Abstract
In this chapter we review the physical, chemical, and clinical principles of hemodialysis as they relate to the treatment of uremia, starting with historical milestones and ending with projections for the future.
Keywords
adequacy, complications, dialyzer, epidemiology, hemodialysis, kinetics, Kt/V, mechanics, modalities, principles, residual syndrome, uremic toxins
Outline
Fundamental Concepts, 339
Uremia: the Target of Hemodialysis, 340
Dialysis, 344
Hemodialyzers, 345
Membrane Composition, Configuration, and Surface Area, 345
Effects of Flow on Clearance, 346
K O A, the Mass Transfer Area Coefficient, 347
Boundary Layers and Streaming Effects, 347
High-Efficiency and High-Flux Dialyzers, 347
Hemodialysis, 348
Types of Clearance, 348
Quantifying Hemodialysis, 350
Kt/V urea , 351
Filtration and Dialysis, 354
Middle and Large Molecule Removal, 355
Importance of Treatment Time, 356
Mechanics of Hemodialysis, 356
Dialysate Delivery Systems, 357
Blood Circuit Components, 358
Computer Controls, 358
Anticoagulation, 359
Online Monitoring of Clearance, Hematocrit, and Access Flow, 359
Future Considerations, 360
More than 2 million people require life-sustaining renal replacement therapy worldwide, including dialysis and transplantation. This dependence on an extracorporeal blood device is both the fulfillment of hopes by some and the dashing of dreams by others and highlights the need for an in-depth understanding of all aspects of hemodialysis, including the human reactions to it. Before one can configure hemodialysis optimally, one must understand its target, the uremic syndrome. In this chapter we review the physical, chemical, and clinical principles of hemodialysis as they relate to the treatment of uremia, starting with historical milestones and ending with projections for the future. The discussions include brief notes of comparison with other modalities, such as peritoneal dialysis and hemofiltration, that are reviewed more extensively in other chapters.
Fundamental Concepts
Historical Development
Hemodialysis was originally termed extracorporeal dialysis because it was performed outside the body. Several early pioneers laid the foundation for therapeutic dialysis. Graham (1805–1869), a professor of chemistry in Scotland, invented the fundamental process of separating solutes using semipermeable membranes in vitro and coined the word dialysis . In 1916 Abel dialyzed rabbits and dogs in the United States with a “vividiffusion” device using celloidin membranes and a leech extract called hirudin as an anticoagulant. Abel was the first to apply dialysis to a living organism and to use the term artificial kidney . In Germany, Haas first used the artificial kidney to dialyze a human in 1924. His attempts were only marginally successful because toxicity from his crude anticoagulant limited his ability to prolong flow in the extracorporeal circuit.
In view of these previous failures, it was not at all certain in 1944 that Kolff’s use of extracorporeal dialysis as a human lifesaving treatment for patients with kidney failure would be successful. Three major advances aided his efforts in the nearly 20 years since Hass’s work: the invention of cellophane, the discovery of antibiotics, and the availability of heparin as an anticoagulant. Through his keen interest in kidney failure and his aptitude for mechanics, Kolff and his patients ultimately met with success. Kolff is often called the “father of hemodialysis” because his method became accepted as the standard for temporary replacement of kidney function in patients with short-duration acute kidney failure.
Attempts to apply hemodialysis to patients who had more prolonged or permanent loss of kidney function were limited because the artery and vein used for blood access had to be tied off after each treatment. In 1960 Scribner, working with Quinton and Dillard at the University of Washington in Seattle, developed a blood access device for repeated dialysis using plastic tubes inserted into the artery and vein. This device, known as the Scribner shunt , and the more permanent arteriovenous (AV) fistulas later introduced by Brescia and Cimino allowed hemodialysis to be repeated for many years as a life-sustaining treatment. For their pioneering work in the field of artificial organs, Kolff and Scribner were granted the prestigious Lasker Clinical Medical Research Award in 2002.
Kidney Replacement Therapy
The goals of extracorporeal kidney replacement therapy include clearance of solutes and to maintain normal volume status. Several methods to achieve this exist, including hemodialysis, hemofiltration, and hemodiafiltration. The most successful intracorporeal modality has been peritoneal dialysis. The most promising replacement therapy is kidney transplantation because it can restore normal or near-normal kidney function, including potential functions not yet discovered, with the least inconvenience to the patient. The kidney is also an endocrine organ involved in the metabolism of hormones such as erythropoietin, calcitriol, prostaglandins, and renin. Overall care of the patient requiring renal replacement therapy also requires anticipation and management of problems; current approaches include attempts to reverse the psychological effects of kidney loss, preventing anemia and bone disease, monitoring the patient for signs of protein-energy wasting, monitoring the function of peripheral AV access devices, expecting hypotension during dialysis in patients with concentric ventricular hypertrophy, adjusting medication doses appropriately, and monitoring the quality of dialysate water (detailed in various chapters). Clinical practice guidelines and practical recommendations have been published and are now available in several countries, each with the ultimate goal of improving quality of life.
Definitions
Dialysis is the passage of molecules in solution by diffusion across a semipermeable membrane. Essential elements of this process are the solvent, which contains dissolved solutes, and the membrane that contains pores through which some or all of the solutes move by diffusion ( Fig. 22.1A ). The molecular kinetics of diffusion are both solute and membrane specific. Solute characteristics that affect movement across a particular membrane include its concentration, molecular weight, shape, charge, and lipid solubility. Membrane characteristics that determine permeability to a particular solute include the average effective pore size; the number, geometry, and distribution of pores within the membrane; membrane surface area and thickness; and surface characteristics, such as charge and hydrophilicity. The solvent itself may also move by diffusion if its chemical activity is not balanced across the membrane. Although solutes may move in both directions across the membrane, it is customary to refer to the compartment containing more vital substances that one wishes to preserve as the dialyzed compartment and to the solution in the other, usually larger, compartment as the dialysate.

The concept of molecular diffusion is critically important to the definition of dialysis. Solutes pass through the membrane down an electrochemical gradient caused primarily by a difference in concentration across the membrane (see Fig. 22.1A ). This concentration gradient, which is the driving force for diffusion, may also be dissipated by the dialysis (i.e., the molecular concentration gradient tends to fall with dialysis).
In the absence of an electrochemical gradient, solutes may also pass through pores in the membrane by filtration, a process of convection. The driving force for filtration is pressure, either hydraulic or osmotic, that is unbalanced across the membrane ( Fig. 22.1B ) and independent of dialysis. During filtration, solute passively accompanies the solvent from one compartment to the other, causing no change in solute concentration. Convective movement may occur in the opposite direction to diffusive movement, and even in the same direction, convective movement may interfere with dialysis (i.e., the two fluxes may not be additive when they occur simultaneously).
Hemodialysis means literally “dialysis of the blood.” This form of dialysis is distinguished by its location outside the body and by the continuous flow of blood across the dialyzer membrane. Therapeutic hemodialysis is most often used to treat kidney failure by equilibrating the blood against an isoosmotic dialysate. Vital solutes are added to the dialysate at concentrations designed to mimic those normally maintained in the blood by the native kidney ( Table 22.1 ; see Fig. 22.1A ). The resulting dialysate is essentially a physiological salt solution that, in addition to creating a gradient for removal of unwanted solutes, reproduces another vital function of normal kidneys, that of maintaining a constant physiological concentration of extracellular electrolytes.
Component | Concentration (mEq/L) |
---|---|
Sodium | 135–145 |
Potassium | 0–4.0 |
Chloride | 102–106 |
Bicarbonate | 30–39 |
Acetate | 2–4 |
Calcium | 0–3.5 |
Magnesium | 0.5–1.0 |
Dextrose | 11 |
pH | 7.1–7.3 |
Uremia: the Target of Hemodialysis
Uremia is the clinical state or syndrome that is reversed by dialysis therapy, and it literally means “urine in the blood.” Whether or not urine output falls, all patients with uremia accumulate solutes, collectively known as uremic toxins. It is this accumulation of solute, the most abundant of which is urea, that prompted the application of dialysis as a treatment for uremia. From another perspective, the concept of uremia as a state of intoxication by substances normally eliminated by the kidney is supported by the success of therapeutic dialysis. The kidney separates large from small solutes by filtration, powered by the heart and accomplishing much the same function as the dialyzer, which also depends on cardiac output to deliver solute for elimination but is not powered by the heart. Some have likened dialysis to the original elimination mechanism of organisms that evolved in the infinite “dialysate” of the primordial sea. Conserved adaptation to diffusive removal of solute might explain the marked success of therapeutic dialysis compared with other artificial organ replacements.
Clinical Syndrome
Although not all patients exhibit all the symptoms and signs of uremia, the monotony of the clinical syndrome in patients with widely divergent causes of kidney failure indicates that the syndrome is the consequence of the kidney failure per se, not the underlying disease. Nearly every organ system is involved, but the most highly targeted are the gastrointestinal tract and the central nervous system. Early symptoms include dysgeusia, loss of appetite, nausea, weight loss, inability to concentrate on a mental task, lethargy, daytime sleepiness, pruritus, and menstrual irregularity in women. Unfortunately, these symptoms are not specific and are sometimes mistaken for an unrelated infection or can be confounded by coexisting anemia. They appear in most patients only at an advanced stage of kidney damage (80% to 90% loss of nephrons). Far advanced symptoms and signs include uremic serositis with pericarditis, once the harbinger of death as a result of uremia; central nervous system suppression leading to uremic coma; overt peripheral neuropathy; and uremic fetor caused by volatile amines emitted in the breath. Fluid accumulation, which is subtle in most patients, contributes to hypertension that eventually leads to cardiac hypertrophy and diastolic dysfunction.
Uremic Toxins
The most life-threatening solutes known to accumulate in uremia ( Table 22.2 ) are low in molecular weight and consequently are dialyzable. A host of other substances have been found to accumulate in renal failure, and data on their potential toxicity are emerging. As the list of uremic retention solutes grows steadily, approaches to analyze these solutes more systematically are underway and realization that a significant number of uremic retention solutes may not be amenable to removal with conventional dialysis is dawning ( Fig. 22.2 ).
Solute | Proposed Toxicity |
---|---|
Free Water Soluble, Low Molecular Weight | |
Sodium | Volume overload |
Potassium | Arrhythmia, muscle weakness |
Hydrogen ion (metabolic acidosis) | Degrades protein (activates ubiquitin proteasome); alters vitamin D and parathyroid hormone levels |
Urea | None |
Creatinine | None |
Guanidines | Immune dysfunction; neurotoxicity |
Oxalate | Tissue deposits; inhibits endothelial cell replication and migration |
Asymmetrical dimethylarginine | Cardiovascular disease; inhibits inducible nitric oxide synthase |
3-carboxyl-4-methyl-5-propyl-2-furanproprionic acid | Displaces drugs bound to albumin; inhibits erythropoiesis; inhibits mitochondrial oxidation |
4-hydroxybenzoic acid (phenolic acid) | Platelet dysfunction; shortened red cell survival; neurological symptoms |
Trimethylamine-N-oxide (TMAO) | Cardiovascular disease |
Protein Bound | |
Hippuric acid | Muscle weakness; neurological symptoms; decreases drug binding to albumin |
Indoxyl sulfate | Displaces drugs bound to albumin; oxidative stress; cardiovascular disease |
p -Cresol | Immune dysfunction; cardiovascular disease; oxidative stress |
Quinolinic acid | Inhibits erythropoiesis; seizures in mice |
Homocysteine | Cardiovascular disease |
Pentosidine | Cardiovascular disease |
Leptin | Cardiovascular disease |
Sequestered | |
Phosphate | Osteodystrophy; cardiovascular disease |
Magnesium | Muscle weakness |
Middle (500–5000 Da) and High (5000–50,000 Da) Molecular Weight | |
Parathyroid hormone | Inhibits mitochondrial oxidation; hypertrophic cardiomyopathy; cardiac fibrosis; immune dysfunction |
β 2 -Microglobulin | Dialysis amyloidosis |
Carbamylated proteins | Possible cardiovascular disease |
Advanced glycation end products | Dialysis amyloidosis; cardiovascular disease; oxidative stress |

Despite its importance as a measure of dialysis adequacy, urea itself has been found to have little toxicity, as reported in experiments where toxicity was alleviated despite addition of urea to the dialysate to prevent its removal. Similarly, although all other solutes mentioned accumulate in patients with kidney failure, their levels are well less than that necessary to evoke toxic responses in animals and in humans, even when measured in patients with end-stage renal disease (ESRD). Even after decades of research, investigators are unable to identify a single toxin or a group of toxins responsible for the immediate life-threatening uremic syndrome that is quickly reversed by dialysis. Because dialysis does little more than remove fluid and dialyzable solutes, the uremic syndrome must result from accumulation of known and unknown toxins in aggregate, perhaps each at subtoxic levels.
Residual Syndrome
It is now clear that the amount of dialysis necessary to sustain life is not enough to maintain a high quality of life. A challenge to investigators is the development of techniques for analyzing and treating this “residual syndrome,” which affects some patients more than others but reduces the quality of life despite apparently adequate dialysis. Several components of the syndrome can be identified, such as anemia, osteodystrophy, dialysis amyloidosis, accelerated atherosclerosis, anorexia, disordered sleep, and fatigue, some of which are treatable (see relevant chapters). The proposed uremic toxins discussed previously may account, in part, for the residual syndrome, but almost certainly other components remain to be defined. These components include (1) the causes of inflammation and malnutrition and the interactions among malnutrition, inflammation, and atherosclerosis in dialysis patients ; (2) the cause of accelerated atherosclerosis ; (3) the role of hyperhomocysteinemia in accelerated atherosclerosis and graft thrombosis ; (4) the role of hyperphosphatemia in cardiovascular disease ; and (5) the role of endothelial dysfunction as a mediator of cardiovascular disease. Other proposed underlying causes of the residual syndrome include accumulation of toxins that are poorly dialyzable because of their larger molecular size, protein binding in the blood or tissues, and sequestration with slow release from compartments other than the blood. A complete understanding of the basis for the residual syndrome remains elusive, but several concepts are emerging, based on evidence from clinical studies. The Hemodialysis (HEMO) Study (see Goals of Hemodialysis) found that increasing the clearance of middle molecules (MMs) such as β 2 -microglobulin using high-flux dialyzers did not alter survival in hemodialysis patients. Subsequent studies reported that the clearance of some protein-bound solutes can be increased by increasing both dialyzer surface area and dialysate flow, postdilution online hemodiafiltration, addition of sorbent to dialysate, and fractionated plasma separation and adsorption. Increasing the frequency of dialysis to 6 days per week may also improve weekly removal of larger or sequestered molecules. An interesting study also found that increased dietary fiber reduces levels of some colon-derived protein-bound solutes in hemodialysis patients. Despite many such developments in our understanding of the contributors to the residual syndrome, clinical outcome studies on the impact of reducing these various uremic toxins are either limited or nonexistent.
Goals of Hemodialysis
The primary goal of hemodialysis is the replacement of kidney excretory function. There is no doubt that hemodialysis can sustain life in patients who have no kidney function. Survival for as long as 30 years has been documented for hemodialysis alone, a treatment that does nothing more for the patient than remove solute. Earlier experience indicates that the most life-threatening toxins are easily dialyzable. Precise goals and standards of dialysis adequacy have been defined, based on outcome studies in large populations, in terms of the clearance of small-molecular-weight, easily dialyzed solutes, the marker for which is urea.
A prospective interventional study of dialysis adequacy in the late 1970s, the US National Cooperative Dialysis Study (NCDS), provided clear-cut evidence for a level of urea clearance that was inadequate. Later uncontrolled experience suggested that more dialysis might be better for the patient. Analysis of solute kinetics, however, indicated that the benefit of dialysis is logarithmically related to the dose and that, depending on the frequency of treatments, a point is reached beyond which more dialysis does nothing more than inconvenience the patient, potentially worsening the quality of life. This theoretical construct was confirmed by the National Institutes of Health (NIH)–sponsored HEMO Study, a multicenter prospective clinical trial that randomly assigned 1846 patients to receive thrice weekly hemodialysis with a target equilibrated Kt/V (where K is dialyzer clearance of urea, t is dialysis time, and V is volume of distribution of urea) of 1.05 (equivalent to single-pool Kt/V of 1.25, the generally accepted minimal standard at the time of the study) versus 1.45 (equivalent to single-pool 1.65). After the publication of uncontrolled studies indicating possible beneficial effects of more frequent dialysis on several outcomes, such as blood pressure, patient-reported outcomes, and laboratory parameters, a randomized trial was needed to definitively determine the optimal dose of hemodialysis. This led to the Frequent Hemodialysis Network (FHN) trial, sponsored mainly by the National Institute of Diabetes and Digestive and Kidney Diseases and the Centers for Medicare and Medicaid Services (CMS). The trial compared standard three-times-a-week hemodialysis to six-times-a-week hemodialysis. The frequent hemodialysis group (which had a standard Kt/V [see later] of 3.54 compared with the standard group Kt/V of 2.49) had beneficial effects on the composite endpoint of death or change in left ventricular mass. The FHN trial also included a nocturnal hemodialysis arm, which achieved a standard Kt/V of 4.5. Patients in the nocturnal group had better control of hyperphosphatemia and blood pressure, but the trial failed to find a beneficial effect on either of the two coprimary endpoints of death or left ventricular mass. One should also bear in mind that more frequent hemodialysis has potential adverse effects as well, such as more frequent vascular access procedures, more rapid loss of residual kidney function, and both patient and caregiver burnout. Long-term posttrial follow-up of the two FHN trials were published in 2015 and 2016, reporting a significant reduction in mortality in patients randomly assigned to frequent in-center hemodialysis but a surprisingly increased mortality risk in the patients randomly assigned to the home nocturnal arm in the FHN Nocturnal study. For now, the dose of dialysis as measured by single-pool Kt/V (sp Kt/V [see later]) of at least 1.2 per dialysis is recommended for three treatments per week, and this is also a CMS clinical performance measure of dialysis facilities. However, the ultimate goal of dialysis should not rely solely on achievement of a “universal target” of Kt/V but also take into account adequate control of uremic symptoms, achievement control of fluid balance and blood pressure, resolution of the “residual syndrome,” and prolonging survival while achieving quality of life for the patient.
Dialysis
As defined previously, dialysis is a process of diffusion of molecules in solution across a semipermeable membrane. Forces that govern the pattern and rate of diffusion have been defined in precise mathematical terms that include properties of the molecule, the solvent, and the membrane. The salient points of the physics applicable to dialysis are discussed here because a detailed analysis is beyond the scope of this chapter. For detailed analysis of the physical laws that govern dialysis, the reader is referred to formal texts on kinetic modeling.
Laws of Diffusion
Diffusion is a consequence of random molecular movements (molecular kinetics) that follow the laws of probability and are driven by temperature, pressure, and concentration. Because temperature and pressure are relatively constant during therapeutic dialysis and among dialysis centers (see later), the major clinical variable that affects diffusion is the solute concentration. Fick’s law of diffusion, derived from mathematical laws of statistical probability, indicates that the rate of diffusion is linearly dependent on the concentration gradient (i.e., the driving force for diffusion):
− j = ( DA / X ) Δ C
Dividing both sides of Eq. 1 by ΔC results in an expression for solute dialysance :
− J / Δ C = dialysance = DA / X
− J / C = clearance = K
If the volume of the dialyzed compartment (V) is constant, dividing both sides of Eq. 3 by V shows that the fractional rate of change in concentration is constant:
− ( J / V ) C = K / V = K
− ( dC / C ) / dt = K / V = K
Eq. 5 indicates that concentration-dependent diffusion is a first-order process; that is, despite the minute-to-minute changes in concentration within the dialyzed compartment, the fractional rate of change is constant when the dialysate concentration remains zero. Flux of solute across the membrane, which is the goal of dialysis, is both driven by the concentration and expressed as a change in concentration. When the rate of change is factored by the driving force ([dC/dt]/C), the resulting fractional rate of change is constant.
The rate constant (k) in Eq. 5 has units of time –1 or a fraction per unit of time and is a function of both the molecular properties of size, shape, charge, and interaction with the membrane and of the membrane itself, including its surface area, porosity, and thickness. Large molecules, those with complex shapes, and those with an electrical charge diffuse less readily across the membrane. Membranes that are more porous, have a larger surface, and are thinner favor passage of solutes by diffusion. Although the rate constant is useful to demonstrate the first-order concept, it has less practical value than the expression for clearance depicted in Eq. 3 .
The difference between the rate constant (k) in Eq. 4 and the clearance (K) shown in Eq. 3 is V, the volume of solute distribution. Eq. 3 has the advantage of expressing the dialysis effect as a volume equivalent of solute diffusing across the membrane per unit of time. The volume transferred per unit of time is constant; that is, a milliliter equivalent of solute is transferred per unit of time regardless of how much solute is contained in that milliliter. The rate of diffusion is directly proportional to the membrane surface area, which is constant for any given model of dialyzer.
Effects of Temperature, Pressure, and Molecular Weight
Diffusion is a consequence of molecular motion, which is affected by pressure and heat energy and by molecular mass. The rate of diffusion is proportional to the absolute temperature, which is approximately 273°K at room temperature. Within the range of temperatures experienced in the dialysis center, the proportionate change in absolute temperature (260°K to 280°K) is so small that its influence on diffusion across the dialysis membrane is negligible. More important are the physiological effects of temperature on blood flow and body water compartmentalization, which have significant effects on solute kinetics within the patient (see Quantifying Hemodialysis). Similarly, pressure effects have little influence on diffusion within the range of pressures recorded in modern dialyzers.
Molecular mass plays a more significant role in determining the rate of diffusion because at a given temperature and pressure, the heavier molecules move more slowly and collide with the semipermeable membrane less frequently. Small-molecular-weight substances, such as urea and creatinine, diffuse readily across a semipermeable membrane, whereas larger substances, such as β 2 -microglobulin or albumin, diffuse slowly or not at all. The larger size of the heavier molecules further impedes diffusion through small pores.
Dialysate
Preparation of the dialysate and the resulting composition are critical to the success of dialysis. For hemodialysis the solution must be prepared from properly treated water and contain the solutes listed in Table 22.1 in concentrations comparable to those of plasma. Dialysate must have a low concentration of endotoxin to prevent pyrogen reactions in the patient, but, in contrast to peritoneal dialysate, sterility is not a requirement because the semipermeable membrane excludes large particles, such as bacteria and viruses. Vital electrolytes and glucose are added to the dialysate to reduce or abolish their concentration gradients, whereas bicarbonate or a bicarbonate precursor is added in higher concentrations to promote accumulation in the patient. Dialysate glucose concentrations are near those of plasma; thus in contrast to peritoneal dialysis, osmotic forces do not play an important role in removing fluid.
In practice, solute concentrations in the dialysate are fairly standard. The most common concentrations that may be individualized are those for potassium, calcium, and bicarbonate (see Table 22.1 ). In many dialysis centers, the bicarbonate concentration is fixed at 35 or 39 mEq/L, although many centers may err on the side of a lower dialysate bicarbonate concentration, after the observation of higher all-cause mortality with higher dialysate bicarbonate concentrations. One should also take into account the contribution of the acid concentrate (acetate) to the total alkali exposure to the patient during a dialysis session, given the conversion of the acetate to bicarbonate. Potassium ranges from 0 to 4 mEq/L, depending on the patient’s serum concentration before dialysis. A compelling reason must exist, however, to use dialysate potassium concentrations of 0 or 1 mEq/L because of the dangers associated with a precipitous drop in the serum concentration. In particular, patients on digoxin must be dialyzed against at least 2 mEq/L of potassium. Calcium concentrations vary from 1 to 3.5 mEq/L. At the lower concentration, calcium is removed from the patient, whereas at the higher concentration, calcium diffuses into the patient during dialysis. (A calcium concentration of 2.5 mEq/L is equal to 5 mg/dL, which is roughly the amount of ionized calcium in plasma—equaling about 10 mg/dL of total calcium.) The concentration of sodium had until recently been fixed at 140 mEq/L, which is the middle of the normal range in whole plasma. More recently, with evidence suggesting that lowering the dialysate sodium level to the range of 134 to 138 mEq/L may be beneficial and avoid intradialysis sodium loading, an increasing number of dialysis facilities are using 135 to 137 mEq/L as the standard dialysate. Results of ongoing clinical trials will hopefully clarify the optimum dialysate sodium levels.
Hemodialyzers
A hemodialyzer, synonymous with dialyzer, is often called an “artificial kidney.” It is configured to allow blood and dialysate to flow, preferably in opposite directions, through individual compartments, separated by a semipermeable membrane. By convention, blood entering the hemodialyzer is designated arterial, whereas blood leaving the hemodialyzer is venous. The principal differences among the many available hemodialyzers are the membrane composition, membrane configuration, and membrane surface area. Hemodialyzers affect the efficiency and the quality of dialysis by virtue of their membranes, which determine their K O A value, and by the rates of blood and dialysate flow, which determine their clearance values (see later discussion of K O A) ( Box 22.1 ).
Properties of the membrane
↑ Membrane porosity
↓ Membrane thickness
↑ Membrane surface area
Properties of the solute
↓ Molecular weight and size
↓ Shape
↓ Charge
Blood side
↓ Unstirred blood layer
↑ Blood flow
Dialysate side
↓ Dialysate channeling and unstirred layer
↑ Dialysate flow
↑ Countercurrent direction of flow
↑, Increases clearance; ↓, decreases clearance.
Membrane Composition, Configuration, and Surface Area
Composition of the Membrane
Two major classes of membrane material are available commercially: (1) cotton fiber, or cellulose-based membranes, and (2) synthetic membranes. Cellulose-based membranes range from unmodified cellulose to substituted cellulose membranes. Unmodified cellulose membranes have many free hydroxyl groups, which are thought to be responsible for their bioincompatibility and propensity to activate white blood cells, platelets, and serum complement. In an effort to improve membrane biocompatibility while keeping costs down, the cellulose polymer is treated with acetate and tertiary amino compounds to form a covalent bond with the hydroxyl groups (e.g., cellulose acetate and animated cellulose [Cellosyn or Hemophan]).
The major polymers in commercial synthetic membranes are polyacrylonitrile, polysulfone, polycarbonate, polyamide, and polymethylmethacrylate. Despite their increased thickness, these membranes can be rendered more permeable than the cellulose membranes, allowing for greater fluid and solute removal. They are also more biocompatible. Because the pore sizes in the synthetic membranes can be made wider, larger-molecular-weight substances, such as β 2 -microglobulin, can be removed more efficiently. High-flux synthetic membranes also clear phosphate more efficiently, although the effect on serum phosphate levels is minimal. Despite their higher cost, synthetic hemodialyzers are increasingly preferred.
Hollow-Fiber Dialyzers
Current hemodialyzers are constructed with a plastic casing, usually polycarbonate that encloses several thousand hollow-fiber semipermeable membranes stretched from one end to the other, embedded at each end into a plastic potting compound, usually polyurethane, that serves as the headers.
The blood compartment fiber bundle volume of the hollow-fiber dialyzer is 60 to 120 mL, which, in contrast to older dialyzer designs, does not expand during dialysis. Each fiber has an inside diameter of approximately 200 μm. The potting material separates the blood compartment from the dialysate compartment where dialysate flows between and around each fiber in the direction usually opposite to blood flow. Blood flows to or from the open end of each fiber through a removable header attached to the blood tubing. In addition to a lower blood-priming volume, the hollow-fiber design increases the area of contact between blood and dialysate, allowing for the most efficient exchange of solutes. Recent efforts to prevent loss of surface area by fiber-to-fiber contact include insertion of spacer yarns between fibers and a wavy moiré configuration of the fibers. Major disadvantages of the hollow-fiber design are thrombosis and the potting compound, which can absorb chemicals used to disinfect newly manufactured dialyzers (e.g., ethylene oxide) or reused dialyzers (e.g., formaldehyde, peracetic acid, or glutaraldehyde). These chemicals can leach slowly from the material and potentially enter the patient’s blood during dialysis.
Surface Area Considerations
Most hemodialyzers have a membrane surface area of 0.8 to 2.1 m 2 . As the area increases, solute transport, often called efficiency of the dialyzer , increases. To maximize membrane surface area, one can increase the length of the hollow fiber, increase the number of hollow fibers, or decrease the diameter of the hollow fiber while holding other parameters constant. Each of these maneuvers, however, has undesirable effects when carried too far. Increasing the fiber length increases shear rate and magnifies the pressure drop between blood entering and exiting the dialyzer. Increased shear rate increases ultrafiltration, whereas the pressure drop decreases ultrafiltration because the transmembrane pressure (TMP) gradient dissipates at the venous end of the dialyzer. Any decrease in ultrafiltration decreases its contribution to solute clearance and offsets the potential advantage of the increased surface area. Increasing the number of hollow fibers increases the volume of extracorporeal blood and may eventually compromise hemodynamic stability. Finally, as the diameter of the hollow fiber decreases, the increase in resistance to blood flow enhances filtration and backfiltration, but clotting is also enhanced. As fibers thrombose, effective surface area for diffusion decreases and solute clearances fall. Because of these adverse consequences, the minimal acceptable internal fiber diameter is 180 μm. The design and geometry of the hollow-fiber dialyzer represent a delicate balance among these factors.
The composition and the thickness of the membrane varies considerably and is often more important than the surface area in determining dialyzer efficiency. In general the thinner the membrane, the more efficient the transport of solutes and fluid across the membrane.
Effects of Flow on Clearance
Blood Flow
Dialyzer blood flow (Q b ) is driven by a roller pump and generally ranges from 200 to 500 mL/min, depending on the type of vascular access. Blood flow influences the efficiency of solute removal (see Box 22.1 ).
As Q b increases, more solute is presented per unit of time to the membrane, and solute removal increases. Urea removal rises steeply as Q b increases to 300 mL/min, and although urea removal continues to rise as Q b approaches 400 to 500 mL/min, the slope is less steep. For larger-molecular-weight substances, removal is slower and more time dependent rather than flow dependent because diffusion across the membrane is limited, as discussed previously.
Dialysate Flow
Most dialysis equipment generates a single pass of dialysate; that is, the dialysate is discarded after one passage through the dialyzer. For sorbent dialysis, however, only about 5 L of water are used and dialysate is constantly regenerated by cycling through a cartridge system to remove the undesirable solutes (e.g., urea, creatinine, and potassium).
Countercurrent flow maximizes the concentration gradient between blood and dialysate throughout the length of the dialyzer (see Box 22.1 ). When blood flow and dialysate flow are in the same direction (cocurrent), small solute clearance decreases by about 10%. In addition to decreasing boundary layers and streaming effects (see later discussion), increasing Q d minimizes the accumulation of waste products in the dialysate, providing a higher solute gradient between blood and dialysate for optimal diffusion. However, even for highly diffusible solutes, the benefits progressively diminish as the dialysate flow rate is increased higher than the blood flow rate. In fact, increasing dialysate flow rate beyond 600 mL/min offers no additional benefit to delivered Kt/V urea .
K O A, the Mass Transfer Area Coefficient
K O A is the product of the mass transfer coefficient, K O , which has units of centimeters per minute, and the membrane area, A. K O is specific for a particular molecule and membrane type, including the membrane’s pore size and thickness, but is independent of solute concentration and membrane surface area. It can be considered the solute flux per unit of area per unit of concentration gradient and is equivalent to D/X in Eq. 2 . Because solute flux per unit of concentration gradient is defined as dialysance, K O may also be expressed as the dialysance per unit of membrane area. K O A, which is the mass transfer area coefficient, therefore has units of milliliters per minute and is equivalent to the dialysance of a membrane with a fixed area during static dialysis (no flow). In addition to being independent of solute concentration, K O A is also independent of blood and dialysate flow within certain limits (see later text). Therefore K O A is the most specific constant that describes the efficiency of a dialyzer for removal of a particular solute and is the best parameter for comparing dialyzers. Higher values indicate more efficient solute removal.
K O A has the same units of measurement as clearance and, in practical terms, can be considered the maximum clearance achievable for a particular dialyzer and solute. Maximum clearance is achieved at the beginning of dialysis when blood solute concentrations along the length of the dialyzer are equal (no flow) and dialysate concentration is 0 or, at the opposite extreme, when blood and dialysate flow rates are infinite. Under these two conditions, the only factor governing a solute’s clearance is the dialysis membrane.
Conversely, when Q b and Q d are finite, the clearance is lower than K O A because both flow rates govern diffusion, as discussed previously and because of the way clearance is expressed, as the solute removal rate divided by the inflow concentration. The net driving force for removal is the mean concentration gradient across the membrane, which is a complex function of Q b and Q d (see next section). The increase in clearance caused by an increase in Q b is the result of a flow-dependent increase in the mean concentration gradient across the membrane, driving more solute into the dialysate. Because the inflow concentration does not change with increased Q b , the conventional measure of clearance as defined previously increases with increasing Q b .
Boundary Layers and Streaming Effects
Despite a rapid flow along the membrane, the solvent tends to adhere to the membrane, creating a boundary layer, or unstirred layer, that adds to the diffusive pathway on both sides of the membrane. This layer of solvent adjacent to the membrane tends to thin out as flow is increased or as turbulence is produced at the membrane surface. In addition to forming boundary layers, dialysate tends to move along the path of least resistance or channel, leading to nonuniform flow and bypassing some of the membrane area. This streaming effect is more pronounced at lower dialysate flow rates, especially in large dialyzers. Both boundary layer and streaming effects cause K O A (the resistance to solute diffusion across the membrane) to increase as dialysate flow increases, although the effect is less in vivo than in vitro. Recent changes in the shape of hollow fibers and the insertion of inert spacer yarns have improved dialyzer performance further through reducing the effects of channeling and unstirred layers. Both effects are less prominent on the blood side of hollow fibers because of the geometrical advantages of flow within hollow fibers, the scrubbing effects of red blood cells, and less variance in Q b.
High-Efficiency and High-Flux Dialyzers
Initial hemodialyses were limited by low dialyzer membrane permeability, requiring more than 6 hours for each treatment. Although treatment times were shortened to 4 hours or less three times a week as dialyzer design improved, the time spent attached to the dialysis machine was still unacceptable to many patients. The next major advancement came in the late 1980s, when the technical problems with bacteriological contamination of bicarbonate dialysate, inadequate blood flow, imprecise ultrafiltration control, and continued low dialyzer solute clearance were solved.
The distinction between high-efficiency and high-flux dialyzers is not always made clear, and sometimes these terms are used interchangeably. In essence, both terms address improved solute and fluid clearance compared with standard hemodialyzers, taking advantage of higher blood and dialysate flow rates to decrease dialysis time while maintaining an adequate dose. The two dialyzer designs are not mutually exclusive and in fact often overlap ( Table 22.3 ). The high-efficiency dialyzer contains either a synthetic or a modified cellulose membrane and has a higher clearance of small molecules, such as urea, compared with a standard dialyzer. The high-flux dialyzer always has a highly permeable synthetic or modified cellulose membrane that removes larger molecules. By their nature, high-flux dialyzers have a higher ultrafiltration coefficient (K Uf ) compared with high-efficiency dialyzers but not necessarily high urea clearances. Conversely, high urea clearance defines high-efficiency dialysis, but the clearance of larger molecules is variable (see Table 22.3 ).
Standard | High Efficiency | High Flux | |
---|---|---|---|
Blood flow rate (mL/min) | 250 | ≥350 | ≥350 |
Dialysate flow rate (mL/min) | 500 | ≥700 | ≥700 |
K o A urea | 300–500 | 600–1000 | Variable |
Urea clearance (mL/min) | <200 | 250–400 | Variable |
Urea clearance/body weight (mL/min/kg) | <3 | >3 | Variable |
Vitamin B 12 clearance (mL/min) | 30–60 | Variable | >100 |
Ultrafiltration coefficient (mL/h/mmHg) | 3.5–5.0 | <15 | >15 |
Membrane | Cellulose | Variable | Variable |
The advent of substituted cellulose and synthetic membranes improved dialyzer permeability because substituted cellulose membranes can be made thinner to increase porosity and surface area, whereas synthetic membranes can be manufactured with more and larger pores. Both high-efficiency and high-flux dialysis require the use of bicarbonate dialysate and volume-controlled filtration. Because the high-efficiency and high-flux dialyzers have a higher K Uf , precise control of ultrafiltration is also mandatory to prevent massive volume depletion (see Mechanics of Hemodialysis).
Because of their greater porosity, high-flux dialyzers can remove larger molecules. Use of these membranes to improve β 2 -microglobulin removal has been associated with a reduction in the risk for carpal tunnel syndrome in long-term patients treated with high-flux dialysis. Other benefits that derive possibly from removal of large molecules are an improved lipid profile, a greater response to erythropoietin, and perhaps lower mortality and hospitalization rates. Potential adverse consequences from increased removal of larger molecules, however, include greater removal of drugs such as vancomycin, amino acids, and albumin, although the last mentioned is disputed. The presence of backfiltration during high-flux dialysis has been postulated to increase the risk for exposing patients to endotoxin from the dialysate, although this potential problem has not been clearly identified in clinical studies. One other potential issue with high-efficiency dialyzers is that even at low blood flows, urea clearances are substantial compared with clearances achieved with low-efficiency membranes, which might pose a risk to critically ill acute-dialysis patients because of rapid removal of urea.
Only a few studies have evaluated the comparative efficacy of standard dialysis versus high efficiency and high flux. Nearly all high-flux dialyzers also have high efficiency, so most studies focus on high-flux versus standard hemodialysis. Randomized controlled or crossover trials using bicarbonate dialysate found no difference in the incidence of hypotension and intradialysis symptoms or in the control of blood pressure among the three modalities. In a small number of patients treated with high-flux dialysis versus standard dialysis, neuropsychological function was comparable. The best data available come from the HEMO Study, which detected no significant difference in mortality or morbidity between patients treated with standard versus high-flux dialysis and only a slight difference in subgroup analyses. A smaller study in Europe (the Membrane Permeability Outcome Study) found a small but significant improvement in mortality but only in the subgroup with low serum albumin levels (<4.0 g/dL). A Cochrane review of high-flux versus low-flux membranes concluded that high-flux membranes had no effect on all-cause mortality but may reduce cardiovascular mortality.
Hemodialysis
Using dialysis as a form of therapy for the patient vastly complicates this otherwise simple procedure. Factors that complicate the delivery of dialysis include the access device, the patient’s adherence with the dialysis prescription and diet, and solute disequilibrium. Developing standards of adequacy requires detailed studies of large populations, with careful attention to the multiple variables that, in addition to the dialysis itself, influence outcome. Achieving target solute concentrations in the patient during and between treatments requires complex mathematical models with multiple variables to account for differences among patients, including differences in size and solute generation rate. These factors add considerable complexity to the relatively simple laws of diffusion and flow discussed earlier, so that the solutions to patient problems are often approximations at best.
Types of Clearance
As noted in the discussion of dialysis and depicted in Eq. 2 , dialyzer clearance is the solute removal rate (flux) factored by the blood inflow concentration. During single-pass dialysis, the flux of urea is directly proportional to the inflow concentration, so that urea clearance tends to be constant despite the fall in blood concentration with time. The simplest type of clearance is the instantaneous dialyzer clearance, which can be measured by sampling blood on both sides of the dialyzer while recording Q b at any instant in time. Although the dialyzer urea clearance tends to remain constant, it may fall during treatment because of loss of surface area from clotting or because of changes in Q b or Q d . The effective clearance, or integrated dialyzer clearance, accounts for these changes by linking the measurement of clearance to the predialysis and postdialysis blood urea nitrogen (BUN). This clearance is essentially the answer to this question: What average urea clearance would be required to drive the BUN down to the measured postdialysis value from the measured predialysis value?
The mathematical solution requires a process known as urea modeling (see Quantifying Dialysis). It can be calculated using either a single-compartment or a two-compartment urea kinetic model, entering a predialysis and immediate postdialysis BUN in the former case and multiple intradialysis and postdialysis BUN in the latter case. In either case, the result is a dialyzer urea clearance that is not affected by urea disequilibrium. The integrated dialyzer clearance is often called the delivered clearance to distinguish it from the prescribed clearance.
During dialysis, solutes must diffuse from body tissues into the blood to reach the dialyzer. Even within the dialyzer as blood passes through it, solutes must diffuse from within red cells to the plasma before diffusion can take place across the dialyzer membrane into the dialysate. The striking difference between the ability of urea and creatinine to traverse red cell membranes shown in Fig. 22.3 explains in part why urea is preferred to creatinine as a marker for small-solute clearance. Such compartmentalization of body fluids adds complexity to the concept of clearance because different values may be chosen for the denominator of Eq. 3 . Even for urea, which diffuses easily across cell membranes from tissue to blood, some disequilibrium still develops among the various body compartments during dialysis, as reflected in the postdialysis urea rebound shown in Fig. 22.4 . As a result, the patient’s clearance, or whole body clearance, defined as the urea removal rate divided by the average urea concentration in total body water, is always less than the dialyzer clearance. Whole body clearance is a virtual clearance (not instantaneously measurable) that can be derived from single-compartment modeling (see Mathematical Models of Urea Kinetics) of the predialysis and equilibrated postdialysis BUN. Like dialyzer clearance, it is also a mean clearance integrated over time on dialysis, but it accounts for the presence of solute disequilibrium.


Adding further complexity to the concept of clearance are the frequency and duration of dialysis. Because residual native kidney clearance (K r ) exerts most of its effect between dialyses when dialyzer clearance is zero, it cannot be directly added to dialyzer clearance (see Quantifying Hemodialysis). Intermittent clearance, as obtained with hemodialysis, is inherently less efficient than the continuous clearance of native kidneys or continuous peritoneal dialysis. There are two explanations for this reduced dialysis efficiency.
First, although dialyzer clearance is not compromised by an intermittent schedule, total solute removal is reduced because blood solute concentrations decline logarithmically and not linearly during dialysis (see Fig. 22.4 ). Because solute levels do not change during continuous dialysis, this effect is absent and solute removal is maximal at all times. Therefore to reduce solute levels to a similar value, intermittent dialysis must be more intense when averaged over a week of treatment, as shown by the uppermost line in Fig. 22.5 . This explanation applies even in the absence of solute disequilibrium.

The second explanation applies to the more realistic situation in which solute concentration in the blood compartment is less than that in other compartments as solute disequilibrium develops during dialysis. Here again, dialyzer clearance is unaffected but solute access to the dialyzer is limited (see later further discussion in Solute Disequilibrium). For continuous replacement modalities and native kidney function, the blood solute concentration is stable and the effect of solute disequilibrium is minimal, so clearances are easy to calculate:
Native Kidney urea clearance = K r = ( U urea × V ) / ( P urea × t )
Peritoneal urea clearance = ( D urea × V ) / ( P urea × t )
It is apparent from the previous discussion that the clearances measured during intermittent forms of dialysis are not directly comparable to native kidney clearance or clearances measured in patients undergoing continuous dialysis. The previous observations also help to explain the significant difference between the minimum recommended weekly dose of dialysis (Kt/V) for hemodialysis (1.2 per dialysis × 3 dialyses per week = 3.6/week) and for peritoneal dialysis (1.7/week). To allow a direct comparison, the following formulas adjust for intermittence by calculating the continuous equivalent of intermittent clearance ( equivalent Kt/V or EKR). For intermittent therapy during a steady state of urea nitrogen balance,
E K R m e a n = removal rate mean concentration = generation rate mean concentration = G TAC
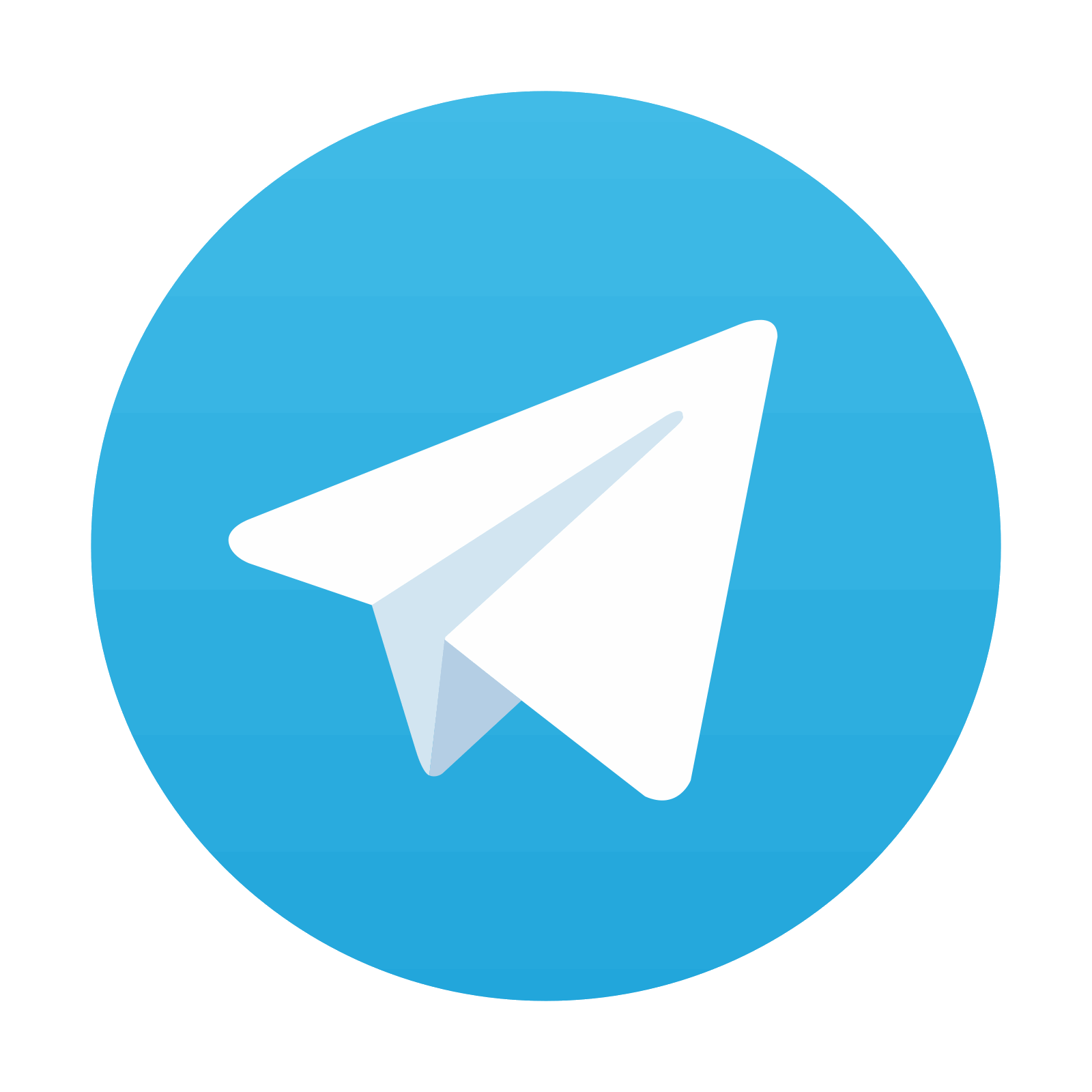
Stay updated, free articles. Join our Telegram channel
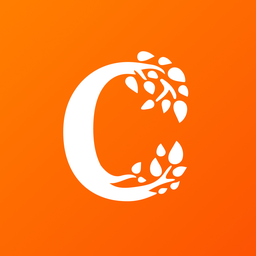
Full access? Get Clinical Tree
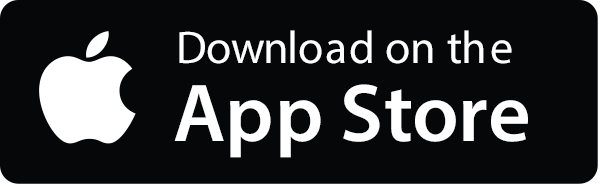
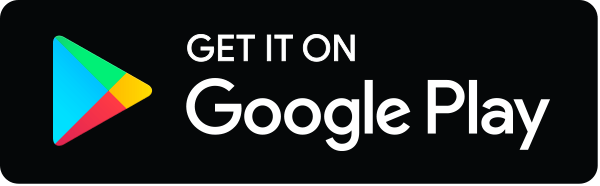