(a) Blood loss anemia
Intraoperative blood loss
Repeated blood draws—phlebotomy
Gastrointestinal
Menstrual blood losses
(b) Decreased RBC production
Decreased effective production
Iron deficiency—absolute or functional
Folate deficiency—medications
Pure red cell aplasia—parvo virus, medications
Bone marrow suppression—medications (Mycophenolate, Imuran), infections (viral)
Poor allograft function—erythropoietin deficiency
Ineffective erythropoiesis
Folate deficiency
Sideroblastic anemia—INH and other drugs
(c) Increased RBC destruction
Microangiopathic hemolytic anemia—calcineurin inhibitors, Sirolimus
G6PD deficiency—sulfa medications
Blood Loss Anemia
Blood loss is a common cause of anemia in kidney transplant patients. In the immediate post-transplant period, intraoperative blood loss and blood loss related to frequent phlebotomies are common causes of anemia. In the first few weeks after transplantation, patients frequently get multiple vials of blood drawn to monitor kidney function, screen for viral infections and also to adjust the doses of immunosuppression medications. In one study, average blood loss related to operative and phlebotomy losses in the first 12 weeks after transplantation was approximately 755–860 mL [17]. This can quickly result in profound anemia, especially if there is preexisting iron deficiency at the time of kidney transplantation or if allograft function is poor. Fortunately, in most patients, brisk production of erythropoietin from the new allograft negates the drop in hemoglobin, despite blood draws.
Later in the post-transplant period, causes of anemia due to blood loss change and include gastrointestinal bleeding and menstrual blood losses. Common causes of gastrointestinal bleeding include gastritis, peptic ulcer disease, diverticulosis, and arterio-venous malformations [18, 19]. While transplant medications, including steroids and mycophenolate, can cause gastritis and significant gastric erosions, infectious etiologies including cytomegalovirus can also play a role. Other rare causes of bleeding include post-transplant lymphoma in GI tract and Kaposi sarcoma.
In women, abnormal uterine bleeding is a common cause of anemia. When on dialysis, hypothalamic–pituitary–ovarian axis dysfunction can lead to menstrual irregularities, anovulation, and infertility [20, 21]. After kidney transplantation, ovarian axis dysfunction may improve resulting in regular menstruation cycles. Similarly, fertility can also be restored with normal kidney function. But in some transplant patients, despite normal allograft function, luteal insufficiency may persist and premature ovarian failure can occur. It is not uncommon to see menorrhagia worsen after kidney transplantation. Unless specifically asked, women often do not report excessive menstrual blood losses to the transplant physician. The slow decline in hemoglobin and mean corpuscular volume (MCV) levels should prompt the physician to inquire about menstrual losses. If confirmed, patients should be referred early to a gynecologist. It is also important to rule out other structural lesions, including endometrial carcinoma and uterine fibroids. In women with significant menstrual losses, parenteral iron may be frequently necessary to keep up with ongoing losses. When indicated, hysteroscopic endometrial ablation is a safe and effective procedure in transplant patients [22]. However, in some women, hysterectomy may be needed. Kidney transplant patients who undergo hysterectomy have higher incidence of perioperative complications, but surgery does not affect graft outcome [23].
Transplant Medications and Anemia
The majority of medications used in kidney transplant recipients to prevent acute rejections and opportunistic infections can cause PTA. While anemia related to myelosuppression is common, there are unique aspects that deserve special mention.
Calcineurin Inhibitors and Anemia
Calcineurin inhibitors (CNI) , tacrolimus and cyclosporine, are associated with microangiopathic hemolytic anemia (MHA) [24, 25]. CNIs exhibit their immunosuppressive properties by inhibiting the cytoplasmic dephosphorylation of NFAT (nuclear factor of activated T-lymphocytes), thereby preventing it from entering the cell nucleus and triggering downstream cytokine production. Similarly, CNI inhibitors also inhibit protein phosphatase 2B in vascular endothelium [26]. This phosphatase inhibition leads to excess secretion of unusually large von Willebrand factor (ULVWF) multimers from vascular endothelial cells, and the anchorage of these long strings of ULVWF to endothelium leads to platelet adhesion and aggregation. Under normal secretory conditions, circulating ADAMTS-13, a vWF cleaving metalloproteinase, will quickly cleave these long strings and avoid platelet thrombi. But CNI inhibitor-induced excess secretion of ULVWF strings appears to overwhelm the capacity of ADAMTS-13 activity, thereby leading to platelet thrombi and TTP. There are also data that CNI inhibitors may mediate Weibel-Palade body exocytosis. WPBs are endothelial granules that store coiled vWF multimers. All these factors play a role in CNI inhibitors causing MHA.
Sirolimus and Anemia
Depending on the anemia definition used, the prevalence of anemia associated with sirolimus (SRL) has been reported to be present in 16–56% of patients [27]. Multiple factors appear to play a role, including the dose of SRL, other transplant medicines used concomitantly, level of allograft function, and time when assessed post-transplant. Evidence for any direct link between SRL and anemia is best exemplified in CNI withdrawal studies. When patients with CNI toxicity or chronic fibrosis were switched from CNI to SRL-based regimen, a significant drop in hemoglobin was noted, despite improvement in allograft function [28]. Some studies have extended this observation to show improvement in hemoglobin after discontinuation of SRL.
Interestingly, the data in children are less clear. A study from the ESPN/ERA-EDTA registry failed to identify SRL as an independent risk factor for anemia in children. But it is important to note that only 2% of children were maintained on SRL-based regimen in the registry.
Sirolimus-induced anemia is associated with low mean corpuscular volume (MCV), microcytosis. While it is tempting to attribute microcytosis to absolute iron deficiency [29], or functional iron deficiency resulting from inflammation and reticuloendothelial blockade [28], observed microcytosis may be independent of iron homeostasis. In some patients, administration of parenteral IV iron improved microcytosis [30], but the role of iron’s contribution to microcytosis is not convincingly proven. Microcytosis may be iron-independent and be related to direct mTOR inhibition affecting protein translation, cell growth, and cell cycle progression [31, 32]. SRL may affect erythroid progenitor differentiation and erythropoietin receptor-mediated proliferation [32, 33]. It is unclear if SRL induces an erythropoietin-resistant state and if this resistance can be overcome with higher level of endogenous or exogenous erythropoietin.
In SRL-treated patients, anemia appears to be less commonly observed than the observation of a low MCV. Some studies suggest that anemia develops only when there is associated EPO or iron deficiency. When the mTOR pathway is inhibited in healthy rats with normal kidney function, microcytosis is induced, but not anemia [34]. But, in humans with allograft dysfunction, SRL often leads to microcytic anemia rather than isolated microcytosis.
There are also reports of microangiopathic hemolytic anemia associated with sirolimus and everolimus use. However, the exact mechanism of MHA associated with mTOR inhibitors is currently unknown.
Azathioprine and Anemia
With the advent of mycophenolate, there has been a significant decline in the use of AZA in kidney transplant patients. Thus the use of AZA is now limited to patients who are unable to tolerate mycophenolate or sirolimus, or when pregnancy is planned since mycophenolate and sirolimus have significant teratogenic potential and should be avoided. With limited indications, anemia resulting from AZA use is not commonly encountered in clinical practice. In contrast to SRL-associated microcytic anemia, azathioprine (AZA) use is associated with macrocytic anemia and is dose-related. While leukopenia is more common, anemia can still be seen in a significant number of patients.
AZA is first metabolized to 6-mercaptopurine (6-MP), which is then activated via the hypoxanthine-guanine phosphoribosyl transferase (HGPRT) pathway . As shown in Fig. 11.1, 6-MP is inactivated by two pathways; thiol methylation via the thiopurine S-methyltransferase (TPMT) pathway, and oxidation via the xanthine oxidase (XO) pathway . Inhibition of either pathway can lead to accumulation of its metabolite, 6-MP, and result in significant bone marrow toxicity. Co-administration of drugs that inhibit XO pathway (Allopurinol) can cause bone marrow toxicity. Similarly, patients who are born with low or absent TPMT activity are also at increased risk of severe bone marrow toxicity.


Fig. 11.1
Metabolism of azathioprine
When severe bone marrow suppression is noted after AZA use, genetic polymorphism of TPMT should be considered. In addition to genotyping tests, phenotyping can be done by measuring red cell TPMT activity. Approximately 10% of Caucasians and African Americans are heterozygous, conferring intermediate TPMT activity.
Mycophenolate and Anemia
Mycophenolate , an inhibitor of inosine-5′-monophosphate dehydrogenase enzyme, is associated with bone marrow suppression and cytopenia [35]. Anemia has been reported in almost a quarter of patients who take mycophenolate. Anemia and leukopenia are more common, when mycophenolate is used in combination with tacrolimus. This is probably related to higher level of mycophenolic acid resulting from drug interaction with tacrolimus. Inhibition of IMP-dehydrogenase in erythroid cells appears to be the cause of anemia [36]. Significant suppression of bone marrow EPO-receptors [37] and genetics [38] may also play a role. In addition to direct bone marrow suppression, mycophenolate is also associated with gastrointestinal bleeding, and resultant anemia from blood loss.
Angiotensin Blockade and Anemia
In kidney transplant patients with stable allograft function, renin–angiotensin system (RAS) blockade is often used for blood pressure control, heart failure management, and for reducing proteinuria. Since angiotensin II (AT II) plays an important role in the pathogenesis of CNI nephrotoxicity and chronic fibrosis, it is often tempting to use RAS blockade to prolong graft half-life. However, the use of Losartan had no significant effect on the composite end point of ESRD, death or doubling of serum creatinine value [39]. Even though these medications are well tolerated, anemia is often an under-recognized complication.
During hematopoiesis, hematopoietic stem cells undergo a complex series of proliferation and differentiation within the bone marrow. This is controlled by numerous cytokines and growth factors, including the renin–angiotensin system (RAS) [40, 41]. There is evidence of the existence of a functional RAS system in bone marrow. AT II interacts with bone marrow AT1 receptors leading to higher numbers of burst-forming unit-erythroid (BFU-E) colonies. This erythroid stimulation is blocked by the addition of an angiotensin receptor blocker, resulting in anemia. In addition to regulating ATII synthesis, angiotensin–converting enzyme (ACE) is primarily responsible for the degradation of a tetrapeptide, acetyl-N-Ser-Asp-Lys-Pro (AcSDKP), an endogenous physiologic inhibitor of hematopoiesis. Hence, inhibition of ACE enzyme will lead to lower generation of hematopoietic stimulant, ATII, and also parallel accumulation of hematopoietic inhibitor, AcSDKP. A better understanding of the role of ATII in hematopoiesis has led to physicians to use RAS blockade drugs for the treatment of post-transplant erythrocytosis [42–45].
Dapsone and Anemia
Dapsone (4,4′-diaminodiphenyl sulfone), a sulfone antibiotic that inhibits folate synthesis, is rarely used in post-transplant patients for prophylaxis against Pneumocystis jirovecii infection [46]. In patients with allergy to sulfamethoxazole-trimethoprim, most centers now use atovaquone or aerosolized pentamidine, instead of dapsone. Dapsone is also occasionally used as alternate therapy for transplant patients with severe toxoplasmosis.
Methemoglobinemia can be a life-threatening condition and has been reported with use of oral dapsone. Methemoglobin is an abnormal form of hemoglobin resulting from the oxidation of iron in the heme molecule from ferrous to ferric form. This change shifts the oxygen-hemoglobin dissociation curve to the left, thereby impairing the release of oxygen to the tissues.
Dapsone is metabolized via cytochrome P450 2C and 3A4 pathways to metabolites. Both the parent drug and metabolites cause oxidant stress to the red cell, and are responsible for the oxidation of heme iron to ferric form, thereby causing methemoglobinemia and resultant hemolytic anemia [47]. Symptoms of methemoglobinemia can range from cyanosis, headache, fatigue to serious events such as cardiac arrhythmias, seizures, and death. While direct measurement of methemoglobin concentration will clinch the diagnosis, oxygen saturation differential between pulse oximetry reading and arterial blood gas measurement should alert the transplant physician. In addition to discontinuation of dapsone, patients may require intravenous methylene blue, or in severe cases, dialysis.
Concurrent G6PD deficiency increases the risk of oxidative stress from glutathione depletion. Since the use of Dapsone is limited to a small number of transplant patients and since it is often co-administered with other medications that cause anemia, it is prudent to check for concurrent G6PD deficiency before starting Dapsone. Dapsone and nitrofurantoin are likely to be unsafe in patients with moderate to severe enzyme deficiency. Other drugs that can cause methemoglobinemia include metoclopramide and sulfonamides.
Isoniazid and Anemia
Isoniazid (INH) is often used in transplant patients to treat latent tuberculosis infection. INH causes sideroblastic anemia by interfering with pyridoxine (vitamin B6) metabolism. INH metabolite can inhibit pyridoxine phosphokinase, an enzyme that converts pyridoxine to active pyridoxal-5-phosphate. Active pyridoxal-5-phosphate plays a pivotal role in heme biosynthesis. During heme biosynthesis, the initial step of glycine and succinyl CoA interaction to form 5′-aminolevulinic acid in the erythroid cell is dependent on a mitochondrial enzyme that requires pyridoxal-5-phosphate as a cofactor. So, the absence of active pyridoxal-5-phophate in INH -treated patients will interfere with heme synthesis and result in ineffective hematopoiesis and anemia. Bone marrow aspirate showing the presence of nucleated erythroblasts with abnormal iron-laden mitochondria in the perinuclear area (ringed sideroblasts) is characteristic of this condition. To avoid this complication, INH is routinely administered with vitamin B6. Since alcohol also causes sideroblastic anemia and can worsen liver injury, patients taking INH should abstain from alcohol use.
Pure Red Cell Aplasia
Pure red cell aplasia (PRCA) can occur in transplant recipients from a wide variety of causes. PRCA is a syndrome characterized by anemia, usually severe, very low reticulocyte count and complete absence of erythroid precursors on bone marrow examination. In PRCA, WBC and platelet counts are normal. PRCA has been rarely reported with the use of mycophenolate and the exact mechanism is unknown. PRCA is sometimes reversible with dose reduction or withdrawal of the drug. Other medications that have been associated with PRCA include azathioprine, sulfonamides, INH, phenytoin, zidovudine, valproic acid, and clopidogrel.
In addition to medications, PRCA can also be associated with parvovirus B-19 infection. By attaching to the blood group P antigen receptor, parvovirus directly destroys proerythroblasts, resulting in PRCA. In this condition, bone marrow examination is characterized by the presence of giant proerythroblasts. The diagnosis is confirmed with the presence of parvovirus B-19 DNA in the serum, and also with anti-B19 IgM antibodies in serum. In some patients, anemia is refractory and may need frequent blood transfusions and multiple doses of intravenous immune globulin (IVIg) infusions. In addition to Parvo-viral infection, several other viral etiologies including viral hepatitis, cytomegalovirus, Epstein–Barr virus, and HIV infection have been reported to cause PRCA.
Diagnosis
An acute drop in hemoglobin concentration in the immediate post-transplant period is most likely related to a combination of intra-operative blood loss and dilutional component from over-zealous fluid replacement in the post-operative period, especially when there is delayed graft function. If the drop is significant and disproportionate, imaging study is warranted to rule out bleeding complication and hematoma formation.
Anemia noted in the outpatient setting is often related to persistent EPO or nutritional deficiencies, transplant drugs or infections. Anemia should warrant complete work up including checking red blood cell indices (mean corpuscular volume, mean corpuscular hemoglobin, mean corpuscular hemoglobin concentration), reticulocyte count, iron studies (serum iron, total iron binding capacity, serum ferritin), folate, and vitamin B12 concentrations. In patients with iron deficiency, stool occult blood should be checked and if positive, will need complete gastrointestinal work up including endoscopy. In women, menstrual blood losses should be ruled out as the cause of anemia. Since nutritional deficiencies are easily correctable, it is prudent to rule them out first. If there is no nutritional deficiency, peripheral blood smear is often helpful, along with search for evidence of hemolysis, including serum LDH, indirect bilirubin, and haptoglobin levels. Peripheral smear is often helpful to diagnose microangiopathic hemolysis (schistocytes).
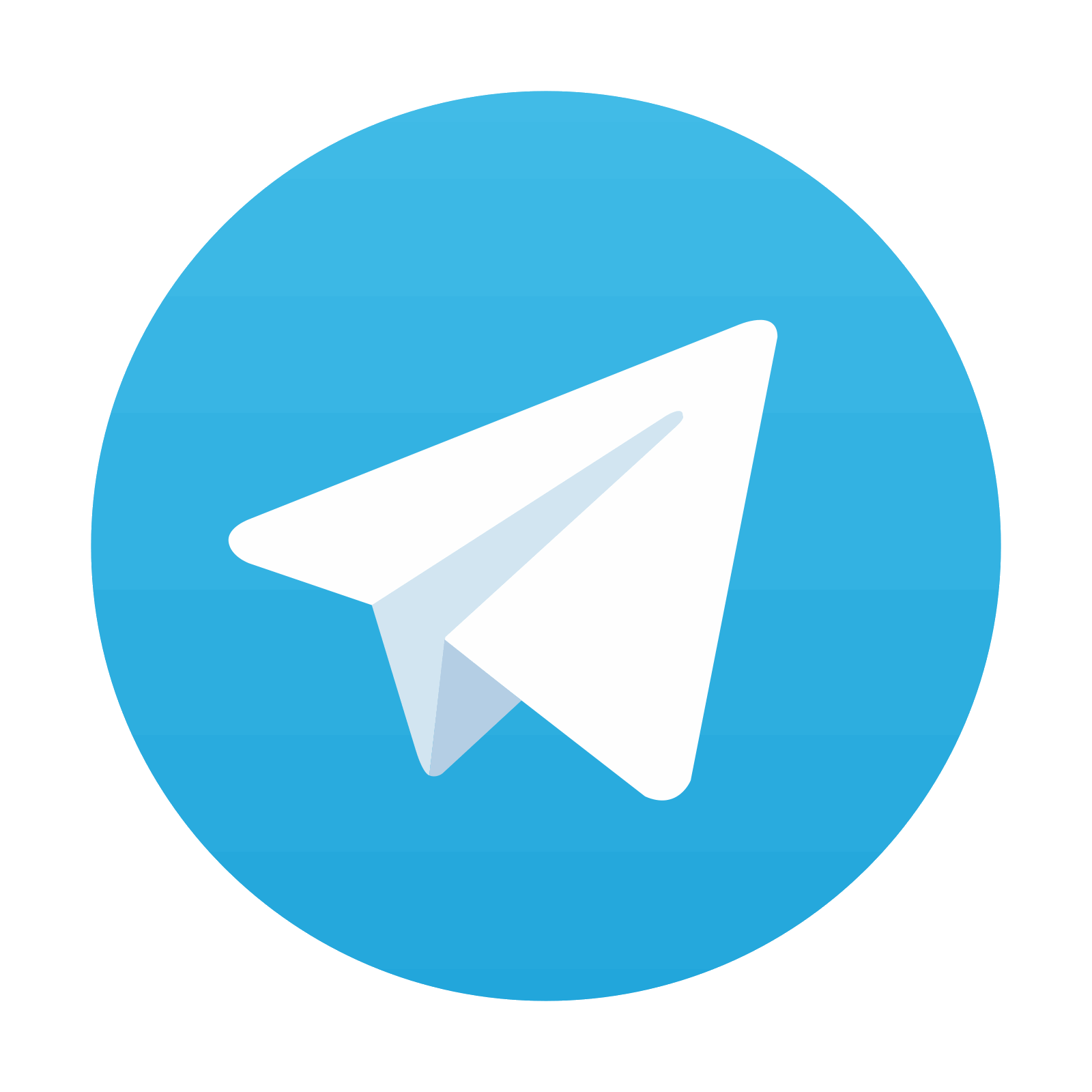
Stay updated, free articles. Join our Telegram channel
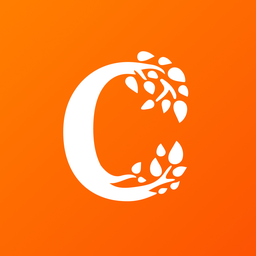
Full access? Get Clinical Tree
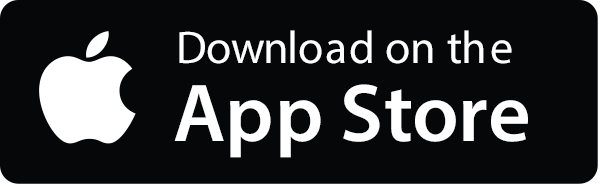
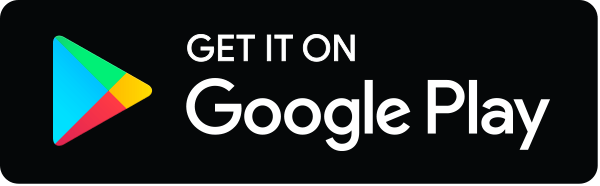