4 Oliver W. Hakenberg Department of Urology, University Hospital Rostock, Rostock, Germany Understanding the basic concept of the cell cycle and the theories behind cancer development and its progression is vital for understanding the principle of oncological therapies. Cancer can evade the immune system by various methods, which gives rise to repeated use of therapeutic agents such as chemotherapy and radiotherapy. In this chapter, the principle concepts of cancer growth and treatment are explained. Keywords carcinogenesis; cancer growth; chemotherapy; radiotherapy In the human body, there are roughly 300 different types of cells and 200 types of human cancers have been recorded. Urological malignancies account for more than 30% of all human organ neoplasias. A large part of urology, therefore, concerns the diagnosis and treatment of cancer. Most of these malignancies are cancers from epithelia of the urinary organs such as renal tumours from the tubular epithelia, urothelial cancer from bladder and upper tract epithelia, and malignancies from the testicles, the prostate, and the penis. Other malignancies such as sarcomas or lymphomas are rare. The natural history of urological cancers is rather diverse. Testicular carcinoma is rapidly progressive and highly aggressive. In contrast, prostate cancer (PCa), now the most common organ malignancy in men, is often a disease occurring in the elderly with slow progression. Urothelial cancers are a heterogeneous group characterised by more molecular subtypes than is apparent histologically. They arise as a result of exposure to carcinogens excreted in the urine. Because the carcinogenic exposure will continue after treatment, urothelial carcinomas have a high recurrence rate. Renal cell carcinoma is often detected incidentally on routine imaging and as the incidence increases with age, concepts of active surveillance for small renal tumours in elderly patients have been proposed. Organ‐preserving tumour surgery with preservation of renal function has become the standard for small renal tumours. Penile cancer is mostly an aggressive squamous cell carcinoma with the majority related to human papilloma viruses. There are many influences that can cause DNA damage and start a carcinogenic development. Mutagenic influences can be chemicals, ionising and ultraviolet (UV) radiation, viruses, or chronic inflammation. Mutagenic chemicals which cause many human cancers are either direct‐ or indirect‐acting carcinogens. Direct‐acting carcinogens are electrophiles that react with oxygen and nitrogen atoms in the DNA structure, altering nucleotides and disturbing normal base pairing. Indirect‐acting carcinogens are unreactive and water‐insoluble but become activated by the introduction of electrophilic compounds, often through the action of liver enzyme systems (e.g. cytochrome p450), which normally detoxifies noxious chemicals by making them water‐soluble and thus can be excreted. Some RNA viruses have amino acid sequences which are almost but not exactly the same as those of a natural human gene. If such a virus also possesses the enzyme reverse transcriptase, it can create a copy of itself in DNA, which will be taken up into the DNA of the human cell, like a kind of genetic cuckoo, and may have oncogenic effects [1, 2]. Human papilloma virus (HPV) is a DNA virus that causes genital warts. It produces two tumorigenic proteins (E5, E7), which stimulate proliferation by binding to growth receptors (E5) and by inhibiting a protein involved in cell cycle control (E7 and the Rb tumour suppressor protein). HPV virus types 16 and 18 induce squamous cell cancers of the genitals in both genders (i.e. penis, vulva, cervix, and anus) [3]. HPV DNA has also been found in the genome of some types of bladder cancer. Cancer is essentially a genetic disease characterised by genome instability. There may be inherited somatic mutations with resulting genetic defects which predispose to tumour development at a relatively early age (e.g. renal cancer in von Hippel‐Lindau syndrome). These account for roughly 10% of cases. However, the majority of cancers are sporadic and develop through a series of multiple‐chance mutations triggered by mutagenic influences. For a cancer to develop, several or many mutations in genes that normally control cell proliferation need to accumulate. This may take years, and therefore, sporadic cancers are a disease mainly of the elderly. Mitosis is the basis of cell replication in normal and cancer tissues. There are four phases of the mitotic cycle (Figure 4.1). First, there is a relatively long gap phase (G1) after the previous cell division when the newly divided cell seems to be resting. Mitosis takes about one hour and cells may live for hours or years, the difference depends on the duration of this first gap (G1). The next phase is that of protein synthesis (S), and DNA is now rapidly synthesised, doubling the original quantity. There follows a second relatively short gap phase (G2) between the completion of DNA synthesis and the beginning of mitosis (cell division), the final phase. Figure 4.1 Mitotic cycle. During mitosis, the genome is most vulnerable to mutations. Genes are helices of DNA strung together in the chromosomes which coil and uncoil in different phases of mitosis. In prophase, they are coiled in tight lengths which are apt to break and stick to each other, so that there may be crossing over during mitosis (Figure 4.2). Whole chromosomes are entirely duplicated, while small lengths, even single genes, can also become inserted into an adjoining chromosome. Normal human cells contain 23 pairs of chromosomes. Cancer cells, in contrast, usually have an abnormal number of chromosomes (aneuploidy) and often contain fused elements from different chromosomes (translocations) resulting from disordered mitosis (Figure 4.2). Figure 4.2 (a–c) In prophase, parts of chromosomes may stick to each other or become exchanged. It is in the brittle stage of prophase between G1 and S that the chromosomes are vulnerable to outside influences. Ionising radiation may cause short chains of genetic material to fall out of the DNA strand. Missing nucleotides are rapidly cemented back into position by the polynucleotide ligase enzyme, but not always in the correct order, and a wrong sequence may act either as a faulty gene or cause malfunction of genes. Such bogus genes may function normally, however, they may fail to respond to a switch‐off signal [4]. An alkylating agent can insert a single pair of bases into a chromosome during the S phase, and this trivial alteration can lead to a mutation [5]. The acquisition of the multiple developmental steps in tumorigenesis depend on a succession of alterations in the cellular genome. Mutations occur frequently in dividing cells (e.g. epithelia). Some mutations confer selection advantages to subclones of cells. Several additive mutations enable these subclones to outgrow others and eventually become dominant in a local tissue environment. Multistep tumour progression is the succession of clonal expansions by the chance development of a mutant genotype [6]. Normally, the stability of the genome is maintained by systems which detect and repair DNA defects, keeping the rate of spontaneous mutations very low. If there is too much DNA damage for repair, these cells are induced to die by programmed cell death (apoptosis). Thus, damage is repaired or severely damaged cells are eliminated, maintaining genomic stability. Cells with mutations on their way to tumorigenesis often avoid and disable the normal control mechanisms [7]. They also often increase their rate of acquiring further mutations by increased sensitivity to mutagenic agents. Progressive tumour development is characterised by increasing genomic instability with gains and losses of gene copy numbers across the cell genome. Several distinctive patterns of DNA mutations in many tumour types have been described [8]. Each cancer is a clone that essentially develops from a single cell. Multiple mutations are required for a cancer clone to develop (‘multiple hit theory’). This theory is supported by the epidemiological fact that the occurrence of most types of cancer increases dramatically with age. With normal cell cycle control mechanisms, the chance development of multiple mutations takes many years, even decades to occur. In precancerous lesions, a series of mutations, which often occur in a well‐defined order, have been identified. Such early somatic mutations occur in genes that are central to genomic control. Examples are the APC gene leading to the inappropriate expression of Myc, a transcription factor that induces expression of many genes which are needed for the transit from the G1 to the S phase of the cell cycle. Other mutations commonly occurring early in carcinogenesis are those of the K‐ras gene and the DCC gene [2]. The latter leads to inactivation of the p53 gene, which controls apoptosis in cells with damaged DNA (Figure 4.3). Figure 4.3 Alterations of cell cycle control mechanisms in cancer cells. Highly complex interacting pathways transmit intra‐ and extracellular signals, which in cancer cells result in genetic changes that sustain and enhance continuous proliferation. Inherited mutations – mutations in a germ line – will render an individual to have a mutated version of a gene in one allele. The other allele will have a normal gene and usually this will be adequate to prevent cancer formation. However, a chance mutation in the same gene in the normal allele can occur, and this chance is higher if only one normal gene is present. Therefore, these individuals will be at a higher risk of cancer development than individuals with two normal alleles (hereditary predisposition). This applies to about 10% of human cancers and usually occurs earlier in life. Oncogenes are genes which stimulate excessive proliferation when mutations occur (e.g. myc, ras, Bcl‐2). The normal gene without mutations is a proto‐oncogene. For a proto‐oncogene to develop into an oncogene, a gain‐of‐function mutation is required by point mutation, localised reduplication (gene amplification), or chromosomal translocation. Any of these can result in overexpression of the gene product. Gain‐of‐function mutations that convert a proto‐oncogene into an oncogene act dominantly because only one allele needs to be affected for an abnormally high expression of the gene product to be expressed. Often, several oncogenes are found in a cancer, and they can enhance each other’s effects (‘cooperativity’). Tumour suppressor genes encode proteins that control normal cell growth and proliferation, and if they acquire mutations, cell cycle control is disturbed. Five classes of proteins are encoded by tumour suppressor genes: intracellular proteins which inhibit cell cycle progression (e.g. p16 cyclin‐kinase inhibitor), membrane receptors for growth inhibitor signals (e.g. tumour‐derived growth factor β), checkpoint control proteins (e.g. p53), apoptosis promotors, and proteins (enzymes) involved in DNA repair [9]. Many cancers have inactivating mutations of tumour suppressor genes (e.g. of APC, p53, RB, p16) (Figure 4.4). These are loss‐of‐function mutations leading to an underexpression of the gene product. Because both alleles of the cell have to be affected for the gene product to be abnormally low or non‐functional, mutations in tumour suppressor genes act recessively. Figure 4.4 Important proteins in cell cycle control (red) are commonly overexpressed as a result of mutations in proto‐oncogenes or underexpressed as a result of mutations in tumour‐suppressor genes. In a somatic cell which contains a mutant tumour suppressor gene, the normal allele will usually maintain normal and functional expression of the encoded regulatory protein. This cell is heterozygous for the tumour suppressor gene. For the mutation to become effective, this heterozygosity has to be lost (loss of heterozygosity [LOH]). This can occur through errors during mitosis involving the chromosomes (non‐disjunction, mitotic recombination). There are many known effectors regulating cell cycle control which can become oncogenes or defective tumour suppressor genes and control complex intracellular pathways (e.g. cyclins, cyclin‐dependent kinases [Cdks], Rb) [10]. Cyclins are mitogenic activators stimulating cyclin‐dependent kinases. The p16 protein (a tumour suppressor) inhibits cyclin‐D‐dependent kinase. The Rb protein binds a transcription factor complex (E2F), which when unbound activates DNA synthesis [11]. Mutations of p16 or Rb are often found in cancers (Figure 4.4). An essential checkpoint is the p53 gene (‘the caretaker of the genome’), and mutations of this gene are found in more than 50% of human cancers [12]. Uncontrolled proliferation of cell clones with mutations can be compared to a car going at high speed, whereby oncogenes are a defect accelerator (which cannot be released) and defective tumour suppressor genes are a nonfunctioning brake. Thus, if both defects occur simultaneously, uncontrolled driving at high speed (proliferation) will occur. Cancer cells are characterised by continuous and unrestricted proliferation, leading to unrestricted growth. There are several biological capabilities which need to be acquired during multistep carcinogenesis. These are the ability (i) to sustain proliferative activity, (ii) to evade growth suppressors, (iii) to resist cell death, (iv) to acquire replicative immortality, (v) to induce angiogenesis, and (vi) to activate invasion and metastasis (‘hallmarks of cancer’) [13]. In addition, cancers have to resist elimination by the immune system and have an altered energy metabolism. Normal tissues control the production and release of growth‐promoting signals that initiate entry into the mitotic cycle, maintaining homeostasis and normal tissue architecture. Cancer cells deregulate these control mechanisms. The promoting signals are usually growth factors that bind to cell‐surface receptors coupled to intracellular tyrosine‐kinase domains. These respond by sending signals through intracellular signalling pathways that regulate the cell cycle. Some of this growth‐inducing signalling is affected by releasing growth factors into the intercellular space and is transferred from cells to their neighbours (paracrine stimulation). Cancer cells acquire the ability to sustain proliferative signalling. This may be by the production of growth factors by the cancer cells themselves (autocrine stimulation), by inducing cells of the tumour stroma to produce growth factors or by becoming hyper‐responsive to normal levels’ growth factors [10]. Some cancer cells may become independent of growth factor stimulation altogether. In some cancer types, somatic mutations lead to the continuous activation of intracellular signalling pathways downstream from the activation of cell membrane receptors (e.g. B‐Raf/MAP‐kinase pathway, PI3‐kinase). Another mechanism can be defects in feedback mechanisms which normally negatively regulate proliferation (e.g. Ras‐oncoprotein, PTEN phosphatase, mTOR pathway). Paradoxically, excessive growth signalling (e.g. produced by oncoproteins such as ras, myc, and raf) may lead to opposite responses in some cancer cells (i.e. the induction of a dormant state [senescence] or cell death [apoptosis]) [14]. In normal cells, potent pathways negatively regulate cell proliferation, and some of these depend on intact tumour suppressor genes (e.g. Rb, p53), which are often inactivated in cancer cells. The gene products (RB, TP53) are central control nodes of two complementary regulatory cellular circuits that control whether cells go into proliferation or into senescence or apoptosis. RB transduces mainly growth‐inhibitory signals that originate largely outside of the cell. TP53 receives input from stress and abnormality sensors within the cell. If there is excessive genomic damage or disturbed metabolism, TP53 can arrest further cell‐cycle progression until things have normalised or it can trigger apoptosis (Figure 4.3). Cancer cells also need to evade ‘contact inhibition’. Some known effectors of normal contact inhibition are proteins such as the Merlin protein (product of the NF2 gene), which acts on cell‐surface adhesion molecules (e.g. E‐cadherin), and the LKB1 protein, which may be deregulated or deficient in cancer cells (Figure 4.3). There are different forms of cell death: apoptosis, autophagy, necrosis, and a dormant state (senescence). The ‘programmed’ cell death (apoptosis) is triggered by intracellular mechanisms in response to various physiologic stresses. Apoptosis can be initiated by both intra‐ and extracellular mechanisms, the intrinsic and the extrinsic system (working via the Fas‐receptor). This leads to activation of proteases (caspase 8 and 9) inducing proteolysis and disassembly of the cell [4]. The ‘apoptotic trigger’ is controlled by the balance of pro‐ and anti‐apoptotic effectors. Inhibitors of apoptosis are members the Bcl‐2 family, pro‐apoptotic signalling proteins are, for example, Bak and Bax, which are released from mitochondrial membranes, and cytochrome C, which activates intracellular caspases. A critical intracellular ‘damage sensor’ is the TP53 protein which activates apoptosis when there is too much DNA damage. Many cancers evade cellular apoptosis by inactivation of the function of TP53. Other tumour cells can increase the expression of anti‐apoptotic regulators (e.g. Bcl‐2), can downregulate pro‐apoptotic factors (Bax, PUMA), or can increase ‘survival signals’ (e.g. insulin‐like growth factors 1/2). ‘Autophagy’ is another physiologic response to cellular stress. It occurs most notably in the case of nutrient deficiency and triggers the breakdown of intracellular organelles (ribosomes, mitochondria). The catabolites are reused for energy metabolism, and this allows survival of the cell in stressful environments. Autophagy is also regulated by a system of promoting and counteracting effector mechanisms. The pathways involve the PI3‐kinase, AKT, and mTOR kinases which block both apoptosis and autophagy. They can be downregulated in cancer cells. Paradoxically, autophagy allows survival of cancer cells in stressful situations such as chemotherapy and radiotherapy. Thus, this survival response may enable the persistence and eventual regrowth of surviving cancer cells. Necrosis leads to bursting of cells, which is in contrast to apoptosis where they shrink and are consumed by their neighbours. Bursting necrotic cells expulse their contents into the environment, and in contrast to apoptosis and autophagy, release proinflammatory signals. These signals recruit inflammatory cells and can be actively tumour‐promoting by inducing angiogenesis, proliferation, and invasiveness. Senescence is the induction of a quiescent state in which cell proliferation is stopped, but the cell does not die. This state may also be induced in response to stressful changes in the microenvironment. Senescent cells can remain dormant for a long time but may be reactivated and turn on proliferation again later. Normal cells are only able to pass through a limited number of cycles of cell division. After repetitive cell cycles, either senescence is induced, or cells undergo apoptosis. Very few cells of a cancer cell population attain immortality with an unlimited number of cell cycles. A crucial factor for achieving immortality seems to be the expression of the enzyme telomerase. Telomeres are the ends of chromosomes and consist of arrays of a very short DNA sequence (TTAGGG). As DNA polymerases are unable to replicate the very ends of a double‐stranded DNA molecule, a reverse transcriptase (telomerase) – if expressed – adds repetitive TTAGGG sequences to the ends of the chromosomes. Most normal human cells do not have a telomerase, and thus, the continual loss of TTAGGG repeats from the chromosomal ends (telomeres) with each cell cycle limiting the number of further possible cell divisions. The progressive loss of telomeres is thought to be a mechanism of human ageing. Cancer cells that express telomerase can attain cellular immortality [15]. Cells need nutrients and oxygen as well as the evacuation of metabolic waste products and CO2. A cancer can grow to roughly 106 cells without its own blood supply. For further growth, the tumour has to develop new vasculature (Figure 4.5). The basal lamina of existing capillaries has to be destroyed, endothelial cells have to migrate to form new tubes (vasculogenesis), and new vessels have to sprout (angiogenesis). In cancer, this is stimulated by growth factors such as basic fibroblast growth factor (bFGF), transforming growth factor‐α (TGFα), and vascular endothelial growth factor (VEGF). Figure 4.5 Diffusion of oxygen and nutrients can only supply a tumour <2 mm in diameter. For further growth, angiogenesis has to be initiated. Temporary angiogenesis is a part of wound healing. In cancer, an ‘angiogenic switch’ has to be activated early on. Again, this angiogenic signal is controlled by a system of counteracting effectors, by promotors of angiogenesis (e.g. VEGF‐A), fibroblast growth factor (FGF) and by antagonists such as angiogenin, endostatin, thrombospondin‐1 (TSP‐1), and direct antagonists of the VEGF receptor. VEGF signalling occurs through three receptor tyrosine‐kinases (VEGFR 1–3) as a complex cascade. VEGF gene expression can be upregulated by hypoxia as well as by oncogene signalling. VEGF ligands can also be released in the extracellular space by matrix‐degrading proteases (e.g. MMP‐9). The essentially irregular signalling which stimulates tumour angiogenesis characteristically results in neovasculature with disordered capillary sprouting, convoluted vessels, and irregular blood flow with microhaemorrhaging and leakiness. Tumour angiogenesis is highly variable; it is characteristically high in renal cancer. Pericytes surrounding and supporting endothelial cells are an important part of tumour angiogenesis. Also, it has become recognised that cell types originating in the bone marrow – macrophages, neutrophils, mast cells and myeloid progenitor cells – infiltrate the periphery of tumours and there promote angiogenesis. Invasion and metastasis is thought of as a multistep process starting with local invasion, followed by intravasation of cancer cells into blood and lymph vessels, transit through these vessels to other sites, escape from vascular lumina (extravasation), formation of very small nodules (micrometasases), and finally the growth into sizable nodules (colonisation). This complex process is understood to follow a regulatory program, the epithelial‐mesenchymal transition (EMT) pathway. There are several transcriptional factors regulating the EMT during embryogenesis (when cells have to migrate for organ development). These factors can be re‐expressed by cancer cells inducing the loss of intercellular adherence junctions, the production of matrix‐degrading enzymes, and the resistance to apoptosis. Tumour‐associated stroma cells have a role in regulating the EMT, which often is most prominent in the tumour periphery (invasion front). Cancer cells that migrate are characterised by a loss of cell‐to‐cell adhesion molecules (e.g. E‐cadherin). In contrast, embryonic promotors of migration are often upregulated (e.g. N‐cadherin). Less is known about colonisation. Cancer cells need to adapt to foreign tissue microenvironments to grow into nodules. Colonisation is not identical with vascular transit because circulating tumour cells can be detected in many patients without metastases. Also, micrometastases often never progress to macroscopic nodules. Some primary tumours release suppressor factors that render micrometastases dormant as shown by the sometimes explosive metastatic growth soon after surgical resection of the primary tumour. Dormant micrometastses may also be kept inactive by a lack of nutrients or by an inability to locally activate angiogenesis. Certain tissue microenvironments may be hostile to colonisation, whereas others can be inducive. Metastatic colonies may proceed to disseminate further to new sites in the body as well as back to the primary tumour (‘reseeding’). The phenotypes and gene expression programmes of the population of cancer cells within primary tumours may be significantly modified by this reverse migration. Virtually every cancer contains immune cells, but this varies considerably. Originally this was thought to reflect an attempt by the immune system to fight malignancy. However, it is now understood that the tumour‐associated inflammatory response largely has the paradoxical effect of enhancing progression. Inflammation contributes to the tumorigenic capabilities by supplying growth, survival and proangiogenic factors, as well as, extracellular matrix modifying enzymes to the microenvironment. Otto Warburg first observed that cancer cell metabolism is different from that of normal cells [16]. Under aerobic conditions, normal cells process glucose in the cytosol to pyruvate via glycolysis and in the mitochondria to CO2. Under anaerobic conditions, glycolysis prevails, and little pyruvate is transferred to the oxygen‐consuming mitochondria. Cancer cells limit their metabolism largely to glycolysis even under aerobic conditions (i.e. aerobic glycolysis). This seems paradoxical because the efficiency of ATP production by glycolysis is much lower (about 18‐fold) compared to oxidative phosphorylation [17]. Cancer cells achieve this by upregulating glucose‐transporters (e.g. GLUT1), thereby increasing glucose transport to the cytoplasm. This is a feature that positron emission tomography (PET) scan imaging with radiolabelled glucose analogues (18F‐fluorodeoxyglucose [FDG]) utilises to visualise malignant lesions. This metabolic switch characteristic of cancer is associated with activated oncogenes (e.g. RAS, Myc), downregulated supressors (e.g. p53), and often increased levels of hypoxia‐inducible factors (HIF‐1α, ‐2α) which upregulate glycolysis and induce angiogenesis. This preference for low oxygen metabolism allows growth under unfavourable hypoxic conditions. Patients who are immunocompromised have an increased incidence of cancers; however, most of these are induced by viruses. Although the theory that the immune system monitors and destroys small malignancies has been challenged, there are anti‐tumoral immune responses in some cancers. However, some highly immunogenic cancer cells develop the ability to evade immune destruction by disabling components of the immune system that have been activated to eliminate them. Cancer cells may paralyse infiltrating cytotoxic lymphocytes (CTLs) and natural killer (NK) cells by secreting tumour growth factor (TGF‐β) or by recruiting inflammatory cells which are immunosuppressive (e.g. regulatory T‐cells). Solid cancers are highly complex tissues where neoplastic cells constitute only one compartment (the parenchyma). In addition, there is a lot of stroma consisting of vasculature as well as inflammatory and other cells. The population of cancer cells within a tumour is highly heterogeneous, sometimes but not always reflected in histological diversity with regions marked by different degrees of differentiation, proliferation, vascularity, inflammation, and invasiveness. An important subclass of cancer cells in a tumour are cancer stem cells (CSCs). Only these seem to have the ability to seed new tumours [18]. The number of CSCs in a tumour is highly variable. As CSCs can regrow after toxic treatment, they are thought to be the source of recurrence because they are more resistant to toxic treatments. CSCs often share transcriptional profiles with some normal tissue stem cell populations. The rate of tumour growth depends on its rate of proliferation. As tumours become larger, the rate of proliferation often slows down (i.e. the Gompertzian effect) (Figure 4.6). Tumour doubling time (DT) depends on the difference between the rates of proliferation and cell death. In small tumours, the DT is fairly constant. After 20 DTs there will be 106 cells (1 mg), after another 10 DTs the resulting 109 cells will weigh about 1 g (Figure 4.7). After another 10 DTs, the tumour will now have 1012 cells and weigh about 1 kg [19]. Such a large tumour by then will have outgrown its blood supply and will have slowed its rate of further growth considerably. If tumour growth is more rapid than its own blood supply can support, often internal necrosis occurs (as in large renal cancers). Tumour DT varies greatly in different cancers. In terms of DT, the tiny tumour which seems ‘early’ to the surgeon has already been present for two‐thirds of its possible natural life. Figure 4.6 The Gompertz curve: cell division slows down as the tumour enlarges. Figure 4.7 Theoretical weight of tumours of different doubling times (one day to three weeks). Cancers are diagnosed either on the basis of symptoms, incidentally by imaging done for unrelated reasons, or detected during a check‐up for an asymptomatic patient. Obtaining a biopsy from a lesion before surgical excision is not necessarily needed. PCa requires histological verification before treatment. Bladder cancer is resected transurethrally, so biopsy and treatment are one step. Renal cancer is characterised reliably by imaging, and percutaneous biopsy is rarely required. Testicular cancer should not be biopsied at all because breaching the anatomic scrotal barriers can induce metastatic spread. The superficially accessible penile cancer should be biopsied before treatment. Tumour markers are proteins that can be measured in serum and can be useful for the diagnosis and for monitoring treatment response. In urology, this applies to prostate and testicular cancer. The availability of prostate‐specific antigen (PSA) testing has increased the ability to detect early PCa. Its unrestrained use has led to the increased detection of low‐risk cancers and to controversial discussions about overdiagnosis and overtreatment. Testicular cancers often produce α‐fetoprotein or human chorionic gonadotropin (β‐HCG), which are foetal proteins which are not produced by healthy adults. These markers are especially useful for monitoring chemotherapy response. Also in testicular cancer, serum lactate dehydrogenase (LDH) is a marker of disease burden in metastatic stages. Treatment of cancer requires adequate grading and staging. The histological diagnosis should provide an assessment of tumour differentiation and the degree of aggressiveness (WHO tumour grading). Staging implies imaging by computed tomography (CT) or magnetic resonance imaging (MRI) to assess tumour extent, involvement of regional lymph nodes, and metastases. For some cancers, grading and staging allows a prognostic risk classification. Stratifying tumours into low‐, intermediate‐, and high‐risk groups has been shown to be useful for treatment decisions in prostate and non‐muscle‐invasive bladder cancer. A similar classification, including tumour markers, is used for testicular cancer. For some cancers, validated nomograms have been devised, based on data of several thousand patients, for the estimation of the likelihood of metastases and progression (e.g. Partin tables in PCa, European Organisation for Research and Treatment of Cancer [EORTC] tables in non‐muscle‐invasive bladder cancer). When and how to treat a neoplasm is sometimes a difficult decision. Tumour stage and extent of disease burden will indicate whether cure is possible, unlikely, or clearly impossible and whether systemic treatment will be needed additionally or on its own. Curative treatment aims at complete removal or destruction of the malignancy with a chance of long‐term survival. In localised disease, treatment with curative intent is the treatment of choice for most patients. If the patient is very old or unfit because of chronic diseases (comorbidity) and the tumour is unlikely to lead to symptoms, treatment may not be indicated or it may be deferred. This applies to localised PCa in patients with a life expectancy of less than 10 years, and it can apply to patients with small renal tumours. Palliative treatment does not aim for cure but for reducing symptoms (e.g. pain), alleviating functional problems (e.g. renal hydronephrosis), or delaying progression and prolonging life (e.g. by palliative chemotherapy). Quality of life (QoL) should always be considered. Palliative treatment is often multimodal, combing surgery, radio‐ and chemotherapy with pain management and supportive treatment. The objectives which can be achieved should be discussed with the patient. The term ‘active surveillance’ is usually applied to PCa. It implies that after histological diagnosis, treatment is deferred or not done at all because the tumour seems unlikely to progress, and curative treatment is offered if signs of progression develop. Therefore, regular examinations and bloods tests are required. In contrast, ‘watchful waiting’ implies that no treatment is undertaken at all until the patient becomes symptomatic. This is an option in patients who are very elderly and have comorbidities. Sometimes these terms are also used for the observation of small renal tumours in elderly patients by regular imaging to see whether these lesions are growing and do require treatment after all. Complete surgical removal of a solid organ malignancy remains the most reliable curative treatment option. The chance is highest if the cancer is confined to an organ. Involvement of regional lymph nodes reduces the chances of recurrence, even with regional lymphadenectomy. With systemic metastatic disease, surgery alone, however, cannot be curative. Radical surgery of a carcinoma usually implies complete organ removal. Organ sparing by excision of only the cancer is a concept which has only been established for renal and penile cancers. Partial cystectomy for muscle‐invasive bladder cancer is not a reliably curative option and organ sparing in testicular cancer in patients with only one testicle is still an experimental approach. There are several basic principles of tumour surgery. First, exposure needs to be adequate to gain safe access to the tumour vessels. Whenever possible, the main supplying vessels should be secured and ligated before handling the tumour itself. Second, the tumour must be handled as little as possible (i.e. not touched) to avoid haematogenous dissemination of tumour cells. Also, the tumour should not be injured and never be incised to avoid spillage of tumour cells (e.g. intraperitoneally). Mobilisation of a tumour or tumour‐bearing organ should include surrounding connective tissue as far as possible to achieve adequate and negative surgical margins. This can be difficult, and in some procedures, such as radical prostatectomy, anatomically limiting. Positive surgical margins (i.e. microscopically incomplete tumour resection) decreases the chance of long‐term survival considerably. The width of a negative margin can be very narrow for some cancers as in partial nephrectomy or in penile cancer. The first metastatic spread from cancer occurs to the regional lymph nodes. The removal of the regional lymph nodes, even if they are macroscopically normal, with the surgery of the primary tumour is therefore considered a standard for most organ malignancies. An exception is renal cancer where regional lymphadenectomy of normal nodes does not improve survival. Surgery for singular or limited metastatic disease may be indicated in some slowly proliferating cancers. For renal cancer, where metastatic disease may appear many years after primary treatment, surgery for limited metastatic disease, especially pulmonary metastases, prolongs survival. In testicular cancer, postchemotherapy surgery for hepatic metastases can be indicated together with retroperitoneal lymph node dissection. It is the use of ionising radiation for the treatment of malignancy with curative intent or as part of palliation for symptom relief. In a linear accelerator machine, electrons (from an electrical source) are accelerated with microwaves to high energies and are abruptly stopped when they collide with a tungsten metal filament. The energy released from the collision produce X‐rays, which are focused into a beam and used on a target organ. γ‐rays are produced by the nuclear decay of radioactive elements, the excess energy emitted from an unstable nucleus as it decays into a stable form. Both x‐rays and γ‐rays are electromagnetic radiation. As the radiation hits the target organ, it causes direct and indirect damage. Direct ionisation of the atoms causes damage which leads to chemical bond breakage and biological cascades that lead to the death of the targeted cell area. Indirect damage is caused when the radiation interacts with atoms and molecules within the cells to produced free radicals. These are highly reactive molecules that can cause damage to the cells and organs. An example of this interaction is when the radiation hits the water in the cells. This leads to ionising the water molecule to form: H2O + H2O+ (ion radical) + e (free radical). The H2O+ then reacts with other water molecules to form hydroxyl radicals (OH): H2O + H2O – H3O+ + OH. OH radicals cause two‐thirds of the damage to the cells and tissue. While free radicals become more reactive by oxygen, the damage to the DNA of cells becomes permanent. The effect of radiation on normal and malignant tissue is understood through the principle concepts of: repair, redistribution, reoxygenation, and repopoulation. Over the last few decades, there have been many improvements in radiotherapy. Advances in radiation planning and delivery have increased its efficacy while reducing toxicity [20]. Modern CT‐based radiotherapy planning relies on improved conformity of treatment portals and use of multiple treatment angles (Figure 4.8). Toxicity can be better predicted and reduced through volumetric calculation of normal tissue doses [21]. Figure 4.8 Using multiple ports of entry, crossfire builds up the dose of radiation to the deeply seated target (e.g. a bladder cancer), while sparing the surrounding tissues. Intensity‐modulated radiation therapy (IMRT) has developed tumour‐ and organ‐motion tracking techniques as well as innovations in target positioning. Image‐guided radiation therapy (IGRT) uses imaging carried out at each treatment session to allow millimetric adjustments in patient positioning. Techniques limiting respiratory organ motion – which applies even to the prostate – such as respiratory gating, adjustment of field sizes, and tumour tracking are increasingly being used. Precise target definition allows better normal tissue sparing and facilitates the safe delivery of higher doses and fractions (i.e. dose escalation), increasing the likelihood of cure. Adaptive radiation therapy (ART) uses a feedback process for dynamic treatment planning with each fraction. Radiotherapy can be given as percutaneous external beam radiotherapy (EBRT) or as an internal application of radioactive material which is inserted into the tumour or an organ (brachytherapy). The ability of radiation to penetrate tissues is related to radiation energy: the greater the energy the deeper the penetration. Radiation dose is measured in in terms of the energy absorbed during the interaction of radiation with tissue (1 Gy = 100 rad). There are differences in the effect of radiation from different energy sources. With conventional X‐rays, most of the absorption is at the surface of the skin; for gamma rays, the maximum absorption is about 5 mm below the surface. With linear accelerator megavoltage, the maximum absorption is about 20 mm below the skin surface (Figure 4.9). In bone, lower energy radiation produces a higher absorbed dose. Figure 4.9 With higher energy radiation, there is less absorption at the skin level. The results of percutaneous radiotherapy can be improved by additionally using radiosensitising chemotherapy (i.e. radiochemotherapy). The one definitive exception to radiotherapy in urological oncology is renal cancer, which is completely resistant to radiotherapy and chemotherapy. Radiotherapy has indications for the treatment of metastatic lesions, especially in the skeletal system, if metastatic bone lesions are painful or show signs of instability and are likely to lead to pathological fractures. Radiotherapy needs time for its effect on malignant cells. The most vulnerable stage of proliferating cancer cells to radiation is the M phase with rapid DNA synthesis, which is a tiny window in time during the mitotic cycle. Radiotherapy fractioning ensures that repetitive exposure destroys as many cancer cells as possible, allows normal tissue to recover, and reduce toxic side effects. Small volume irradiation is tolerated more than large. Radio‐resistance is related to the survival of CSCs as the source of continuous cancer evolution and plasticity. These can be considered a ‘moving target’ that can be hard to eradicate by radiotherapy [22]. The early morbidity after radiotherapy is the result of three processes: (i) acute inflammation (e.g. radiation cystitis), (ii) the death of stem cells, and (iii) the vascular response [21]. The early changes are most marked in the skin and the bowel where cell turnover is most rapid, but because enough stem cells remain, these epithelia usually recover. Late morbidity results from the aftermath of the inflammatory process, which includes fibrosis and changes within small blood vessels, which lead to ischaemia and sometimes necrosis (e.g. distal ureteral stenosis after radiotherapy for cervical cancer). Infertility can occur with doses as low as 3 to 6 Gy. A concern in long‐term survivors of radiotherapy is the potential of inducing second malignancies, the incidence rate for this risk is about 1% per year [23]. The normal kidney is rather radio‐sensitive. A dose of more than 2000 rad within five weeks will give rise to acute nephritis after several months, often leading to hypertension. Protection of the kidney is therefore vital. In the bladder, the sequelae of radiation vary and are largely unpredictable. An early ‘cute radiation cystitis may occur and can be made worse by infection [24]. Chronic radiation cystitis may occur after 6–24 months with sometimes severe symptoms and haematuria. Treatment is symptom based. Symptoms may subside, but often slowly get worse. In severe forms, it may be complicated by ischaemic necrosis with fistulae formation. Chemotherapy is treatment with drugs that interfere with cell replication (Table 4.1). Cancers with a fast proliferation rate are most sensitive to chemotherapy (e.g. testicular cancer). Table 4.1 Cycle action of common chemotherapeutics. Chemotherapy can be truly life‐saving as in testicular cancer with long‐term cure rates of 98–99% [25]. In other urological cancers, its role is adjunctive to surgery, where it may improve outcomes, or palliative as in metastatic urothelial and PCa. As cancer cells proliferate and divide more quickly than replicating normal tissues, chemotherapy affects cancer growth most. However, proliferating normal tissues (i.e. bone marrow, epithelium, and hair follicles) are also affected, resulting in loss of hair, mucosal erosions of the gastrointestinal tract, haematological toxicity with the reduction of all cellular blood elements, and loss of spermatogenesis. These toxic side effects are temporary and dose dependent. Lasting toxicity can also occur with some drugs (e.g. renal damage with cisplatin, peripheral neuropathy with vinblastine). Because of the effect on bone marrow, immunity against infection is also depressed. Severe leucopenia can result in life‐threatening septicaemia. Patients with leucopenia and fever require isolation, prophylactic antibiotics, and bone marrow stimulation by growth factors (e.g. filgrastim). Though single‐drug chemotherapy can be effective (e.g. docetaxel for castration‐resistant PCa), the effects are higher if several drugs with different mechanisms are combined (polychemotherapy) (Table 4.2). Chemotherapy needs to be given repeatedly to achieve a lasting effect. It is therefore given in treatment cycles or courses of usually 21 days, whereby treatment is given during the first few days and about two weeks are allowed for recovery. As part of a multimodal treatment, chemotherapy may be used before (i.e. neoadjuvant) or after (i.e. adjuvant) the primary treatment (surgery or radiotherapy) (e.g. chemotherapy before radical treatment of muscle‐invasive bladder cancer). Table 4.2 Mechanisms of action of commonly used chemotherapy drugs. The efficacy of chemotherapy in terms of response and survival is related to the sensitivity of the tumour but also to the patient’s general condition in terms of comorbidity and total tumour burden. The general condition can be assessed by the performance status (e.g. the Karnofsky score; Table 4.3) [26]. Table 4.3 Karnofsky performance scale. The effect of chemotherapy is assessed by the measurable response of lesions on imaging, usually CT scanning. A measurable shrinkage or disappearance of lesions is called a response, and a complete response is the disappearance of all measurable lesions for more than four weeks. Partial response (PR) is defined as a shrinkage of more than 50% for more than four weeks; if there is neither progression nor measurable shrinkage, this is called stable disease (SD). In the worst case, there is progression under chemotherapy (i.e. progressive disease [PD]). In the latter case, a change in the chemotherapy regimen is needed, whereas PR and CR indicate successful treatment. However, with PR and even with CR, the duration of these responses are decisive. Often, an SD response has to be considered a success because of a lack of more effective alternatives. Unique to urology is the intracavitary application of chemotherapy into the bladder, which is used in non‐muscle‐invasive bladder cancer as an adjunct to transurethral resection (e.g. mitomycin C). Advances in the understanding of the molecular biology of cancer have led to the development of drugs which affect specific intracellular pathways or effectors. Inhibitors of the tyrosine‐kinase mechanism (e.g. sunitinib and sorafenib) or the mTOR‐pathway (e.g. everolimus) are used in metastatic renal cancer to inhibit angiogenesis and slow down progression. In PCa, drugs targeting the androgen‐receptor (e.g. abiraterone and enzalutamide) can prolong survival in castration‐resistant disease. Immunotherapy is used or bladder, prostate, and renal cell cancers. The intravesical treatment with attenuated mycobacteria (BCG) is used as an adjuvant for high‐risk non‐muscle‐invasive bladder cancer and as the primary treatment for carcinoma in situ. In metastatic renal cancer, systemic immunotherapy with interferon and interleukin (often combined with fluorouracil) used to be the only systemic treatment before the introduction of targeted drugs with responses limited almost exclusively to pulmonary metastases. Recent advances have been made with the advent of drugs acting on programmed death ligand receptors which decrease the ability of a neoplasm to evade immune activity. These drugs, e.g. nivolumab,.can prolong survival considerably in metastatic renal and urothelial carcinoma. The intravenous injection of radionuclides can be used to treat bone metastases. In PCa, some radionuclides (e.g. strontium89) have an effect on bone pain but considerable bone marrow toxicity. Alpharadin (radium223) is a more targeted radionuclear treatment for metastatic bone lesions of PCa without bone marrow toxicity. Prostate‐specific membrane antigen (PSMA)–ligated radionuclides also act on soft tissue metastases in PCa. The combination of several treatment modalities should theoretically improve treatment outcomes. Locally advanced cancers, positive surgical margins, and the presence of regional lymph node metastasis often require multimodal treatment, combining surgery with chemotherapy or radiotherapy. Surgery and chemotherapy is often combined (e.g. in bladder cancer), but there are no established combinations of surgery and radiotherapy in uro‐oncology. For PCa, radiotherapy is often combined with hormone ablation. Multidisciplinary care of cancer patients by a team of surgeons, radiotherapists, medical oncologists, pathologists and psychologists as well as other specialists has become a standard in many countries. This is time‐consuming but is generally expected to improve patient care. Ideally, all members of an interdisciplinary care team should be specialised in uro‐oncology. Centralization of care of patients with certain cancer entities to high‐volume centres has been instituted in some countries (e.g. Great Britain, Sweden) and it is believed that this will improve outcomes. Better outcomes for surgery have been shown to depend on surgical volume for some procedures (e.g. radical cystectomy). Centralization also seems of importance for rare cancers (e.g. penile cancer).
Principles of Urologic Oncology
Abstract
4.1 The Scope of Urologic Oncology
4.2 Carcinogenesis
4.2.1 Genetics of Cancer
4.2.2 Mitotic Cycle
4.2.3 Genetic Instability of Cancer
4.2.4 Oncogenes and Tumour Suppressor Genes
4.3 Molecular Biology of Cancer Growth
4.3.1 Sustaining Proliferation
4.3.2 Evading Growth Suppressors
4.3.3 Resisting Cell Death
4.3.4 Enabling Replicative Immortality
4.3.5 Inducing Angiogenesis
4.3.6 Invasion and Metastasis
4.4 Tumour‐Promoting Inflammation
4.5 Reprogramming Energy Metabolism
4.6 Evading Immune Destruction
4.7 The Tumour Microenvironment
4.8 The Rate of Cancer Growth
4.9 Principles of Treatment
4.9.1 Diagnosing and Treating Solid Tumours
4.9.2 Estimating Prognosis by Staging, Risk Stratification, and Nomograms
4.9.3 When to Treat and When Not to Treat
4.9.4 Active Surveillance and Watchful Waiting
4.10 Oncologic Surgery
4.10.1 Surgery of the Primary Tumour
4.10.2 General Principles in Tumour Surgery
4.10.3 Regional Lymphadenectomy
4.10.4 Surgery for Metastatic Disease
4.10.5 Radiotherapy
4.10.5.1 Radiosensitivity of the Urogenital Tract
4.10.6 Chemotherapy
Cellular cycle phase specific
Gemcitabine
Hydroxyurea
Thiouracil/Fluorouracil
Methotrexate
Mercaptopurine
Vincristine/vinblastine
Docetaxel/paclitaxel
Cellular cycle nonspecific
Mitomycin C
Cisplatin
Carboplatin
Cyclophosphamide
Doxorubicin
Etoposide
Cellular cycle independent
Bleomycin
antimetabolites
(purine antagonists, pyrimidine antagonists, ribonucleotide reductase inhibitors)
6‐mercatopurine, methotrexate
5‐fluorouracil, methotrexate
hydroxyl urea
antagonists of DNA polymerase
cytarabine
alkylating agents interfering with DNA synthesis
n‐lost derivatives
nitrosoureas
platinum derivatives
mitomycin C
mitoxantrone
antagonists of topoisomerase
etoposide,
anthracyclines
mitoxantrone
irinotecan
protein degradation
asparaginase
inhibitors of mitosis
vinca alkaloids
taxanes
Normal: no complaints; no evidence of disease
100
Able to carry on normal activity; minor signs or symptoms of disease
90
Normal activity with effort: some signs or symptoms of disease
80
Cares for self: unable to carry on normal activity or do active work
70
Able to care for most self needs: requires occasional assistance
60
Requires occasional considerable assistance and frequent medical care
50
Disabled: requires special care and assistance
40
Severely disabled: hospitalisation is indicated although death not imminent
30
Very sick: hospitalisation necessary; active supportive treatment is necessary
20
Dead
0
4.10.7 Targeted Drugs
4.10.8 Immunotherapy
4.10.9 Radionuclide Treatment
4.10.10 Multimodal Treatment
4.10.11 Interdisciplinary Care and Centralization
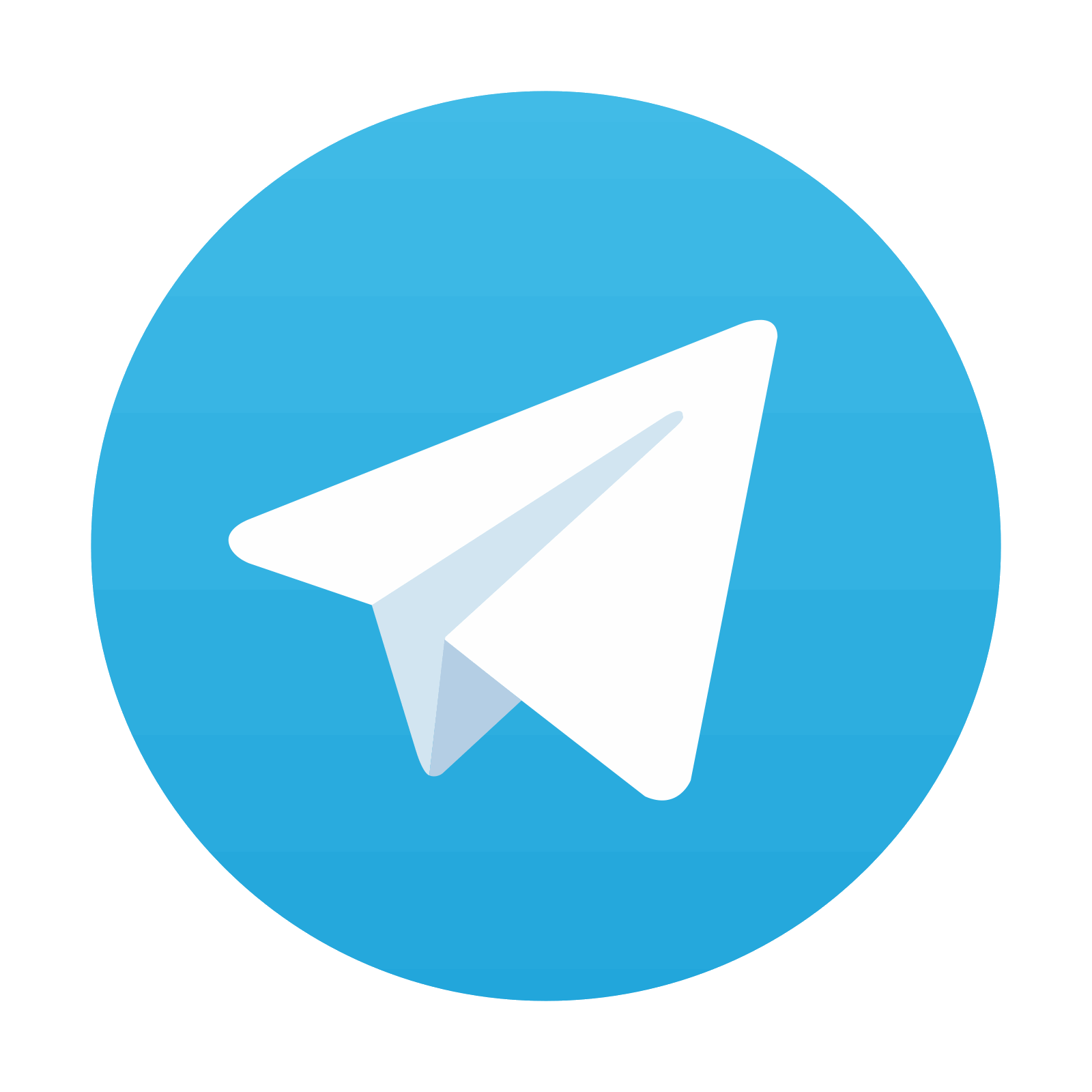
Stay updated, free articles. Join our Telegram channel
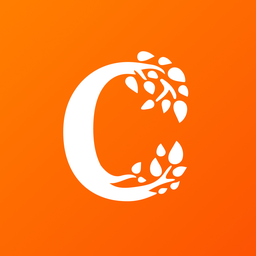
Full access? Get Clinical Tree
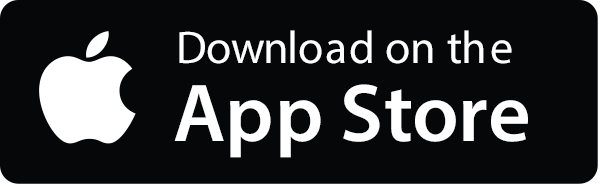
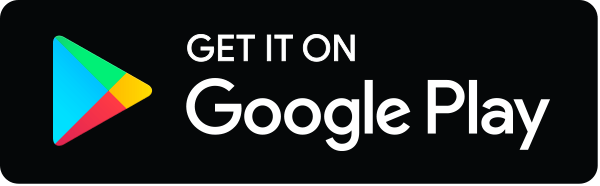