et al: Cell growth-regulated expression of mammalian MCM5 and MCM6 genes mediated by the transcription factor E2F. Oncogene 1999; 18: pp. 2299-2309
- 63. Helin K., CL W., Fattaey A.R., Lees J.A., Dynlacht B.D., Ngwu C., et al: Heterodimerization of the transcription factors E2F-1 and DP-1 leads to cooperative trans-activation. Genes Dev 1993; 7: pp. 1850-1861
- 64. Harbour J.W., Luo R.X., Dei Santi A., Postigo A.A., and Dean D.C.: Cdk phosphorylation triggers sequential intramolecular interactions that progressively block Rb functions as cells move through G1. Cell 1999; 98: pp. 859-869
- 65. Zhang H.S., Postigo A.A., and Dean D.C.: Active transcriptional repression by the Rb-E2F complex mediates G1 arrest triggered by p16INK4a, TGFbeta, and contact inhibition. Cell 1999; 97: pp. 53-61
- 66. Geng Y., Whoriskey W., Park M.Y., Bronson R.T., Medema R.H., Li T., et al: Rescue of cyclin D1 deficiency by knockin cyclin E. Cell 1999; 97: pp. 767-777
- 67. Bertoli C., Skotheim J.M., and de Bruin R.A.: Control of cell cycle transcription during G1 and S phases. Nat Rev Mol Cell Biol 2013; 14: pp. 518-528
- 68. Dulic V., Lees E., and Reed S.I.: Association of human cyclin E with a periodic G1-S phase protein kinase. Science 1992; 257: pp. 1958-1961
- 69. Koff A., Giordano A., Desai D., Yamashita K., Harper J.W., Elledge S., et al: Formation and activation of a cyclin E-cdk2 complex during the G1 phase of the human cell cycle. Science 1992; 257: pp. 1689-1694
- 70. Yu Q., and Sicinski P.: Mammalian cell cycles without cyclin E-CDK2. Cell Cycle 2004; 3: pp. 292-295
- 71. Teixeira L.K., Wang X., Li Y., Ekholm-Reed S., Wu X., Wang P., et al: Cyclin E deregulation promotes loss of specific genomic regions. Curr Biol 2015; 25: pp. 1327-1333
- 72. Hu W., Nevzorova Y.A., Haas U., Moro N., Sicinski P., Geng Y., et al: Concurrent deletion of cyclin E1 and cyclin-dependent kinase 2 in hepatocytes inhibits DNA replication and liver regeneration in mice. Hepatology 2014; 59: pp. 651-660
- 73. Clurman B.E., Sheaff R.J., Thress K., Groudine M., and Roberts J.M.: Turnover of cyclin E by the ubiquitin-proteasome pathway is regulated by cdk2 binding and cyclin phosphorylation. Genes Dev 1996; 10: pp. 1979-1990
- 74. Won K.A., and Reed S.I.: Activation of cyclin E/CDK2 is coupled to site-specific autophosphorylation and ubiquitin-dependent degradation of cyclin E. EMBO J 1996; 15: pp. 4182-4193
- 75. Welcker M., Singer J., Loeb K.R., Grim J., Bloecher A., Gurien-West M., et al: Multisite phosphorylation by Cdk2 and GSK3 controls cyclin E degradation. Mol Cell 2003; 12: pp. 381-392
- 76. Sweeney C., Murphy M., Kubelka M., Ravnik S.E., Hawkins C.F., Wolgemuth D.J., et al: A distinct cyclin A is expressed in germ cells in the mouse. Development 1996; 122: pp. 53-64
- 77. Krude T., Jackman M., Pines J., and Laskey R.A.: Cyclin/Cdk-dependent initiation of DNA replication in a human cell-free system. Cell 1997; 88: pp. 109-119
- 78. Hua X.H., and Newport J.: Identification of a preinitiation step in DNA replication that is independent of origin recognition complex and cdc6, but dependent on cdk2. J Cell Biol 1998; 140: pp. 271-281
- 79. Coverley D., Laman H., and Laskey R.A.: Distinct roles for cyclins E and A during DNA replication complex assembly and activation. Nat Cell Biol 2002; 4: pp. 523-528
- 80. Mitra J., and Enders G.H.: Cyclin A/Cdk2 complexes regulate activation of Cdk1 and Cdc25 phosphatases in human cells. Oncogene 2004; 23: pp. 3361-3367
- 81. Nguyen T.B., Manova K., Capodieci P., Lindon C., Bottega S., Wang X.Y., et al: Characterization and expression of mammalian cyclin b3, a prepachytene meiotic cyclin. J Biol Chem 2002; 277: pp. 41960-41969
- 82. Nigg E.A.: Mitotic kinases as regulators of cell division and its checkpoints. Nat Rev Mol Cell Biol 2001; 2: pp. 21-32
- 83. Ubersax J.A., Woodbury E.L., Quang P.N., Paraz M., Blethrow J.D., Shah K., et al: Targets of the cyclin-dependent kinase Cdk1. Nature 2003; 425: pp. 859-864
- 84. Fisher D., Krasinska L., Coudreuse D., and Novak B.: Phosphorylation network dynamics in the control of cell cycle transitions. J Cell Sci 2012; 125: pp. 4703-4711
- 85. Crasta K., Lim H.H., Giddings T.H., Winey M., and Surana U.: Inactivation of Cdh1 by synergistic action of Cdk1 and polo kinase is necessary for proper assembly of the mitotic spindle. Nat Cell Biol 2008; 10: pp. 665-675
- 86. McHugh B., and Heck M.M.: Regulation of chromosome condensation and segregation. Curr Opin Genet Dev 2003; 13: pp. 185-190
- 87. Meyer H., Drozdowska A., and Dobrynin G.: A role for Cdc48/p97 and Aurora B in controlling chromatin condensation during exit from mitosis. Biochem Cell Biol 2010; 88: pp. 23-28
- 88. Gavet O., and Pines J.: Activation of cyclin B1-Cdk1 synchronizes events in the nucleus and the cytoplasm at mitosis. J Cell Biol 2010; 189: pp. 247-259
- 89. Draviam V.M., Orrechia S., Lowe M., Pardi R., and Pines J.: The localization of human cyclins B1 and B2 determines CDK1 substrate specificity and neither enzyme requires MEK to disassemble the Golgi apparatus. J Cell Biol 2001; 152: pp. 945-958
- 90. Harper J.W., Burton J.L., and Solomon M.J.: The anaphase-promoting complex: iťs not just for mitosis any more. Genes Dev 2002; 16: pp. 2179-2206
- 91. Noton E., and Diffley J.F.: CDK inactivation is the only essential function of the APC/C and the mitotic exit network proteins for origin resetting during mitosis. Mol Cell 2000; 5: pp. 85-95
- 92. Peters J.M.: The anaphase-promoting complex: proteolysis in mitosis and beyond. Mol Cell 2002; 9: pp. 931-943
- 93. Castro A., Bernis C., Vigneron S., Labbe J.C., and Lorca T.: The anaphase-promoting complex: a key factor in the regulation of cell cycle. Oncogene 2005; 24: pp. 314-325
- 94. Teixeira L.K., and Reed S.I.: Ubiquitin ligases and cell cycle control. Annu Rev Biochem 2013; 82: pp. 387-414
- 95. Mocciaro A., and Rape M.: Emerging regulatory mechanisms in ubiquitin- dependent cell cycle control. J Cell Sci 2012; 125: pp. 255-263
- 96. Russell P., and Nurse P.: [object Object]. Cell 1986; 45: pp. 781-782
- 97. Lee M.G., and Nurse P.: Cell cycle genes of the fission yeast. Sci Prog 1987; 71: pp. 1-14
- 98. Draetta G., Brizuela L., Potashkin J., and Beach D.: Identification of p34 and p13, human homologs of the cell cycle regulators of fission yeast encoded by cdc2 . Cell 1987; 50: pp. 319-325
- 99. Lee M.G., and Nurse P.: Complementation used to clone a human homologue of the fission yeast cell cycle control gene cdc2. Nature 1987; 327: pp. 31-35
- 100. Hanks S.K.: Homology probing: identification of cDNA clones encoding members of the protein-serine kinase family. Proc Natl Acad Sci U S A 1987; 84: pp. 388-392
- 101. Elledge S.J., and Spottswood M.R.: A new human p34 protein kinase, CDK2, identified by complementation of a cdc28 mutation in . EMBO J 1991; 10: pp. 2653-2659
- 102. Paris J., Le Guellec R., Couturier A., Le Guellec K., Omilli F., Camonis J., et al: Cloning by differential screening of a Xenopus cDNA coding for a protein highly homologous to cdc2. Proc Natl Acad Sci U S A 1991; 88: pp. 1039-1043
- 103. Tsai L.H., Harlow E., and Meyerson M.: Isolation of the human cdk2 gene that encodes the cyclin A- and adenovirus E1A-associated p33 kinase. Nature 1991; 353: pp. 174-177
- 104. Ninomiya-Tsuji J., Nomoto S., Yasuda H., Reed S.I., and Matsumoto K.: Cloning of a human cDNA encoding a CDC2-related kinase by complementation of a budding yeast cdc28 mutation. Proc Natl Acad Sci U S A 1991; 88: pp. 9006-9010
- 105. Matsushime H., Ewen M.E., Strom D.K., Kato J.Y., Hanks S.K., Roussel M.F., et al: Identification and properties of an atypical catalytic subunit (p34PSK-J3/cdk4) for mammalian D type G1 cyclins. Cell 1992; 71: pp. 323-334
- 106. Xiong Y., Zhang H., and Beach D.: D type cyclins associate with multiple protein kinases and the DNA replication and repair factor PCNA. Cell 1992; 71: pp. 505-514
- 107. Malumbres M., and Barbacid M.: To cycle or not to cycle: a critical decision in cancer. Nat Rev Cancer 2001; 1: pp. 222-231
- 108. Bendris N., Lemmers B., and Blanchard J.M.: Cell cycle, cytoskeleton dynamics and beyond: the many functions of cyclins and CDK inhibitors. Cell Cycle 2015; 14: pp. 1786-1798
- 109. Lolli G., and Johnson L.N.: CAK-Cyclin-dependent activating kinase: a key kinase in cell cycle control and a target for drugs? Cell Cycle 2005; 4: pp. 572-577
- 110. Kaldis P.: The cdk-activating kinase (CAK): from yeast to mammals. Cell Mol Life Sci 1999; 55: pp. 284-296
- 111. Fisher R.P.: Secrets of a double agent: CDK7 in cell-cycle control and transcription. J Cell Sci 2005; 118: pp. 5171-5180
- 112. Yang L., MacLellan W.R., Han Z., Weiss J.N., and Qu Z.: Multisite phosphorylation and network dynamics of cyclin-dependent kinase signaling in the eukaryotic cell cycle. Biophys J 2004; 86: pp. 3432-3443
- 113. Watanabe N., Broome M., and Hunter T.: Regulation of the human WEE1Hu CDK tyrosine 15-kinase during the cell cycle. EMBO J 1995; 14: pp. 1878-1891
- 114. Berry L.D., and Gould K.L.: Regulation of Cdc2 activity by phosphorylation at T14/Y15. Prog Cell Cycle Res 1996; 2: pp. 99-105
- 115. Parker L.L., and Piwnica-Worms H.: Inactivation of the p34cdc2-cyclin B complex by the human WEE1 tyrosine kinase. Science 1992; 257: pp. 1955-1957
- 116. McGowan C.H., and Russell P.: Human Wee1 kinase inhibits cell division by phosphorylating p34cdc2 exclusively on Tyr15. EMBO J 1993; 12: pp. 75-85
- 117. Fattaey A., and Booher R.N.: Myt1: a Wee1-type kinase that phosphorylates Cdc2 on residue Thr14. Prog Cell Cycle Res 1997; 3: pp. 233-240
- 118. Leise W., and Mueller P.R.: Multiple Cdk1 inhibitory kinases regulate the cell cycle during development. Dev Biol 2002; 249: pp. 156-173
- 119. Sorensen C.S., and Syljuasen R.G.: Safeguarding genome integrity: the checkpoint kinases ATR, CHK1 and WEE1 restrain CDK activity during normal DNA replication. Nucleic Acids Res 2012; 40: pp. 477-486
- 120. Aressy B., and Ducommun B.: Cell cycle control by the CDC25 phosphatases. Anti Cancer Agents Med Chem 2008; 8: pp. 818-824
- 121. Rudolph J.: Cdc25 phosphatases: structure, specificity, and mechanism. Biochemistry 2007; 46: pp. 3595-3604
- 122. Coleman T.R., and Dunphy W.G.: Cdc2 regulatory factors. Curr Opin Cell Biol 1994; 6: pp. 877-882
- 123. Lavecchia A., Di Giovanni C., and Novellino E.: CDC25 phosphatase inhibitors: an update. Mini-Rev Med Chem 2012; 12: pp. 62-73
- 124. Santamaria D., and Ortega S.: Cyclins and CDKS in development and cancer: lessons from genetically modified mice. Front Biosci 2006; 11: pp. 1164-1188
- 125. Satyanarayana A., and Kaldis P.: Mammalian cell-cycle regulation: several Cdks, numerous cyclins and diverse compensatory mechanisms. Oncogene 2009; 28: pp. 2925-2939
- 126. Aleem E., and Kaldis P.: Mouse models of cell cycle regulators: new paradigms. Results Probl Cell Differ 2006; 42: pp. 271-328
- 127. Risal S., Adhikari D., and Liu K.: Animal models for studying the in vivo functions of cell cycle CDKs. Methods Mol Biol 2016; 1336: pp. 155-166
- 128. Santamaria D., Barriere C., Cerqueira A., Hunt S., Tardy C., Newton K., et al: Cdk1 is sufficient to drive the mammalian cell cycle. Nature 2007; 448: pp. 811-815
- 129. Serrano M., Hannon G.J., and Beach D.: A new regulatory motif in cell-cycle control causing specific inhibition of cyclin D/CDK4. Nature 1993; 366: pp. 704-707
- 130. Hannon G.J., and Beach D.: p15INK4B is a potential effector of TGF-beta-induced cell cycle arrest. Nature 1994; 371: pp. 257-261
- 131. Guan K.L., Jenkins C.W., Li Y., Nichols M.A., Wu X., O’Keefe C.L., et al: Growth suppression by p18, a p16INK4/MTS1- and p14INK4B/MTS2-related CDK6 inhibitor, correlates with wild-type pRb function. Genes Dev 1994; 8: pp. 2939-2952
- 132. Hirai H., Roussel M.F., Kato J.Y., Ashmun R.A., and Sherr C.J.: Novel INK4 proteins, p19 and p18, are specific inhibitors of the cyclin D-dependent kinases CDK4 and CDK6. Mol Cell Biol 1995; 15: pp. 2672-2681
- 133. Chan F.K., Zhang J., Cheng L., Shapiro D.N., and Winoto A.: Identification of human and mouse p19, a novel CDK4 and CDK6 inhibitor with homology to p16ink4. Mol Cell Biol 1995; 15: pp. 2682-2688
- 134. Sherr C.J., and Roberts J.M.: Inhibitors of mammalian G1 cyclin-dependent kinases. Genes Dev 1995; 9: pp. 1149-1163
- 135. Pavletich N.P.: Mechanisms of cyclin-dependent kinase regulation: structures of Cdks, their cyclin activators, and Cip and INK4 inhibitors. J Mol Biol 1999; 287: pp. 821-828
- 136. Gu Y., Turck C.W., and Morgan D.O.: Inhibition of CDK2 activity in vivo by an associated 20K regulatory subunit. Nature 1993; 366: pp. 707-710
- 137. Harper J.W., Adami G.R., Wei N., Keyomarsi K., and Elledge S.J.: The p21 Cdk-interacting protein Cip1 is a potent inhibitor of G1 cyclin-dependent kinases. Cell 1993; 75: pp. 805-816
- 138. el-Deiry W.S., Tokino T., Velculescu V.E., Levy D.B., Parsons R., Trent J.M., et al: WAF1, a potential mediator of p53 tumor suppression. Cell 1993; 75: pp. 817-825
- 139. Xiong Y., Hannon G.J., Zhang H., Casso D., Kobayashi R., and Beach D.: p21 is a universal inhibitor of cyclin kinases. Nature 1993; 366: pp. 701-704
- 140. Dulic V., Kaufmann W.K., Wilson S.J., Tlsty T.D., Lees E., Harper J.W., et al: p53-dependent inhibition of cyclin-dependent kinase activities in human fibroblasts during radiation-induced G1 arrest. Cell 1994; 76: pp. 1013-1023
- 141. Noda A., Ning Y., Venable S.F., Pereira-Smith O.M., and Smith J.R.: Cloning of senescent cell-derived inhibitors of DNA synthesis using an expression screen. Exp Cell Res 1994; 211: pp. 90-98
- 142. Polyak K., Lee M.H., Erdjument-Bromage H., Koff A., Roberts J.M., Tempst P., et al: Cloning of p27Kip1, a cyclin-dependent kinase inhibitor and a potential mediator of extracellular antimitogenic signals. Cell 1994; 78: pp. 59-66
- 143. Polyak K., Kato J.Y., Solomon M.J., Sherr C.J., Massague J., Roberts J.M., et al: p27Kip1, a cyclin-Cdk inhibitor, links transforming growth factor-beta and contact inhibition to cell cycle arrest. Genes Dev 1994; 8: pp. 9-22
- 144. Toyoshima H., and Hunter T.: p27, a novel inhibitor of G1 cyclin-Cdk protein kinase activity, is related to p21. Cell 1994; 78: pp. 67-74
- 145. Lee M.H., Reynisdottir I., and Massague J.: Cloning of p57KIP2, a cyclin-dependent kinase inhibitor with unique domain structure and tissue distribution. Genes Dev 1995; 9: pp. 639-649
- 146. Matsuoka S., Edwards M.C., Bai C., Parker S., Zhang P., Baldini A., et al: p57KIP2, a structurally distinct member of the p21CIP1 Cdk inhibitor family, is a candidate tumor suppressor gene. Genes Dev 1995; 9: pp. 650-662
- 147. Chen J., Jackson P.K., Kirschner M.W., and Dutta A.: Separate domains of p21 involved in the inhibition of Cdk kinase and PCNA. Nature 1995; 374: pp. 386-388
- 148. Chen J., Saha P., Kornbluth S., Dynlacht B.D., and Dutta A.: Cyclin-binding motifs are essential for the function of p21CIP1. Mol Cell Biol 1996; 16: pp. 4673-4682
- 149. Nakanishi M., Robetorye R.S., Adami G.R., Pereira-Smith O.M., and Smith J.R.: Identification of the active region of the DNA synthesis inhibitory gene p21Sdi1/CIP1/WAF1. EMBO J 1995; 14: pp. 555-563
- 150. Warbrick E., Lane D.P., Glover D.M., and Cox L.S.: A small peptide inhibitor of DNA replication defines the site of interaction between the cyclin-dependent kinase inhibitor p21WAF1 and proliferating cell nuclear antigen. Curr Biol 1995; 5: pp. 275-282
- 151. Lin J., Reichner C., Wu X., and Levine A.J.: Analysis of wild-type and mutant p21WAF-1 gene activities. Mol Cell Biol 1996; 16: pp. 1786-1793
- 152. Russo A.A., Jeffrey P.D., Patten A.K., Massague J., and Pavletich N.P.: Crystal structure of the p27Kip1 cyclin-dependent-kinase inhibitor bound to the cyclin A-Cdk2 complex. Nature 1996; 382: pp. 325-331
- 153. Elledge S.J.: Cell cycle checkpoints: preventing an identity crisis. Science 1996; 274: pp. 1664-1672
- 154. Murray A.: Cell cycle checkpoints. Curr Opin Cell Biol 1994; 6: pp. 872-876
- 155. Pietenpol J.A., and Stewart Z.A.: Cell cycle checkpoint signaling: cell cycle arrest versus apoptosis. Toxicology 2002; 181–182: pp. 475-481
- 156. Xie S., Xie B., Lee M.Y., and Dai W.: Regulation of cell cycle checkpoints by polo-like kinases. Oncogene 2005; 24: pp. 277-286
- 157. O’Connor P.M.: Mammalian G1 and G2 phase checkpoints. Cancer Surv 1997; 29: pp. 151-182
- 158. Donjerkovic D., and Scott D.W.: Regulation of the G1 phase of the mammalian cell cycle. Cell Res 2000; 10: pp. 1-16
- 159. Malumbres M.: Revisiting the “Cdk-centric” view of the mammalian cell cycle. Cell Cycle 2005; 4: pp. 206-210
- 160. Holland A.J., and Cleveland D.W.: Boveri revisited: chromosomal instability, aneuploidy and tumorigenesis. Nat Rev Mol Cell Biol 2009; 10: pp. 478-487
- 161. Yen T.J., and Kao G.D.: Mitotic checkpoint, aneuploidy and cancer. Adv Exp Med Biol 2005; 570: pp. 477-499
- 162. Qi W., and Yu H.: The spindle checkpoint and chromosomal stability. Genome Dyn 2006; 1: pp. 116-130
- 163. Kops G.J.: The kinetochore and spindle checkpoint in mammals. Front Biosci 2008; 13: pp. 3606-3620
- 164. Suijkerbuijk S.J., and Kops G.J.: Preventing aneuploidy: the contribution of mitotic checkpoint proteins. Biochim Biophys Acta 2008; 1786: pp. 24-31
- 165. Sczaniecka M.M., and Hardwick K.G.: The spindle checkpoint: how do cells delay anaphase onset? SEB Exp Biol Ser 2008; 59: pp. 243-256
- 166. Decordier I., Cundari E., and Kirsch-Volders M.: Mitotic checkpoints and the maintenance of the chromosome karyotype. Mutat Res 2008; 651: pp. 3-13
- 167. Musacchio A., and Salmon E.D.: The spindle-assembly checkpoint in space and time. Nat Rev Mol Cell Biol 2007; 8: pp. 379-393
- 168. May K.M., and Hardwick K.G.: The spindle checkpoint. J Cell Sci 2006; 119: pp. 4139-4142
- 169. Hartwell L.H., and Weinert T.A.: Checkpoints: controls that ensure the order of cell cycle events. Science 1989; 246: pp. 629-634
- 170. Lukas J., Parry D., Aagaard L., Mann D.J., Bartkova J., Strauss M., et al: Retinoblastoma-protein-dependent cell-cycle inhibition by the tumour suppressor p16. Nature 1995; 375: pp. 503-506
- 171. Medema R.H., Herrera R.E., Lam F., and Weinberg R.A.: Growth suppression by p16ink4 requires functional retinoblastoma protein. Proc Natl Acad Sci U S A 1995; 92: pp. 6289-6293
- 172. Blain S.W., Montalvo E., and Massague J.: Differential interaction of the cyclin-dependent kinase (Cdk) inhibitor p27Kip1 with cyclin A-Cdk2 and cyclin D2-Cdk4. J Biol Chem 1997; 272: pp. 25863-25872
- 173. LaBaer J., Garrett M.D., Stevenson L.F., Slingerland J.M., Sandhu C., Chou H.S., et al: New functional activities for the p21 family of CDK inhibitors. Genes Dev 1997; 11: pp. 847-862
- 174. Cheng M., Olivier P., Diehl J.A., Fero M., Roussel M.F., Roberts J.M., et al: The p21(Cip1) and p27(Kip1) CDK ‘inhibitors’ are essential activators of cyclin D-dependent kinases in murine fibroblasts. EMBO J 1999; 18: pp. 1571-1583
- 175. Roussel M.F.: The INK4 family of cell cycle inhibitors in cancer. Oncogene 1999; 18: pp. 5311-5317
- 176. Schmitt E., Paquet C., Beauchemin M., and Bertrand R.: DNA-damage response network at the crossroads of cell-cycle checkpoints, cellular senescence and apoptosis. J Zhejiang Univ Sci B 2007; 8: pp. 377-397
- 177. Sancar A., Lindsey-Boltz L.A., Unsal-Kacmaz K., and Linn S.: Molecular mechanisms of mammalian DNA repair and the DNA damage checkpoints. Annu Rev Biochem 2004; 73: pp. 39-85
- 178. Motoyama N., and Naka K.: DNA damage tumor suppressor genes and genomic instability. Curr Opin Genet Dev 2004; 14: pp. 11-16
- 179. Bartek J., and Lukas J.: DNA damage checkpoints: from initiation to recovery or adaptation. Curr Opin Cell Biol 2007; 19: pp. 238-245
- 180. Ishikawa K., Ishii H., and Saito T.: DNA damage-dependent cell cycle checkpoints and genomic stability. DNA Cell Biol 2006; 25: pp. 406-411
- 181. Niida H., and Nakanishi M.: DNA damage checkpoints in mammals. Mutagenesis 2006; 21: pp. 3-9
- 182. Nojima H.: Protein kinases that regulate chromosome stability and their downstream targets. Genome Dyn 2006; 1: pp. 131-148
- 183. Cimprich K.A., and Cortez D.: ATR: an essential regulator of genome integrity. Nat Rev Mol Cell Biol 2008; 9: pp. 616-627
- 184. Lavin M.F., and Kozlov S.: ATM activation and DNA damage response. Cell Cycle 2007; 6: pp. 931-942
- 185. Paulsen R.D., and Cimprich K.A.: The ATR pathway: fine-tuning the fork. DNA Repair (Amst) 2007; 6: pp. 953-966
- 186. Hurley P.J., and Bunz F.: ATM and ATR: components of an integrated circuit. Cell Cycle 2007; 6: pp. 414-417
- 187. Awasthi P., Foiani M., and Kumar A.: ATM and ATR signaling at a glance. J Cell Sci 2015; 128: pp. 4255-4262
- 188. Marechal A., and Zou L.: DNA damage sensing by the ATM and ATR kinases. Cold Spring Harb Perspect Biol 2013; 5:
- 189. Falck J., Mailand N., Syljuasen R.G., Bartek J., and Lukas J.: The ATM-Chk2-Cdc25A checkpoint pathway guards against radioresistant DNA synthesis. Nature 2001; 410: pp. 842-847
- 190. Bartek J., and Lukas J.: Chk1 and Chk2 kinases in checkpoint control and cancer. Cancer Cell 2003; 3: pp. 421-429
- 191. Donzelli M., and Draetta G.F.: Regulating mammalian checkpoints through Cdc25 inactivation. EMBO Rep 2003; 4: pp. 671-677
- 192. Lempiainen H., and Halazonetis T.D.: Emerging common themes in regulation of PIKKs and PI3Ks. EMBO J 2009; 28: pp. 3067-3073
- 193. Lovejoy C.A., and Cortez D.: Common mechanisms of PIKK regulation. DNA Repair (Amst) 2009; 8: pp. 1004-1008
- 194. Matsuoka S., Ballif B.A., Smogorzewska A., ER M.D., Hurov K.E., Luo J., et al: ATM and ATR substrate analysis reveals extensive protein networks responsive to DNA damage. Science 2007; 316: pp. 1160-1166
- 195. Smolka M.B., Albuquerque C.P., Chen S.H., and Zhou H.: Proteome-wide identification of in vivo targets of DNA damage checkpoint kinases. Proc Natl Acad Sci U S A 2007; 104: pp. 10364-10369
- 196. Bensimon A., Schmidt A., Ziv Y., Elkon R., Wang S.Y., Chen D.J., et al: ATM-dependent and -independent dynamics of the nuclear phosphoproteome after DNA damage. Sci Signal 2010; 3: pp. rs3
- 197. Beli P., Lukashchuk N., Wagner S.A., Weinert B.T., Olsen J.V., Baskcomb L., et al: Proteomic investigations reveal a role for RNA processing factor THRAP3 in the DNA damage response. Mol Cell 2012; 46: pp. 212-225
- 198. Farnebo M., Bykov V.J., and Wiman K.G.: The p53 tumor suppressor: a master regulator of diverse cellular processes and therapeutic target in cancer. Biochem Biophys Res Commun 2010; 396: pp. 85-89
- 199. Boehme K.A., and Blattner C.: Regulation of p53–insights into a complex process. Crit Rev Biochem Mol Biol 2009; 44: pp. 367-392
- 200. Meek D.W.: Tumour suppression by p53: a role for the DNA damage response? Nat Rev Cancer 2009; 9: pp. 714-723
- 201. Vazquez A., Bond E.E., Levine A.J., and Bond G.L.: The genetics of the p53 pathway, apoptosis and cancer therapy. Nat Rev Drug Discov 2008; 7: pp. 979-987
- 202. Viadiu H.: Molecular architecture of tumor suppressor p53. Curr Top Med Chem 2008; 8: pp. 1327-1334
- 203. Varley J.: TP53, hChk2, and the Li-Fraumeni syndrome. Methods Mol Biol 2003; 222: pp. 117-129
- 204. Schwartz D., and Rotter V.: p53-dependent cell cycle control: response to genotoxic stress. Semin Cancer Biol 1998; 8: pp. 325-336
- 205. Shieh S.Y., Ikeda M., Taya Y., and Prives C.: DNA damage-induced phosphorylation of p53 alleviates inhibition by MDM2. Cell 1997; 91: pp. 325-334
- 206. Agami R., and Bernards R.: Distinct initiation and maintenance mechanisms cooperate to induce G1 cell cycle arrest in response to DNA damage. Cell 2000; 102: pp. 55-66
- 207. Xu B., Kim S.T., Lim D.S., and Kastan M.B.: Two molecularly distinct G(2)/M checkpoints are induced by ionizing irradiation. Mol Cell Biol 2002; 22: pp. 1049-1059
- 208. Nyberg K.A., Michelson R.J., Putnam C.W., and Weinert T.A.: Toward maintaining the genome: DNA damage and replication checkpoints. Annu Rev Genet 2002; 36: pp. 617-656
- 209. Mailand N., Podtelejnikov A.V., Groth A., Mann M., Bartek J., and Lukas J.: Regulation of G(2)/M events by Cdc25A through phosphorylation-dependent modulation of its stability. EMBO J 2002; 21: pp. 5911-5920
- 210. Taylor W.R., and Stark G.R.: Regulation of the G2/M transition by p53. Oncogene 2001; 20: pp. 1803-1815
- 211. Grallert B., and Boye E.: The multiple facets of the intra-S checkpoint. Cell Cycle 2008; 7: pp. 2315-2320
- 212. Bartek J., Lukas C., and Lukas J.: Checking on DNA damage in S phase. Nat Rev Mol Cell Biol 2004; 5: pp. 792-804
- 213. Canman C.E.: Replication checkpoint: preventing mitotic catastrophe. Curr Biol 2001; 11: pp. R121-R124
- 214. Friedel A.M., Pike B.L., and Gasser S.M.: ATR/Mec1: coordinating fork stability and repair. Curr Opin Cell Biol 2009; 21: pp. 237-244
- 215. Segurado M., and Tercero J.A.: The S-phase checkpoint: targeting the replication fork. Biol Cell 2009; 101: pp. 617-627
- 216. Bartek J., and Lukas J.: Mammalian G1- and S-phase checkpoints in response to DNA damage. Curr Opin Cell Biol 2001; 13: pp. 738-747
- 217. Shiloh Y.: ATM and related protein kinases: safeguarding genome integrity. Nat Rev Cancer 2003; 3: pp. 155-168
- 218. Bakkenist C.J., and Kastan M.B.: DNA damage activates ATM through intermolecular autophosphorylation and dimer dissociation. Nature 2003; 421: pp. 499-506
- 219. Zou L., and Elledge S.J.: Sensing DNA damage through ATRIP recognition of RPA-ssDNA complexes. Science 2003; 300: pp. 1542-1548
- 220. Helt C.E., Cliby W.A., Keng P.C., Bambara R.A., and O’Reilly M.A.: Ataxia telangiectasia mutated (ATM) and ATM and Rad3-related protein exhibit selective target specificities in response to different forms of DNA damage. J Biol Chem 2005; 280: pp. 1186-1192
- 221. Heffernan T.P., Simpson D.A., Frank A.R., Heinloth A.N., Paules R.S., Cordeiro-Stone M., et al: An ATR- and Chk1-dependent S checkpoint inhibits replicon initiation following UVC-induced DNA damage. Mol Cell Biol 2002; 22: pp. 8552-8561
- 222. Gatei M., Sloper K., Sorensen C., Syljuasen R., Falck J., Hobson K., et al: Ataxia-telangiectasia-mutated (ATM) and NBS1-dependent phosphorylation of Chk1 on Ser-317 in response to ionizing radiation. J Biol Chem 2003; 278: pp. 14806-14811
- 223. Wang X.Q., Redpath J.L., Fan S.T., and Stanbridge E.J.: ATR dependent activation of Chk2. J Cell Physiol 2006; 208: pp. 613-619
- 224. Broderick R., and Nasheuer H.P.: Regulation of Cdc45 in the cell cycle and after DNA damage. Biochem Soc Trans 2009; 37: pp. 926-930
- 225. Kitagawa R., Bakkenist C.J., McKinnon P.J., and Kastan M.B.: Phosphorylation of SMC1 is a critical downstream event in the ATM-NBS1-BRCA1 pathway. Genes Dev 2004; 18: pp. 1423-1438
- 226. Kim S.T., Xu B., and Kastan M.B.: Involvement of the cohesin protein, Smc1, in Atm-dependent and independent responses to DNA damage. Genes Dev 2002; 16: pp. 560-570
- 227. Yazdi P.T., Wang Y., Zhao S., Patel N., Lee E.Y., and Qin J.: SMC1 is a downstream effector in the ATM/NBS1 branch of the human S-phase checkpoint. Genes Dev 2002; 16: pp. 571-582
- 228. Kops G.J., Weaver B.A., and Cleveland D.W.: On the road to cancer: aneuploidy and the mitotic checkpoint. Nat Rev Cancer 2005; 5: pp. 773-785
- 229. Rieder C.L., and Maiato H.: Stuck in division or passing through: what happens when cells cannot satisfy the spindle assembly checkpoint. Dev Cell 2004; 7: pp. 637-651
- 230. Malmanche N., Maia A., and Sunkel C.E.: The spindle assembly checkpoint: preventing chromosome mis-segregation during mitosis and meiosis. FEBS Lett 2006; 580: pp. 2888-2895
- 231. Shah J.V., and Cleveland D.W.: Waiting for anaphase: Mad2 and the spindle assembly checkpoint. Cell 2000; 103: pp. 997-1000
- 232. Sacristan C., and Kops G.J.: Joined at the hip: kinetochores, microtubules, and spindle assembly checkpoint signaling. Trends Cell Biol 2015; 25: pp. 21-28
- 233. Wang Y., Jin F., Higgins R., and McKnight K.: The current view for the silencing of the spindle assembly checkpoint. Cell Cycle 2014; 13: pp. 1694-1701
- 234. Hoyt M.A., Totis L., and Roberts B.T.: S. cerevisiae genes required for cell cycle arrest in response to loss of microtubule function. Cell 1991; 66: pp. 507-517
- 235. Li R., and Murray A.W.: Feedback control of mitosis in budding yeast. Cell 1991; 66: pp. 519-531
- 236. Musacchio A., and Hardwick K.G.: The spindle checkpoint: structural insights into dynamic signalling. Nat Rev Mol Cell Biol 2002; 3: pp. 731-741
- 237. Taylor S.S., Scott M.I., and Holland A.J.: The spindle checkpoint: a quality control mechanism which ensures accurate chromosome segregation. Chromosom Res 2004; 12: pp. 599-616
- 238. London N., and Biggins S.: Signalling dynamics in the spindle checkpoint response. Nat Rev Mol Cell Biol 2014; 15: pp. 736-747
- 239. Joglekar A.P.: A cell biological perspective on past, present and future investigations of the spindle assembly checkpoint. Biology 2016; 5:
- 240. Lischetti T., and Nilsson J.: Regulation of mitotic progression by the spindle assembly checkpoint. Mol Cell Oncol 2015; 2:
- 241. Kim S.H., Lin D.P., Matsumoto S., Kitazono A., and Matsumoto T.: Fission yeast Slp1: an effector of the Mad2-dependent spindle checkpoint. Science 1998; 279: pp. 1045-1047
- 242. Hwang L.H., Lau L.F., Smith D.L., Mistrot C.A., Hardwick K.G., Hwang E.S., et al: Budding yeast Cdc20: a target of the spindle checkpoint. Science 1998; 279: pp. 1041-1044
- 243. Simpson-Lavy K.J., Oren Y.S., Feine O., Sajman J., Listovsky T., and Brandeis M.: Fifteen years of APC/cyclosome: a short and impressive biography. Biochem Soc Trans 2010; 38: pp. 78-82
- 244. Wasch R., Robbins J.A., and Cross F.R.: The emerging role of APC/CCdh1 in controlling differentiation, genomic stability and tumor suppression. Oncogene 2010; 29: pp. 1-10
- 245. Pesin J.A., and Orr-Weaver T.L.: Regulation of APC/C activators in mitosis and meiosis. Annu Rev Cell Dev Biol 2008; 24: pp. 475-499
- 246. Lindon C.: Control of mitotic exit and cytokinesis by the APC/C. Biochem Soc Trans 2008; 36: pp. 405-410
- 247. Baker D.J., Dawlaty M.M., Galardy P., and van Deursen J.M.: Mitotic regulation of the anaphase-promoting complex. Cell Mol Life Sci 2007; 64: pp. 589-600
- 248. Peters J.M.: The anaphase promoting complex/cyclosome: a machine designed to destroy. Nat Rev Mol Cell Biol 2006; 7: pp. 644-656
- 249. Acquaviva C., and Pines J.: The anaphase-promoting complex/cyclosome: APC/C. J Cell Sci 2006; 119: pp. 2401-2404
- 250. Vanoosthuyse V., and Hardwick K.G.: Bub1 and the multilayered inhibition of Cdc20-APC/C in mitosis. Trends Cell Biol 2005; 15: pp. 231-233
- 251. Vodermaier H.C.: APC/C and SCF: controlling each other and the cell cycle. Curr Biol 2004; 14: pp. R787-R796
- 252. Meadows J.C., and Millar J.B.: Sharpening the anaphase switch. Biochem Soc Trans 2015; 43: pp. 19-22
- 253. Sivakumar S., and Gorbsky G.J.: Spatiotemporal regulation of the anaphase-promoting complex in mitosis. Nat Rev Mol Cell Biol 2015; 16: pp. 82-94
- 254. Hershko A.: Mechanisms and regulation of the degradation of cyclin B. Philos Trans R Soc Lond Ser B Biol Sci 1999; 354: pp. 1571-1575
- 255. Wolf F., Sigl R., and Geley S.: ‘…The end of the beginning’: cdk1 thresholds and exit from mitosis. Cell Cycle 2007; 6: pp. 1408-1411
- 256. Irniger S.: Cyclin destruction in mitosis: a crucial task of Cdc20. FEBS Lett 2002; 532: pp. 7-11
- 257. John P.C., Mews M., and Moore R.: Cyclin/Cdk complexes: their involvement in cell cycle progression and mitotic division. Protoplasma 2001; 216: pp. 119-142
- 258. Stemmann O., Gorr I.H., and Boos D.: Anaphase topsy-turvy: Cdk1 a securin, separase a CKI. Cell Cycle 2006; 5: pp. 11-13
- 259. Yanagida M.: Cell cycle mechanisms of sister chromatid separation; roles of Cut1/separin and Cut2/securin. Genes Cells 2000; 5: pp. 1-8
- 260. Nagao K., and Yanagida M.: Regulating sister chromatid separation by separase phosphorylation. Dev Cell 2002; 2: pp. 2-4
- 261. Uhlmann F.: Chromosome cohesion and separation: from men and molecules. Curr Biol 2003; 13: pp. R104-R114
- 262. Pellman D., and Christman M.F.: Separase anxiety: dissolving the sister bond and more. Nat Cell Biol 2001; 3: pp. E207-E209
- 263. Agarwal R., and Cohen-Fix O.: Mitotic regulation: the fine tuning of separase activity. Cell Cycle 2002; 1: pp. 255-257
- 264. Uhlmann F.: Separase regulation during mitosis. Biochem Soc Symp 2003; 70: pp. 243-251
- 265. Hydbring P., Malumbres M., and Sicinski P.: Non-canonical functions of cell cycle cyclins and cyclin-dependent kinases. Nat Rev Mol Cell Biol 2016; 17: pp. 280-292
- 266. Lim S., and Kaldis P.: Cdks, cyclins and CKIs: roles beyond cell cycle regulation. Development 2013; 140: pp. 3079-3093
- 267. Fujimoto T., Anderson K., Jacobsen S.E., Nishikawa S.I., and Nerlov C.: Cdk6 blocks myeloid differentiation by interfering with Runx1 DNA binding and Runx1-C/EBPalpha interaction. EMBO J 2007; 26: pp. 2361-2370
- 268. Kollmann K., Heller G., Schneckenleithner C., Warsch W., Scheicher R., Ott R.G., et al: A kinase-independent function of CDK6 links the cell cycle to tumor angiogenesis. Cancer Cell 2013; 24: pp. 167-181
- 269. Le Guen T., Ragu S., Guirouilh-Barbat J., and Lopez B.S.: Role of the double-strand break repair pathway in the maintenance of genomic stability. Mol Cell Oncol 2015; 2:
- 270. Jirawatnotai S., Hu Y., Michowski W., Elias J.E., Becks L., Bienvenu F., et al: A function for cyclin D1 in DNA repair uncovered by protein interactome analyses in human cancers. Nature 2011; 474: pp. 230-234
- 271. Chen L., Nievera C.J., Lee A.Y., and Wu X.: Cell cycle-dependent complex formation of BRCA1.CtIP.MRN is important for DNA double-strand break repair. J Biol Chem 2008; 283: pp. 7713-7720
- 272. Hustedt N., and Durocher D.: The control of DNA repair by the cell cycle. Nat Cell Biol 2016; 19: pp. 1-9
- 273. Starostina N.G., and Kipreos E.T.: Multiple degradation pathways regulate versatile CIP/KIP CDK inhibitors. Trends Cell Biol 2012; 22: pp. 33-41
- 274. Abbas T., and Dutta A.: p21 in cancer: intricate networks and multiple activities. Nat Rev Cancer 2009; 9: pp. 400-414
- 275. Huang S., Shu L., Dilling M.B., Easton J., Harwood F.C., Ichijo H., et al: Sustained activation of the JNK cascade and rapamycin-induced apoptosis are suppressed by p53/p21(Cip1). Mol Cell 2003; 11: pp. 1491-1501
- 276. Dotto G.P.: p21(WAF1/Cip1): more than a break to the cell cycle? Biochim Biophys Acta 2000; 1471: pp. M43-M56
- 277. Narumiya S., Tanji M., and Ishizaki T.: Rho signaling, ROCK and mDia1, in transformation, metastasis and invasion. Cancer Metastasis Rev 2009; 28: pp. 65-76
- 278. Bernstein B.W., and Bamburg J.R.: ADF/cofilin: a functional node in cell biology. Trends Cell Biol 2010; 20: pp. 187-195
- 279. Wood L.D., Parsons D.W., Jones S., Lin J., Sjoblom T., Leary R.J., et al: The genomic landscapes of human breast and colorectal cancers. Science 2007; 318: pp. 1108-1113
- 280. Sjoblom T., Jones S., Wood L.D., Parsons D.W., Lin J., Barber T.D., et al: The consensus coding sequences of human breast and colorectal cancers. Science 2006; 314: pp. 268-274
- 281. Vogelstein B., Papadopoulos N., Velculescu V.E., Zhou S., Diaz L.A., and Kinzler K.W.: Cancer genome landscapes. Science 2013; 339: pp. 1546-1558
- 282. Copeland N.G., and Jenkins N.A.: Deciphering the genetic landscape of cancer—from genes to pathways. Trends Genet 2009; 25: pp. 455-462
- 283. Schweiger M.R., Hussong M., Rohr C., and Lehrach H.: Genomics and epigenomics of colorectal cancer. Wiley Interdiscip Rev Syst Biol Med 2013; 5: pp. 205-219
- 284. Beijersbergen R.L., and Bernards R.: Cell cycle regulation by the retinoblastoma family of growth inhibitory proteins. Biochim Biophys Acta 1996; 1287: pp. 103-120
- 285. Kaelin W.G.: Recent insights into the functions of the retinoblastoma susceptibility gene product. Cancer Investig 1997; 15: pp. 243-254
- 286. Sellers W.R., and Kaelin W.G.: Role of the retinoblastoma protein in the pathogenesis of human cancer. J Clin Oncol 1997; 15: pp. 3301-3312
- 287. Nevins J.R.: The Rb/E2F pathway and cancer. Hum Mol Genet 2001; 10: pp. 699-703
- 288. Hahn W.C., and Weinberg R.A.: Modelling the molecular circuitry of cancer. Nat Rev Cancer 2002; 2: pp. 331-341
- 289. Ortega S., Malumbres M., and Barbacid M.: Cyclin D-dependent kinases, INK4 inhibitors and cancer. Biochim Biophys Acta 2002; 1602: pp. 73-87
- 290. Sherr C.J., and McCormick F.: The RB and p53 pathways in cancer. Cancer Cell 2002; 2: pp. 103-112
- 291. Sherr C.J.: The INK4a/ARF network in tumour suppression. Nat Rev Mol Cell Biol 2001; 2: pp. 731-737
- 292. Wikenheiser-Brokamp K.A.: Retinoblastoma family proteins: insights gained through genetic manipulation of mice. Cell Mol Life Sci 2006; 63: pp. 767-780
- 293. Dyson N.J.: RB1: a prototype tumor suppressor and an enigma. Genes Dev 2016; 30: pp. 1492-1502
- 294. Indovina P., Pentimalli F., Casini N., Vocca I., and Giordano A.: RB1 dual role in proliferation and apoptosis: cell fate control and implications for cancer therapy. Oncotarget 2015; 6: pp. 17873-17890
- 295. Dick F.A., and Rubin S.M.: Molecular mechanisms underlying RB protein function. Nat Rev Mol Cell Biol 2013; 14: pp. 297-306
- 296. Kamb A., Shattuck-Eidens D., Eeles R., Liu Q., Gruis N.A., Ding W., et al: Analysis of the p16 gene (CDKN2) as a candidate for the chromosome 9p melanoma susceptibility locus. Nat Genet 1994; 8: pp. 23-26
- 297. Ruas M., and Peters G.: The p16INK4a/CDKN2A tumor suppressor and its relatives. Biochim Biophys Acta 1998; 1378: pp. F115-F177
- 298. Li J., Poi M.J., and Tsai M.D.: Regulatory mechanisms of tumor suppressor P16(INK4A) and their relevance to cancer. Biochemistry 2011; 50: pp. 5566-5582
- 299. LaPak K.M., and Burd C.E.: The molecular balancing act of p16(INK4a) in cancer and aging. Mol Cancer Res 2014; 12: pp. 167-183
- 300. Johnson J., Thijssen B., McDermott U., Garnett M., Wessels L.F., and Bernards R.: Targeting the RB-E2F pathway in breast cancer. Oncogene 2016; 35: pp. 4829-4835
- 301. Hutcheson J., Witkiewicz A.K., and Knudsen E.S.: The RB tumor suppressor at the intersection of proliferation and immunity: relevance to disease immune evasion and immunotherapy. Cell Cycle 2015; 14: pp. 3812-3819
- 302. Radulescu R.T.: From the RB tumor suppressor to MCR peptides. Protein Pept Lett 2014; 21: pp. 589-592
- 303. Levine A.J.: p53, the cellular gatekeeper for growth and division. Cell 1997; 88: pp. 323-331
- 304. Hollstein M., Rice K., Greenblatt M.S., Soussi T., Fuchs R., Sorlie T., et al: Database of p53 gene somatic mutations in human tumors and cell lines. Nucleic Acids Res 1994; 22: pp. 3551-3555
- 305. Giaccia A.J., and Kastan M.B.: The complexity of p53 modulation: emerging patterns from divergent signals. Genes Dev 1998; 12: pp. 2973-2983
- 306. Gottifredi V., Shieh S.Y., and Prives C.: Regulation of p53 after different forms of stress and at different cell cycle stages. Cold Spring Harb Symp Quant Biol 2000; 65: pp. 483-488
- 307. Helton E.S., and Chen X.: p53 modulation of the DNA damage response. J Cell Biochem 2007; 100: pp. 883-896
- 308. Williams A.B., and Schumacher B.: p53 in the DNA-damage-repair process. Cold Spring Harb Perspect Med 2016; 6:
- 309. Speidel D.: The role of DNA damage responses in p53 biology. Arch Toxicol 2015; 89: pp. 501-517
- 310. Jackson S.P., and Bartek J.: The DNA-damage response in human biology and disease. Nature 2009; 461: pp. 1071-1078
- 311. Bieging K.T., Mello S.S., and Attardi L.D.: Unravelling mechanisms of p53-mediated tumour suppression. Nat Rev Cancer 2014; 14: pp. 359-370
- 312. Sionov R.V., and Haupt Y.: The cellular response to p53: the decision between life and death. Oncogene 1999; 18: pp. 6145-6157
- 313. Bates S., and Vousden K.H.: Mechanisms of p53-mediated apoptosis. Cell Mol Life Sci 1999; 55: pp. 28-37
- 314. Corn P.G., and El-Deiry W.S.: Microarray analysis of p53-dependent gene expression in response to hypoxia and DNA damage. Cancer Biol Ther 2007; 6: pp. 1858-1866
- 315. Riley T., Sontag E., Chen P., and Levine A.: Transcriptional control of human p53-regulated genes. Nat Rev Mol Cell Biol 2008; 9: pp. 402-412
- 316. Horvath M.M., Wang X., Resnick M.A., and Bell D.A.: Divergent evolution of human p53 binding sites: cell cycle versus apoptosis. PLoS Genet 2007; 3:
- 317. Jen K.Y., and Cheung V.G.: Identification of novel p53 target genes in ionizing radiation response. Cancer Res 2005; 65: pp. 7666-7673
- 318. Mirza A., Wu Q., Wang L., McClanahan T., Bishop W.R., Gheyas F., et al: Global transcriptional program of p53 target genes during the process of apoptosis and cell cycle progression. Oncogene 2003; 22: pp. 3645-3654
- 319. Rashi-Elkeles S., Elkon R., Shavit S., Lerenthal Y., Linhart C., Kupershtein A., et al: Transcriptional modulation induced by ionizing radiation: p53 remains a central player. Mol Oncol 2011; 5: pp. 336-348
- 320. Beckerman R., and Prives C.: Transcriptional regulation by p53. Cold Spring Harb Perspect Biol 2010; 2:
- 321. McCubrey J.A., Lertpiriyapong K., Fitzgerald T.L., Martelli A.M., Cocco L., Rakus D., et al: Roles of TP53 in determining therapeutic sensitivity, growth, cellular senescence, invasion and metastasis. Adv Biol Regul 2017; 63: pp. 32-48
- 322. Chipuk J.E., Kuwana T., Bouchier-Hayes L., Droin N.M., Newmeyer D.D., Schuler M., et al: Direct activation of Bax by p53 mediates mitochondrial membrane permeabilization and apoptosis. Science 2004; 303: pp. 1010-1014
- 323. Oltvai Z.N., Milliman C.L., and Korsmeyer S.J.: Bcl-2 heterodimerizes in vivo with a conserved homolog, Bax, that accelerates programmed cell death. Cell 1993; 74: pp. 609-619
- 324. Luna-Vargas M.P., and Chipuk J.E.: Physiological and Pharmacological Control of BAK, BAX, and Beyond. Trends Cell Biol 2016; 26: pp. 906-917
- 325. Renault T.T., Teijido O., Antonsson B., Dejean L.M., and Manon S.: Regulation of Bax mitochondrial localization by Bcl-2 and Bcl-x(L): keep your friends close but your enemies closer. Int J Biochem Cell Biol 2013; 45: pp. 64-67
- 326. Juven-Gershon T., and Oren M.: Mdm2: the ups and downs. Mol Med 1999; 5: pp. 71-83
- 327. Zhang Y., and Xiong Y.: Control of p53 ubiquitination and nuclear export by MDM2 and ARF. Cell Growth Differ 2001; 12: pp. 175-186
- 328. Michael D., and Oren M.: The p53-Mdm2 module and the ubiquitin system. Semin Cancer Biol 2003; 13: pp. 49-58
- 329. Prives C.: Signaling to p53: breaking the MDM2-p53 circuit. Cell 1998; 95: pp. 5-8
- 330. Piette J., Neel H., and Marechal V.: Mdm2: keeping p53 under control. Oncogene 1997; 15: pp. 1001-1010
- 331. Lowe S.W., and Sherr C.J.: Tumor suppression by Ink4a-Arf: progress and puzzles. Curr Opin Genet Dev 2003; 13: pp. 77-83
- 332. James M.C., and Peters G.: Alternative product of the p16/CKDN2A locus connects the Rb and p53 tumor suppressors. Prog Cell Cycle Res 2000; 4: pp. 71-81
- 333. Serrano M.: The INK4a/ARF locus in murine tumorigenesis. Carcinogenesis 2000; 21: pp. 865-869
- 334. Sharpless N.E.: INK4a/ARF: a multifunctional tumor suppressor locus. Mutat Res 2005; 576: pp. 22-38
- 335. Ko A., Han S.Y., and Song J.: Dynamics of ARF regulation that control senescence and cancer. BMB Rep 2016; 49: pp. 598-606
- 336. Zhao Y., Yu H., and Hu W.: The regulation of MDM2 oncogene and its impact on human cancers. Acta Biochim Biophys Sin Shanghai 2014; 46: pp. 180-189
- 337. Bueso-Ramos C.E., Yang Y., deLeon E., McCown P., Stass S.A., and Albitar M.: The human MDM-2 oncogene is overexpressed in leukemias. Blood 1993; 82: pp. 2617-2623
- 338. Oliner J.D., Kinzler K.W., Meltzer P.S., George D.L., and Vogelstein B.: Amplification of a gene encoding a p53-associated protein in human sarcomas. Nature 1992; 358: pp. 80-83
- 339. Borg A., Johannsson U., Johannsson O., Hakansson S., Westerdahl J., Masback A., et al: Novel germline p16 mutation in familial malignant melanoma in southern Sweden. Cancer Res 1996; 56: pp. 2497-2500
- 340. Cairns P., Mao L., Merlo A., Lee D.J., Schwab D., Eby Y., et al: Rates of p16 (MTS1) mutations in primary tumors with 9p loss. Science 1994; 265: pp. 415-417
- 341. Caldas C., Hahn S.A., da Costa L.T., Redston M.S., Schutte M., Seymour A.B., et al: Frequent somatic mutations and homozygous deletions of the p16 (MTS1) gene in pancreatic adenocarcinoma. Nat Genet 1994; 8: pp. 27-32
- 342. Meng X., Franklin D.A., Dong J., and Zhang Y.: MDM2-p53 pathway in hepatocellular carcinoma. Cancer Res 2014; 74: pp. 7161-7167
- 343. Quelle D.E., Zindy F., Ashmun R.A., and Sherr C.J.: Alternative reading frames of the INK4a tumor suppressor gene encode two unrelated proteins capable of inducing cell cycle arrest. Cell 1995; 83: pp. 993-1000
- 344. Bashir T., and Pagano M.: Aberrant ubiquitin-mediated proteolysis of cell cycle regulatory proteins and oncogenesis. Adv Cancer Res 2003; 88: pp. 101-144
- 345. Devoy A., Soane T., Welchman R., and Mayer R.J.: The ubiquitin-proteasome system and cancer. Essays Biochem 2005; 41: pp. 187-203
- 346. Mani A., and Gelmann E.P.: The ubiquitin-proteasome pathway and its role in cancer. J Clin Oncol 2005; 23: pp. 4776-4789
- 347. Reed S.I.: The ubiquitin-proteasome pathway in cell cycle control. Results Probl Cell Differ 2006; 42: pp. 147-181
- 348. Yamasaki L., and Pagano M.: Cell cycle, proteolysis and cancer. Curr Opin Cell Biol 2004; 16: pp. 623-628
- 349. Cenciarelli C., Chiaur D.S., Guardavaccaro D., Parks W., Vidal M., and Pagano M.: Identification of a family of human F-box proteins. Curr Biol 1999; 9: pp. 1177-1179
- 350. Kipreos E.T., and Pagano M.: The F-box protein family. Genome Biol 2000; 1:
- 351. Skaar J.R., and Pagano M.: Control of cell growth by the SCF and APC/C ubiquitin ligases. Curr Opin Cell Biol 2009; 21: pp. 816-824
- 352. Nakayama K.I., and Nakayama K.: Regulation of the cell cycle by SCF-type ubiquitin ligases. Semin Cell Dev Biol 2005; 16: pp. 323-333
- 353. Peters J.M.: SCF and APC: the Yin and Yang of cell cycle regulated proteolysis. Curr Opin Cell Biol 1998; 10: pp. 759-768
- 354. Cardozo T., and Pagano M.: The SCF ubiquitin ligase: insights into a molecular machine. Nat Rev Mol Cell Biol 2004; 5: pp. 739-751
- 355. Nishitani H., Sugimoto N., Roukos V., Nakanishi Y., Saijo M., Obuse C., et al: Two E3 ubiquitin ligases, SCF-Skp2 and DDB1-Cul4, target human Cdt1 for proteolysis. EMBO J 2006; 25: pp. 1126-1136
- 356. Cao J., Ge M.H., and Ling Z.Q.: Fbxw7 tumor suppressor: a vital regulator contributes to human tumorigenesis. Medicine (Baltimore) 2016; 95:
- 357. Xu W., Taranets L., and Popov N.: Regulating Fbw7 on the road to cancer. Semin Cancer Biol 2016; 36: pp. 62-70
- 358. Wang H., and Maitra A.: The emerging roles of F-box proteins in pancreatic tumorigenesis. Semin Cancer Biol 2016; 36: pp. 88-94
- 359. Heestand G.M., and Kurzrock R.: Molecular landscape of pancreatic cancer: implications for current clinical trials. Oncotarget 2015; 6: pp. 4553-4561
- 360. Borras E., San Lucas F.A., Chang K., Zhou R., Masand G., Fowler J., et al: Genomic landscape of colorectal mucosa and adenomas. Cancer Prev Res (Phila) 2016; 9: pp. 417-427
- 361. Zhang W., and Koepp D.M.: Fbw7 isoform interaction contributes to cyclin E proteolysis. Mol Cancer Res 2006; 4: pp. 935-943
- 362. Nash P., Tang X., Orlicky S., Chen Q., Gertler F.B., Mendenhall M.D., et al: Multisite phosphorylation of a CDK inhibitor sets a threshold for the onset of DNA replication. Nature 2001; 414: pp. 514-521
- 363. Tan Y., Sangfelt O., and Spruck C.: The Fbxw7/hCdc4 tumor suppressor in human cancer. Cancer Lett 2008; 271: pp. 1-12
- 364. Fujii Y., Yada M., Nishiyama M., Kamura T., Takahashi H., Tsunematsu R., et al: Fbxw7 contributes to tumor suppression by targeting multiple proteins for ubiquitin-dependent degradation. Cancer Sci 2006; 97: pp. 729-736
- 365. Fuchs S.Y.: Tumor suppressor activities of the Fbw7 E3 ubiquitin ligase receptor. Cancer Biol Ther 2005; 4: pp. 506-508
- 366. Minella A.C., and Clurman B.E.: Mechanisms of tumor suppression by the SCF(Fbw7). Cell Cycle 2005; 4: pp. 1356-1359
- 367. Wang Z., Inuzuka H., Zhong J., Wan L., Fukushima H., Sarkar F.H., et al: Tumor suppressor functions of FBW7 in cancer development and progression. FEBS Lett 2012; 586: pp. 1409-1418
- 368. Akhoondi S., Sun D., von der Lehr N., Apostolidou S., Klotz K., Maljukova A., et al: FBXW7/hCDC4 is a general tumor suppressor in human cancer. Cancer Res 2007; 67: pp. 9006-9012
- 369. Wang L., Ye X., Liu Y., Wei W., and Wang Z.: Aberrant regulation of FBW7 in cancer. Oncotarget 2014; 5: pp. 2000-2015
- 370. Lau A.W., Fukushima H., and Wei W.: The Fbw7 and betaTRCP E3 ubiquitin ligases and their roles in tumorigenesis. Front Biosci 2012; 17: pp. 2197-2212
- 371. Furstenthal L., Kaiser B.K., Swanson C., and Jackson P.K.: Cyclin E uses Cdc6 as a chromatin-associated receptor required for DNA replication. J Cell Biol 2001; 152: pp. 1267-1278
- 372. Ekholm-Reed S., Mendez J., Tedesco D., Zetterberg A., Stillman B., and Reed S.I.: Deregulation of cyclin E in human cells interferes with prereplication complex assembly. J Cell Biol 2004; 165: pp. 789-800
- 373. Rajagopalan H., and Lengauer C.: hCDC4 and genetic instability in cancer. Cell Cycle 2004; 3: pp. 693-694
- 374. Rajagopalan H., Jallepalli P.V., Rago C., Velculescu V.E., Kinzler K.W., Vogelstein B., et al: Inactivation of hCDC4 can cause chromosomal instability. Nature 2004; 428: pp. 77-81
- 375. Spruck C.H., Won K.A., and Reed S.I.: Deregulated cyclin E induces chromosome instability. Nature 1999; 401: pp. 297-300
- 376. Hanashiro K., Kanai M., Geng Y., Sicinski P., and Fukasawa K.: Roles of cyclins A and E in induction of centrosome amplification in p53-compromised cells. Oncogene 2008; 27: pp. 5288-5302
- 377. Mussman J.G., Horn H.F., Carroll P.E., Okuda M., Tarapore P., Donehower L.A., et al: Synergistic induction of centrosome hyperamplification by loss of p53 and cyclin E overexpression. Oncogene 2000; 19: pp. 1635-1646
- 378. Kawamura K., Izumi H., Ma Z., Ikeda R., Moriyama M., Tanaka T., et al: Induction of centrosome amplification and chromosome instability in human bladder cancer cells by p53 mutation and cyclin E overexpression. Cancer Res 2004; 64: pp. 4800-4809
- 379. Simone C., Resta N., Bagella L., Giordano A., and Guanti G.: Cyclin E and chromosome instability in colorectal cancer cell lines. Mol Pathol 2002; 55: pp. 200-203
- 380. Koepp D.M., Schaefer L.K., Ye X., Keyomarsi K., Chu C., Harper J.W., et al: Phosphorylation-dependent ubiquitination of cyclin E by the SCFFbw7 ubiquitin ligase. Science 2001; 294: pp. 173-177
- 381. Hao B., Oehlmann S., Sowa M.E., Harper J.W., and Pavletich N.P.: Structure of a Fbw7-Skp1-cyclin E complex: multisite-phosphorylated substrate recognition by SCF ubiquitin ligases. Mol Cell 2007; 26: pp. 131-143
- 382. Strohmaier H., Spruck C.H., Kaiser P., Won K.A., Sangfelt O., and Reed S.I.: Human F-box protein hCdc4 targets cyclin E for proteolysis and is mutated in a breast cancer cell line. Nature 2001; 413: pp. 316-322
- 383. Lau A.W., Inuzuka H., Fukushima H., Wan L., Liu P., Gao D., et al: Regulation of APC(Cdh1) E3 ligase activity by the Fbw7/cyclin E signaling axis contributes to the tumor suppressor function of Fbw7. Cell Res 2013; 23: pp. 947-961
- 384. Bhaskaran N., van Drogen F., Ng H.F., Kumar R., Ekholm-Reed S., Peter M., et al: Fbw7alpha and Fbw7gamma collaborate to shuttle cyclin E1 into the nucleolus for multiubiquitylation. Mol Cell Biol 2013; 33: pp. 85-97
- 385. Grady W.M., and Carethers J.M.: Genomic and epigenetic instability in colorectal cancer pathogenesis. Gastroenterology 2008; 135: pp. 1079-1099
- 386. Lengauer C., Kinzler K.W., and Vogelstein B.: Genetic instability in colorectal cancers. Nature 1997; 386: pp. 623-627
- 387. Lengauer C., Kinzler K.W., and Vogelstein B.: Genetic instabilities in human cancers. Nature 1998; 396: pp. 643-649
- 388. Gelsomino F., Barbolini M., Spallanzani A., Pugliese G., and Cascinu S.: The evolving role of microsatellite instability in colorectal cancer: a review. Cancer Treat Rev 2016; 51: pp. 19-26
- 389. Yamamoto H., and Imai K.: Microsatellite instability: an update. Arch Toxicol 2015; 89: pp. 899-921
- 390. Rajagopalan H., and Lengauer C.: CIN-ful cancers. Cancer Chemother Pharmacol 2004; 54: pp. S65-S68
- 391. Michor F., Iwasa Y., Vogelstein B., Lengauer C., and Nowak M.A.: Can chromosomal instability initiate tumorigenesis? Semin Cancer Biol 2005; 15: pp. 43-49
- 392. Rajagopalan H., and Lengauer C.: Aneuploidy and cancer. Nature 2004; 432: pp. 338-341
- 393. Rajagopalan H., Nowak M.A., Vogelstein B., and Lengauer C.: The significance of unstable chromosomes in colorectal cancer. Nat Rev Cancer 2003; 3: pp. 695-701
- 394. Shih I.M., Zhou W., Goodman S.N., Lengauer C., Kinzler K.W., and Vogelstein B.: Evidence that genetic instability occurs at an early stage of colorectal tumorigenesis. Cancer Res 2001; 61: pp. 818-822
- 395. Sansregret L., and Swanton C.: The role of aneuploidy in cancer evolution. Cold Spring Harb Perspect Med 2017; 7:
- 396. Nicholson J.M., and Cimini D.: Link between aneuploidy and chromosome instability. Int Rev Cell Mol Biol 2015; 315: pp. 299-317
- 397. Potapova T.A., Zhu J., and Li R.: Aneuploidy and chromosomal instability: a vicious cycle driving cellular evolution and cancer genome chaos. Cancer Metastasis Rev 2013; 32: pp. 377-389
- 398. Thompson S.L., Bakhoum S.F., and Compton D.A.: Mechanisms of chromosomal instability. Curr Biol 2010; 20: pp. R285-R295
- 399. Lingle W.L., Lukasiewicz K., and Salisbury J.L.: Deregulation of the centrosome cycle and the origin of chromosomal instability in cancer. Adv Exp Med Biol 2005; 570: pp. 393-421
- 400. Pihan G.A., and Doxsey S.J.: The mitotic machinery as a source of genetic instability in cancer. Semin Cancer Biol 1999; 9: pp. 289-302
- 401. Tomonaga T., and Nomura F.: Chromosome instability and kinetochore dysfunction. Histol Histopathol 2007; 22: pp. 191-197
- 402. Bharadwaj R., and Yu H.: The spindle checkpoint, aneuploidy, and cancer. Oncogene 2004; 23: pp. 2016-2027
- 403. Michel L.S., Liberal V., Chatterjee A., Kirchwegger R., Pasche B., Gerald W., et al: MAD2 haplo-insufficiency causes premature anaphase and chromosome instability in mammalian cells. Nature 2001; 409: pp. 355-359
- 404. Kalitsis P., Earle E., Fowler K.J., and Choo K.H.: Bub3 gene disruption in mice reveals essential mitotic spindle checkpoint function during early embryogenesis. Genes Dev 2000; 14: pp. 2277-2282
- 405. Iwanaga Y., Chi Y.H., Miyazato A., Sheleg S., Haller K., Peloponese J.M., et al: Heterozygous deletion of mitotic arrest-deficient protein 1 (MAD1) increases the incidence of tumors in mice. Cancer Res 2007; 67: pp. 160-166
- 406. Babu J.R., Jeganathan K.B., Baker D.J., Wu X., Kang-Decker N., and van Deursen J.M.: Rae1 is an essential mitotic checkpoint regulator that cooperates with Bub3 to prevent chromosome missegregation. J Cell Biol 2003; 160: pp. 341-353
- 407. Perera D., Tilston V., Hopwood J.A., Barchi M., Boot-Handford R.P., and Taylor S.S.: Bub1 maintains centromeric cohesion by activation of the spindle checkpoint. Dev Cell 2007; 13: pp. 566-579
- 408. Putkey F.R., Cramer T., Morphew M.K., Silk A.D., Johnson R.S., McIntosh J.R., et al: Unstable kinetochore-microtubule capture and chromosomal instability following deletion of CENP-E. Dev Cell 2002; 3: pp. 351-365
- 409. Dai W., Wang Q., Liu T., Swamy M., Fang Y., Xie S., et al: Slippage of mitotic arrest and enhanced tumor development in mice with BubR1 haploinsufficiency. Cancer Res 2004; 64: pp. 440-445
- 410. Wang Q., Liu T., Fang Y., Xie S., Huang X., Mahmood R., et al: BUBR1 deficiency results in abnormal megakaryopoiesis. Blood 2004; 103: pp. 1278-1285
- 411. Baker D.J., Jeganathan K.B., Cameron J.D., Thompson M., Juneja S., Kopecka A., et al: BubR1 insufficiency causes early onset of aging-associated phenotypes and infertility in mice. Nat Genet 2004; 36: pp. 744-749
- 412. Jeganathan K., Malureanu L., Baker D.J., Abraham S.C., and van Deursen J.M.: Bub1 mediates cell death in response to chromosome missegregation and acts to suppress spontaneous tumorigenesis. J Cell Biol 2007; 179: pp. 255-267
- 413. Funk L.C., Zasadil L.M., and Weaver B.A.: Living in CIN: mitotic infidelity and its consequences for tumor promotion and suppression. Dev Cell 2016; 39: pp. 638-652
- 414. Schuyler S.C., YF W., and Kuan V.J.: The Mad1-Mad2 balancing act—a damaged spindle checkpoint in chromosome instability and cancer. J Cell Sci 2012; 125: pp. 4197-4206
- 415. Cahill D.P., Lengauer C., Yu J., Riggins G.J., Willson J.K., Markowitz S.D., et al: Mutations of mitotic checkpoint genes in human cancers. Nature 1998; 392: pp. 300-303
- 416. Shichiri M., Yoshinaga K., Hisatomi H., Sugihara K., and Hirata Y.: Genetic and epigenetic inactivation of mitotic checkpoint genes hBUB1 and hBUBR1 and their relationship to survival. Cancer Res 2002; 62: pp. 13-17
- 417. Gemma A., Seike M., Seike Y., Uematsu K., Hibino S., Kurimoto F., et al: Somatic mutation of the hBUB1 mitotic checkpoint gene in primary lung cancer. Genes Chromosom Cancer 2000; 29: pp. 213-218
- 418. Hempen P.M., Kurpad H., Calhoun E.S., Abraham S., and Kern S.E.: A double missense variation of the BUB1 gene and a defective mitotic spindle checkpoint in the pancreatic cancer cell line Hs766T. Hum Mutat 2003; 21: pp. 445
- 419. Imai Y., Shiratori Y., Kato N., Inoue T., and Omata M.: Mutational inactivation of mitotic checkpoint genes, hsMAD2 and hBUB1, is rare in sporadic digestive tract cancers. Jpn J Cancer Res 1999; 90: pp. 837-840
- 420. Ohshima K., Haraoka S., Yoshioka S., Hamasaki M., Fujiki T., Suzumiya J., et al: Mutation analysis of mitotic checkpoint genes (hBUB1 and hBUBR1) and microsatellite instability in adult T-cell leukemia/lymphoma. Cancer Lett 2000; 158: pp. 141-150
- 421. Nomoto S., Haruki N., Takahashi T., Masuda A., Koshikawa T., Fujii Y., et al: Search for in vivo somatic mutations in the mitotic checkpoint gene, hMAD1, in human lung cancers. Oncogene 1999; 18: pp. 7180-7183
- 422. Tsukasaki K., Miller C.W., Greenspun E., Eshaghian S., Kawabata H., Fujimoto T., et al: Mutations in the mitotic check point gene, MAD1L1, in human cancers. Oncogene 2001; 20: pp. 3301-3305
- 423. Wang Z., Cummins J.M., Shen D., Cahill D.P., Jallepalli P.V., Wang T.L., et al: Three classes of genes mutated in colorectal cancers with chromosomal instability. Cancer Res 2004; 64: pp. 2998-3001
- 424. Dominguez-Brauer C., Thu K.L., Mason J.M., Blaser H., Bray M.R., and Mak T.W.: Targeting mitosis in cancer: emerging strategies. Mol Cell 2015; 60: pp. 524-536
- 425. Gavriilidis P., Giakoustidis A., and Giakoustidis D.: Aurora kinases and potential medical applications of aurora kinase inhibitors: a review. J Clin Med Res 2015; 7: pp. 742-751
- 426. Penna L.S., Henriques J.A., and Bonatto D.: Anti-mitotic agents: are they emerging molecules for cancer treatment? Pharmacol Ther 2017; undefined: pp. 67-82
- 427. Katsha A., Belkhiri A., Goff L., and El-Rifai W.: Aurora kinase A in gastrointestinal cancers: time to target. Mol Cancer 2015; 14: pp. 106
- 428. Malumbres M., and Perez de Castro I.: Aurora kinase A inhibitors: promising agents in antitumoral therapy. Expert Opin Ther Targets 2014; 18: pp. 1377-1393
- 429. Chan K.S., Koh C.G., and Li H.Y.: Mitosis-targeted anti-cancer therapies: where they stand. Cell Death Dis 2012; 3:
- 430. Kinzler K.W., Nilbert M.C., LK S., Vogelstein B., Bryan T.M., Levy D.B., et al: Identification of FAP locus genes from chromosome 5q21. Science 1991; 253: pp. 661-665
- 431. Nishisho I., Nakamura Y., Miyoshi Y., Miki Y., Ando H., Horii A., et al: Mutations of chromosome 5q21 genes in FAP and colorectal cancer patients. Science 1991; 253: pp. 665-669
- 432. Nakamura Y., Nishisho I., Kinzler K.W., Vogelstein B., Miyoshi Y., Miki Y., et al: Mutations of the APC (adenomatous polyposis coli) gene in FAP (familial polyposis coli) patients and in sporadic colorectal tumors. Tohoku J Exp Med 1992; 168: pp. 141-147
- 433. Kinzler K.W., and Vogelstein B.: Lessons from hereditary colorectal cancer. Cell 1996; 87: pp. 159-170
- 434. LK S., Vogelstein B., and Kinzler K.W.: Association of the APC tumor suppressor protein with catenins. Science 1993; 262: pp. 1734-1737
- 435. Rubinfeld B., Souza B., Albert I., Muller O., Chamberlain S.H., Masiarz F.R., et al: Association of the APC gene product with beta-catenin. Science 1993; 262: pp. 1731-1734
- 436. Gumbiner B.M.: Signal transduction of beta-catenin. Curr Opin Cell Biol 1995; 7: pp. 634-640
- 437. Bienz M.: Beta-catenin: a pivot between cell adhesion and Wnt signalling. Curr Biol 2005; 15: pp. R64-R67
- 438. Willert K., and Nusse R.: Beta-catenin: a key mediator of Wnt signaling. Curr Opin Genet Dev 1998; 8: pp. 95-102
- 439. Novak A., and Dedhar S.: Signaling through beta-catenin and Lef/Tcf. Cell Mol Life Sci 1999; 56: pp. 523-537
- 440. Moon R.T., Bowerman B., Boutros M., and Perrimon N.: The promise and perils of Wnt signaling through beta-catenin. Science 2002; 296: pp. 1644-1646
- 441. Kikuchi A.: Regulation of beta-catenin signaling in the Wnt pathway. Biochem Biophys Res Commun 2000; 268: pp. 243-248
- 442. Haegebarth A., and Clevers H.: Wnt signaling, lgr5, and stem cells in the intestine and skin. Am J Pathol 2009; 174: pp. 715-721
- 443. van der Flier L.G., and Clevers H.: Stem cells, self-renewal, and differentiation in the intestinal epithelium. Annu Rev Physiol 2009; 71: pp. 241-260
- 444. Barker N., van de Wetering M., and Clevers H.: The intestinal stem cell. Genes Dev 2008; 22: pp. 1856-1864
- 445. de Lau W., Barker N., and Clevers H.: WNT signaling in the normal intestine and colorectal cancer. Front Biosci 2007; 12: pp. 471-491
- 446. Pinto D., and Clevers H.: Wnt control of stem cells and differentiation in the intestinal epithelium. Exp Cell Res 2005; 306: pp. 357-363
- 447. Pinto D., and Clevers H.: Wnt, stem cells and cancer in the intestine. Biol Cell 2005; 97: pp. 185-196
- 448. Reya T., and Clevers H.: Wnt signalling in stem cells and cancer. Nature 2005; 434: pp. 843-850
- 449. Clevers H., Loh K.M., and Nusse R.: Stem cell signaling. An integral program for tissue renewal and regeneration: wnt signaling and stem cell control. Science 2014; 346:
- 450. Krausova M., and Korinek V.: Wnt signaling in adult intestinal stem cells and cancer. Cell Signal 2014; 26: pp. 570-579
- 451. Yang V.W.: APC as a checkpoint gene: the beginning or the end? Gastroenterology 2002; 123: pp. 935-939
- 452. Bustos V.H., Ferrarese A., Venerando A., Marin O., Allende J.E., and Pinna L.A.: The first armadillo repeat is involved in the recognition and regulation of beta-catenin phosphorylation by protein kinase CK1. Proc Natl Acad Sci U S A 2006; 103: pp. 19725-19730
- 453. Marikawa Y., and Elinson R.P.: beta-TrCP is a negative regulator of Wnt/beta-catenin signaling pathway and dorsal axis formation in Xenopus embryos. Mech Dev 1998; 77: pp. 75-80
- 454. Winston J.T., Strack P., Beer-Romero P., Chu C.Y., Elledge S.J., and Harper J.W.: The SCFbeta-TRCP-ubiquitin ligase complex associates specifically with phosphorylated destruction motifs in IkappaBalpha and beta-catenin and stimulates IkappaBalpha ubiquitination in vitro. Genes Dev 1999; 13: pp. 270-283
- 455. Latres E., Chiaur D.S., and Pagano M.: The human F box protein beta-Trcp associates with the Cul1/Skp1 complex and regulates the stability of beta-catenin. Oncogene 1999; 18: pp. 849-854
- 456. Sakanaka C.: Phosphorylation and regulation of beta-catenin by casein kinase I epsilon. J Biochem 2002; 132: pp. 697-703
- 457. Gao Z.H., Seeling J.M., Hill V., Yochum A., and Virshup D.M.: Casein kinase I phosphorylates and destabilizes the beta-catenin degradation complex. Proc Natl Acad Sci U S A 2002; 99: pp. 1182-1187
- 458. Rubinfeld B., Albert I., Porfiri E., Fiol C., Munemitsu S., and Polakis P.: Binding of GSK3beta to the APC-beta-catenin complex and regulation of complex assembly. Science 1996; 272: pp. 1023-1026
- 459. Hart M.J., de los Santos R., Albert I.N., Rubinfeld B., and Polakis P.: Downregulation of beta-catenin by human Axin and its association with the APC tumor suppressor, beta-catenin and GSK3 beta. Curr Biol 1998; 8: pp. 573-581
- 460. Shtutman M., Zhurinsky J., Simcha I., Albanese C., D’Amico M., Pestell R., et al: The cyclin D1 gene is a target of the beta-catenin/LEF-1 pathway. Proc Natl Acad Sci U S A 1999; 96: pp. 5522-5527
- 461. He T.C., Sparks A.B., Rago C., Hermeking H., Zawel L., da Costa L.T., et al: Identification of c-MYC as a target of the APC pathway. Science 1998; 281: pp. 1509-1512
- 462. Tetsu O., and McCormick F.: Beta-catenin regulates expression of cyclin D1 in colon carcinoma cells. Nature 1999; 398: pp. 422-426
- 463. Green R.A., and Kaplan K.B.: Chromosome instability in colorectal tumor cells is associated with defects in microtubule plus-end attachments caused by a dominant mutation in APC. J Cell Biol 2003; 163: pp. 949-961
- 464. Green R.A., Wollman R., and Kaplan K.B.: APC and EB1 function together in mitosis to regulate spindle dynamics and chromosome alignment. Mol Biol Cell 2005; 16: pp. 4609-4622
- 465. Kaplan K.B., Burds A.A., Swedlow J.R., Bekir S.S., Sorger P.K., and Nathke I.S.: A role for the Adenomatous Polyposis Coli protein in chromosome segregation. Nat Cell Biol 2001; 3: pp. 429-432
- 466. Kita K., Wittmann T., Nathke I.S., and Waterman-Storer C.M.: Adenomatous polyposis coli on microtubule plus ends in cell extensions can promote microtubule net growth with or without EB1. Mol Biol Cell 2006; 17: pp. 2331-2345
- 467. Mogensen M.M., Tucker J.B., Mackie J.B., Prescott A.R., and Nathke I.S.: The adenomatous polyposis coli protein unambiguously localizes to microtubule plus ends and is involved in establishing parallel arrays of microtubule bundles in highly polarized epithelial cells. J Cell Biol 2002; 157: pp. 1041-1048
- 468. Bahmanyar S., Nelson W.J., and Barth A.I.: Role of APC and its binding partners in regulating microtubules in mitosis. Adv Exp Med Biol 2009; 656: pp. 65-74
- 469. Fodde R., Smits R., and Clevers H.: APC, signal transduction and genetic instability in colorectal cancer. Nat Rev Cancer 2001; 1: pp. 55-67
- 470. Fodde R., Kuipers J., Rosenberg C., Smits R., Kielman M., Gaspar C., et al: Mutations in the APC tumour suppressor gene cause chromosomal instability. Nat Cell Biol 2001; 3: pp. 433-438
- 471. Caldwell C.M., and Kaplan K.B.: The role of APC in mitosis and in chromosome instability. Adv Exp Med Biol 2009; 656: pp. 51-64
- 472. Tighe A., Johnson V.L., and Taylor S.S.: Truncating APC mutations have dominant effects on proliferation, spindle checkpoint control, survival and chromosome stability. J Cell Sci 2004; 117: pp. 6339-6353
- 473. Abal M., Obrador-Hevia A., Janssen K.P., Casadome L., Menendez M., Carpentier S., et al: APC inactivation associates with abnormal mitosis completion and concomitant BUB1B/MAD2L1 up-regulation. Gastroenterology 2007; 132: pp. 2448-2458
- 474. Aoki K., Aoki M., Sugai M., Harada N., Miyoshi H., Tsukamoto T., et al: Chromosomal instability by beta-catenin/TCF transcription in APC or beta-catenin mutant cells. Oncogene 2007; 26: pp. 3511-3520
- 475. Stumpff J., Ghule P.N., Shimamura A., Stein J.L., and Greenblatt M.: Spindle microtubule dysfunction and cancer predisposition. J Cell Physiol 2014; 229: pp. 1881-1883
- 476. Sherr C.J.: A new cell-cycle target in cancer—inhibiting cyclin D-dependent kinases 4 and 6. N Engl J Med 2016; 375: pp. 1920-1923
- 477. Sherr C.J., Beach D., and Shapiro G.I.: Targeting CDK4 and CDK6: from discovery to therapy. Cancer Discov 2016; 6: pp. 353-367
- 478. Finn R.S., Crown J.P., Ettl J., Schmidt M., Bondarenko I.M., Lang I., et al: Efficacy and safety of palbociclib in combination with letrozole as first-line treatment of ER-positive, HER2-negative, advanced breast cancer: expanded analyses of subgroups from the randomized pivotal trial PALOMA-1/TRIO-18. Breast Cancer Res 2016; 18: pp. 67
- 479. Casimiro M.C., Velasco-Velazquez M., Aguirre-Alvarado C., and Pestell R.G.: Overview of cyclins D1 function in cancer and the CDK inhibitor landscape: past and present. Expert Opin Investig Drugs 2014; 23: pp. 295-304
- 480. Finn R.S., Martin M., Rugo H.S., Jones S., Im S.A., Gelmon K., et al: Palbociclib and letrozole in advanced breast cancer. N Engl J Med 2016; 375: pp. 1925-1936
- 481. VanArsdale T., Boshoff C., Arndt K.T., and Abraham R.T.: Molecular pathways: targeting the cyclin D-CDK4/6 axis for cancer treatment. Clin Cancer Res 2015; 21: pp. 2905-2910
- 482. Dickson M.A.: Molecular pathways: CDK4 inhibitors for cancer therapy. Clin Cancer Res 2014; 20: pp. 3379-3383
- 483. Hamilton E., and Infante J.R.: Targeting CDK4/6 in patients with cancer. Cancer Treat Rev 2016; 45: pp. 129-138
- 484. Roskoski R.: Cyclin-dependent protein kinase inhibitors including palbociclib as anticancer drugs. Pharmacol Res 2016; 107: pp. 249-275
- 485. DiPippo A.J., Patel N.K., and Barnett C.M.: Cyclin-dependent kinase inhibitors for the treatment of breast cancer: past, present, and future. Pharmacotherapy 2016; 36: pp. 652-667
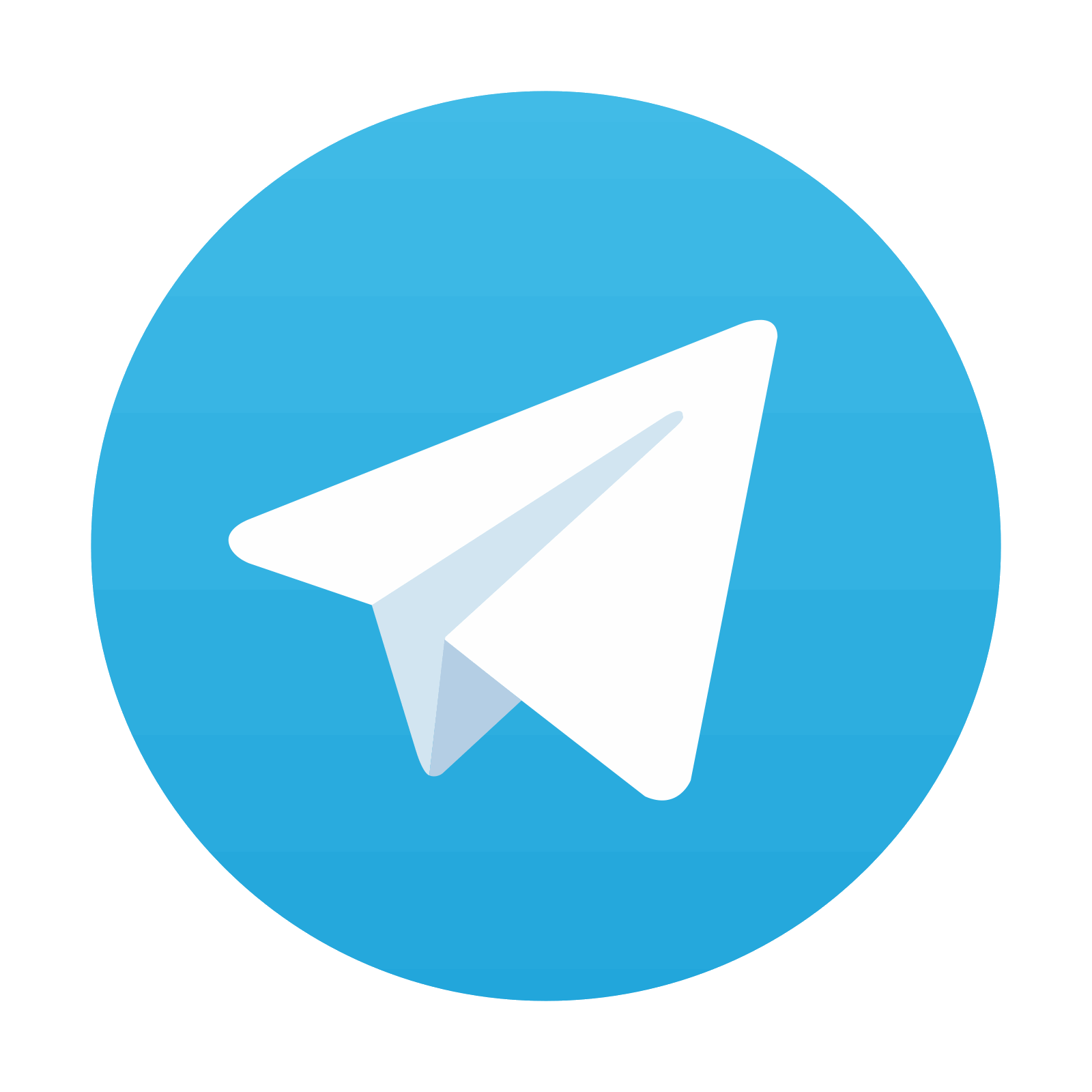
Stay updated, free articles. Join our Telegram channel
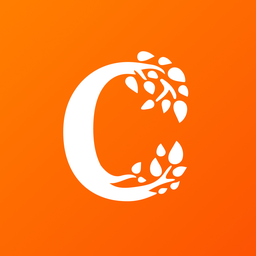
Full access? Get Clinical Tree
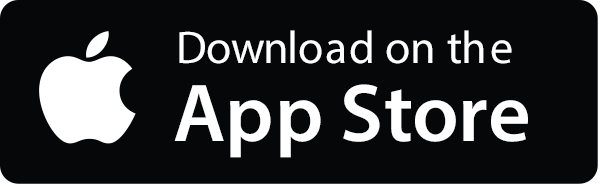
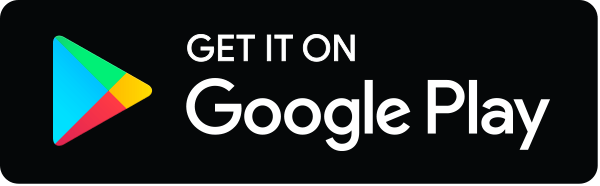